DOI:
10.1039/D1NA00508A
(Minireview)
Nanoscale Adv., 2021,
3, 6040-6047
Recent progress in cellulose-based electrospun nanofibers as multifunctional materials
Received
29th June 2021
, Accepted 6th September 2021
First published on 6th September 2021
Abstract
Cellulose, the most abundant natural polymer, has good biocompatibility, biodegradability, and non-toxicity, which make it and its derivatives promising candidates for the fabrication of multifunctional materials, while maintaining sustainability and environmental friendliness. The combination of electrospinning technology and cellulose (and its derivatives) provides a feasible approach to produce nanostructured porous materials with promising functionalities, flexibility, renewability and biodegradability. At the same time, it enables value-added applications of cellulose and its derivatives that are derived from nature or even biomass waste. This review summarizes and discusses the latest progress in cellulose-based electrospun nanofibers, including their construction methods and conditions, various available raw materials, and applications in multiple areas (water treatment, biomaterials, sensors, electro-conductive materials, active packaging, and so on), which are followed by the conclusion and prospects associated with future opportunities and challenges in this active research area.
1. Introduction
Electrospinning is a facile and efficient method for preparing fibers with nano-scaled diameters, which have a large specific surface area, good interconnectivity, and structural stability. Meanwhile owing to the random arrangement of nanofibers prepared by electrospinning, a large number of isotropic pores evenly distribute among fibers.1,2 These characteristics make electrospun nanofibers useful in a wide range of applications, including filtration & adsorption materials, “smart” materials, catalytic materials, and so on.3–7 However, the existing nanofibers fabricated by electrospinning are usually based on non-degradable polymers, which may cause environmental issues after disposal.
As the most abundant biodegradable polymer on the earth, cellulose possesses many fascinating properties, such as biocompatibility, environmental friendliness, and inexhaustible renewability.8 Taking advantage of the reactive, numerous, and regularly arranged hydroxyl groups along cellulose chains, a wide variety of cellulose derivatives have been developed by simple reactions between functional substituents and active hydroxyls.9 The derivatives possess interesting properties inherited from both cellulose and modified functional groups, including good solubility and processability, excellent flexibility, high mechanical strength, and so on.10,11 Hence, the combination of electrospinning technology and cellulose (and its derivatives) provides a feasible approach to produce nanostructured porous materials with desirable properties. At the same time, it enables value-added applications of cellulose and its derivatives that are derived from nature or even biomass waste.
Overviewing the research work published in last 3 years (2018–2021), various electrospun nanofibers have been successfully prepared from cellulose and cellulose derivatives in different solvent systems.12–17 These nanofibers demonstrated promising applications in the fields of filtration, tissue engineering and biomedical engineering.18–22 According to SciFinder, 17 literature reviews with the keywords “cellulose” and “electrospin” have been published during 2018–2021. It is certain that this is an active research area. However, all these reviews focus on either a single compound (e.g., cellulose acetate and nanocellulose)/single solvent system (e.g., ionic liquids) or a single application (e.g., supercapacitors, adsorbents, and wound healing materials). Therefore, this review will provide the researchers with a holistic view of recently reported cellulose-based electrospun nanofibers, including their raw materials (cellulose, cellulose derivatives, and nanocellulose), construction methods (electrospinning solvents and conditions), and applications (water treatment, biomaterials, sensors, electro-conductive materials, active packaging, etc.).
2. Cellulose-based electrospun nanofiber construction
Cellulose can be directly electrospun into nanofibers, but the process is limited by the poor solubility of cellulose in most commonly used solvents. Therefore, many research studies reported the electrospinning of cellulose derivatives. For different raw materials, optimized electrospinning conditions are essential for the successful fabrication of nanofibers. Typical examples of cellulose-based electrospun nanofibers and their production conditions published in the last three years are summarized in Table 1.
Table 1 Typical cellulose-based electrospun nanofibers and their production conditionsa
Raw materials |
Solvents |
Electrospinning conditions (concentrations, flow rates, voltages, and receiving distances) |
Ref. |
Note: PVA, poly(vinyl alcohol); CNCs, cellulose nanocrystals; PLA, poly(lactic acid); PVDF, polyvinylidene difluoride; PAN, poly(acrylonitrile); CNFs, cellulose nanofibrils; DMAc, dimethylacetamide; TFA, trifluoroacetic acid; DMF, N,N-dimethylformamide; THF, tetrahydrofuran.
|
Wood pulp cellulose |
DMAc/LiCl |
3 wt%; 1 mL h−1; 15 kV; 15 cm |
23
|
Bacterial cellulose |
TFA |
2–5 wt%; 0.5 mL h−1; 20 kV; 20 cm |
24
|
Cellulose acetate |
Acetic acid |
19 wt%; 0.75 mL h−1; 20 kV; 15 cm |
25
|
Cellulose acetate |
Acetone/DMF |
5 wt%; 35 μL min−1; 16 kV; 10 cm |
26
|
Cellulose acetate |
Acetone/DMAc |
16 wt%; 0.3 mL h−1; 11 kV; 15 cm |
13
|
Hydroxypropyl methylcellulose |
Ethanol |
1–6 wt%; 0.5–1 mL h−1; 10 kV; 15 cm |
27
|
Ethyl(hydroxyethyl) cellulose |
DMF |
5 wt%; 0.01–3.0 mL h−1; 7–14 kV; 10–20 cm |
28
|
Ethyl(hydroxyethyl) cellulose |
THF |
1 wt%; 0.2 mL h−1; 27 kV; 15 cm |
29
|
Ethyl cellulose |
Ethanol |
2 wt%; 0.3 mL h−1; 10–13 kV; 13 cm |
30
|
Aldehyde cellulose |
Water |
7.5 wt%; 0.01 mL min−1 10 kV; 20 cm |
31
|
PVA/CNCs |
Water |
7 wt%; 0.5 mL h−1; 22 kV; 10 cm |
32
|
PLA/CNCs |
CHCl3/DMF |
10 wt%; 16 kV; 15 cm |
33
|
PVDF/CNCs |
Acetone/DMF |
12 wt%; 0.02 mL min−1; 12 kV; 18 cm |
34
|
PAN/CNFs |
DMF |
1–10 wt%; 15 μL min; 20 kV; 20 cm |
35
|
2.1 Electrospinning of cellulose
The most effective way is to directly convert cellulose into nanofibers; however, raw materials have to be dissolved or melted for electrospinning. Although several cellulose solvent systems have been developed, such as ionic liquids,36N-methylmorpholine-N-oxide (NMMO),37 LiCl/DMAc,38 tetra(n-butyl)ammonium hydroxide/dimethyl sulfoxide (TBAH/DMSO),39 alkali/urea aqueous solution,40 and sulphuric acid solution,41 most of them are not suitable for electrospinning due to their high boiling point or high salt concentration. LiCl/DMAc has been proved as an effective solvent, and recent research focused on improving the current process and looking for new solvent systems. Shu et al.23 reported that wood pulp cellulose nanofibers were successfully prepared by the design of a new electrospinning receiving device and optimization of process parameters in a LiCl/DMAc dissolution system (Fig. 1). Compared to using a sink as the receiver, a novel drum-type receiving device was able to deal with high boiling-point solvents and obtain nanofibers with uniform diameters. The diameters of the resultant nanofibers were in the range of 200–550 nm; the porosity of the nanofiber mat was 76.5%; and there was no LiCl residue in the nanofibers. The breaking strength of the nanofibers could reach 148.2 cN. T. Jayani et al.24 developed a new approach to electrospin bacterial cellulose in a TFA/PVA solvent system. Without the addition of PVA as a co-solvent, only beaded particles were collected, and there was no nanofiber formation, irrespective of the solution concentration, voltage, and flow rate. Continuous nanofibers were formed due to the excellent spinnability of PVA and the hydrogen bonding interactions between PVA and bacterial cellulose, which exhibited an average breaking strength of 448 g F, breaking elongation of 10%, surface area of 4.248 m2 g−1, average pore volume of 0.005 cm3 g−1, and pore diameter of 1.72 nm.
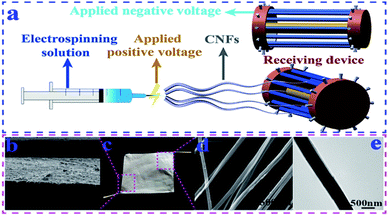 |
| Fig. 1 (a) Improved electrospinning receiving device. (b) SEM image of the cross section of the cellulose nanofiber mat. (c) Photo of a piece of the cellulose nanofiber mat. (d) SEM image of CNFs. (e) TEM image of CNFs (reprinted with permission,23 Elsevier publishing). | |
2.2 Electrospinning of cellulose derivatives
Compared to the poor solubility of cellulose, cellulose derivatives have greatly improved solubility in many organic and inorganic solvents due to changes in the structure of cellulose. Cellulose acetate (CA) is one of the most commonly used cellulose derivatives for the production of electrospun nanofibers because CA can achieve continuous and controllable electrospinning in several conventional solvents, such as acetic acid,25 acetone/DMAC solvent,13 and acetone/DMF/water,26 and the obtained CA nanofibers can be easily hydrolysed into cellulose nanofibers. The utilization of other cellulose derivatives has also been reported recently. For example, P. M. Silva et al.27 produced electrospun nanofibers from hydroxypropyl methylcellulose (HPMC) with different molecular weights. High molecular weight HPMC formed beaded fibers at a concentration of 1% (w/v) and smooth fibers when the concentrations were between 1.5 and 2.25% (w/v). However, HPMC with a low molecular weight could not form nanofibers but just particles. Electrospun nanofibers were also fabricated from ethyl cellulose and modified ethyl cellulose in DMF, THF, ethanol solutions for fluorescent materials, drug carriers, and food preservation, respectively, since ethyl cellulose has high versatility, biocompatibility, and excellent mechanical properties.28–30 At the same time, it was noticed that some water-soluble cellulose derivatives were employed for electrospinning in order to avoid the use of unfavourable organic solvents. Zhang et al.31 prepared water-soluble aldehyde cellulose by periodate oxidation, which was capable of forming nanofibers with a smooth surface and uniform size. The nanofiber diameters decreased from 833 nm to 303 nm with the increase of the oxidation degree from 34% to 91%. The prepared fibrous mats showed a moderate wet mechanical strength of around 1 MPa, and were able to absorb water equal to 30 times their own weight. These aldehyde cellulose nanofibers were particularly designed for biomedical fields, such as wound healing and tissue regeneration, and displayed a Murine L929 fibroblast cell viability of higher than 90%, suggesting good biocompatibility. However, their water-solubility limited the applications in high humidity or water environments.
2.3 Electrospinning of nanocellulose
Generally, the term “nanocellulose” encompasses rigid, rod-shaped cellulose nanocrystals (CNCs) and flexible, fiber-like cellulose nanofibrils (CNFs).42 Nanocellulose is distinguished by high mechanical strength, structurally configurable chemistry, a high aspect ratio, and biodegradability and has been applied as a sustainable “green” filler in many industrial applications.43 Due to its nanoscale dimensions, nanocellulose can be easily incorporated into electrospun nanofibers by dispersing in spinning solutions rather than dissolving, so as to maintain the nanostructure and special features. It is worth noting that nanocellulose can be modified to be dispersed in different organic and inorganic solutions. Recently, nanocellulose has been combined with various polymers (i.e., PLA,33 PVDF,34 and PAN35) to prepare composite nanofibers. For example, Zhang et al.32 fabricated electrospun PVA/CNC nanofibers for the filtration of particulate matter. The addition of nanocellulose might increase the diameter of nanofibers by interfering with the interactions of the original matrix44 or reduce the nanofiber diameter by increasing the charge density of electrospinning solution.32
3. Cellulose-based electrospun nanofiber applications
3.1 Water treatment
With the rapid development of urbanization and industrialization, wastewater discharge from various sources (agricultural wastes, biomedical industry, food industry, petroleum industry, manufacturing industry, etc.) poses a significant threat to the sustainability of the ecosystem and human health. Cellulose and its derivatives are widely involved in the treatment of contaminated water in the form of electrospun nanofibers, not only due to their intrinsic nanostructures but also owing to their active functional groups and subsequently good surface chemistry. For example, Phan et al.45 reported electrospun chitosan/cellulose nanofibers for the effective adsorption of heavy metal ions, where arsenic(V), lead(II), and copper(II) were chosen as representatives. It was illustrated that the hydrogen bonding in the structure of cellulose contributed to the high water stability of nanofibers, hindering the swelling behavior of chitosan in water to enable high adsorption capacity.
CA can be easily dissolved in conventional solvents, making it attractive as a carrier for loading other polymers and functional fillers with desired properties. Therefore, CA was of great significance in the field of water treatment in the last three years. For example, to tackle the aggregation issue of hydroxyapatite nanoparticles (a mineral fixator), Hamad et al.46 impregnated them into the CA matrix through the electrospinning technique. Hydroxyapatite particles with concentrations of up to 3 wt/v% could be well dispersed in the spinning solution, enabling the improvement of the mechanical strength and adsorption performance of nanofibers. The strong hydrogen bonding interactions between the nanoparticles and CA matrix also contributed to the composite integrity and stability during the adsorption process. Other modifications of electrospun CA nanofibers, such as the addition of thiol groups,47 citric acid,48 and polyvinylamine,13 have also been applied for the removal of Cu(II), Cr(VI), and organoarsenic contaminants, respectively. Photocatalysts such as ZnO49 and metal–organic frameworks50 are another group of functional fillers that are usually incorporated into electrospun nanofibers for degrading organic contaminants like effluent dye molecules. Besides the most widely used CA, cellulose acetate butyrate was also employed as a low-cost and accessible bearer for the immobilization of photocatalysts, which could provide a smooth and uniform membrane with an average fiber diameter of 1 μm for growing ZnO nanocrystals.49
The release of petroleum hydrocarbons from oil spills has serious environmental impacts on marine ecosystems. Cellulose has been electrospun into nanofibers for the application of oil/water separation, delivering extraordinary separation efficiencies of up to 99.5% for the emulsion of vacuum pump oil and water.51 A water film was generated immediately on the surface of the electrospun cellulose membrane owing to the existence of hydroxyl groups, while the oil droplets were extruded by the membrane due to their relatively larger diameters compared to the porous fiber network (Fig. 2). Electrospun cellulose nanofibers are usually obtained by deacetylating CA fibers.52,53 The incorporation of CNCs into a PVDF nanofibrous membrane also contributed to the oil/water separation by forming convex and concave structures on the nanofiber surface.34 The 4 wt% CNC/PVDF membrane showed the highest flux of water-in-toluene emulsion (5842 L
m−2 h−1) and separation efficiency (97%), which were due to the larger water contact angle along with the increased roughness, higher porosity, and larger average pore sizes. However, the water contact angle started to drop when the amount of CNCs increased to 6 wt%, owing to the presence of a high number of hydrophilic hydroxyl groups.
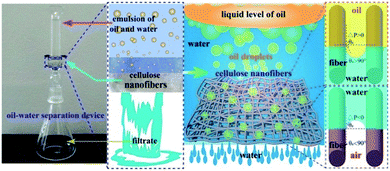 |
| Fig. 2 Illustration of the oil–water separation process and mechanisms by using electrospun cellulose nanofibers (reprinted with permission,51 Elsevier publishing). | |
3.2 Biomaterials
Electrospun nanofibers from cellulose and their derivatives have been widely used in the fields of biomedical materials and tissue engineering. For example, the requirements for wound healing materials such as biocompatibility, oxygen permeability, water absorption, and non-toxicity can be fulfilled by electrospun cellulose-based fabrics. Electrospun CA/collagen nanofibers were incorporated with latex (L) from C. Procera (medicinal plant) for wound healing, and it was found that CA played an important role in restricting the swelling behaviour and strengthening the fabrics.54 Similar electrospun CA nanofibers loaded with gelatin and Zataria multiflora (antibacterial plant) could absorb accumulated wound exudates and exhibited good wound healing capacity and antibacterial properties.55 A considerably smaller wound area on the burned upper back of rats was observed compared to the commercial gauze treatment after 22 days (Fig. 3). Moreover, electrospun ethyl cellulose56 and cellulose diacetate57 nanofibers with antibacterial properties were prepared by doping with silver sulfadiazine and protoporphyrin, respectively. The former showed good inhibition activities against Bacillus (9.71 ± 1.15 mm) and E. coli (12.46 ± 1.31 mm),56 while the protoporphyrin IX-embedded cellulose diacetate membranes exhibited 99.8% reduction in Gram-positive S. aureus after illumination.57
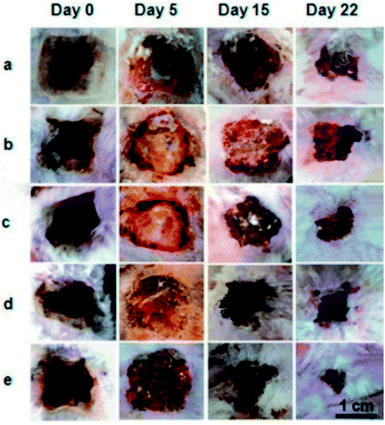 |
| Fig. 3 Representative images of the burn wound healing process treated with (a) commercial gauze, (b) drug-free nanofiber CA/Gel = 100 : 0, (c) drug-free nanofiber CA/Gel = 50 : 50, (d) drug-loaded nanofiber CA/Gel = 100 : 0, and (e) drug-loaded nanofiber CA/Gel = 50 : 50 (reprinted with permission,55 Elsevier publishing). | |
Bioactive compounds have also been loaded into electrospun cellulose-based nanofibers to increase their stability in harsh environments and controlled release at the destinations where they are typically absorbed. Recently, flufenamic acid (an anti-inflammatory drug) was encapsulated in electrospun carboxymethyl cellulose/PVA nanofibers for prolonged drug release.58 Covalent bonds between the amine groups of the drug and the carboxyl groups of carboxymethyl cellulose were established to extend the biological half-life of the drug. Electrospun CNC/polycaprolactone nanofibers for the release of tetracycline were reported by Hivechi et al.59 The presence of CNCs resulted in improved mechanical strength, degradability, and controlled release. The nanofibers were broken in the second week, and the number of broken fibers increased when the CNC content was up to 1 wt%.
3.3 Sensors
Electrospun nanofibers have a large surface area to volume ratio and excellent pore-interconnectivity, which are particularly important for initiating the signalling pathways for targeted compound detection in a short period of time. Moreover, compared to free nanoparticles, electrospun nonwoven fabrics have fewer safety issues owing to the immobilization of responsive compounds on the fibers, and better handling properties enabling easy application in various devices. Ethyl(hydroxyethyl)cellulose (EHEC) was functionalized by anchoring 4-(2-(pyridine-4-yl)vinyl)phenol (PBM) and 4-[4-(dimethylamino)styryl]pyridine, and then mixed with poly(methyl methacrylate) (PMMA) to prepare electrospun nanofibers for the detection of CN− in water.60 EHEC endowed the entire matrix with high hydrophilicity even at a low concentration (PMMA/EHEC 20
:
1 wt/wt), so as to have a high water retention capacity for application in fluorogenic optical devices and achieve the detection and quantification limits of 2.15 × 10−5 and 7.17 × 10−5 mol L−1, respectively.
More research work was based on electrospun CA matrices. For instance, core–shell ferrous nanoparticles functionalized with Rhodamine B were embedded into electrospun CA nanofibers for gas ammonia sensing.61 It was revealed that the encapsulation of γ-Fe2O3/SiO2/Rhodamine B nanoparticles impeded the release of fluorescent molecules to a significant extent and ensured the stability of sensing materials. Moreover, the good spinnability of CA enabled the loading of a high amount of sensing elements, and the large surface-to-volume ratio of CA nanofibers contributed to a high sensitivity in the response concentration range of 200 ppm to 12
750 ppm and a quick response time (30 s). Jia et al.62 exploited electrospun CA-based ratiometric fluorescent materials for monitoring the freshness of seafood through ammonia sensing (Fig. 4). The fluorescent unit, protoporphyrin IX, was covalently bonded to CA, which secured food safety by preventing migration. An instant fluorescent color-response (<1 s) of the prepared nanofibrous membrane to a drop of ammonia (concentration as low as 5.0 ppm) was demonstrated. Similar approaches were applied to synthesize CA-based nanofiber sensors for detecting mercury(II) and lead(II) ions, hydrogen chloride vapors, and gas ammonia.63,64 All of them exhibited fast detection of targeted substances.
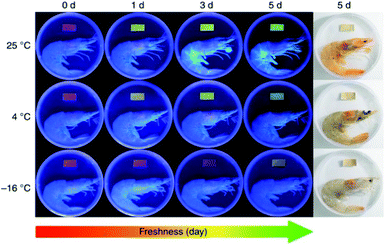 |
| Fig. 4 Electrospun cellulose acetate-based ratiometric fluorescent material with red initial fluorescence for monitoring the freshness of shrimps stored under different conditions (reprinted with permission,62 Springer Nature publishing). | |
3.4 Electro-conductive materials
A flexible support material with a three-dimensional porous structure is essential for developing conducting electrodes. Wu et al.65 reported electrospun polyindole/carbon nanotube/bacterial cellulose electrodes for energy storage devices. Its outstanding specific capacitance was ascribed to the three-dimensional hierarchical network formed by bacterial cellulose and polyindole for effective charge transfer. An electrospun polyacrylonitrile/cellulose nanofibril electrode carbon material has been developed35 and displayed improved mechanical strength and electrical conductivity compared to a neat polyacrylonitrile membrane. Cellulose nanofibrils also accelerated the stabilization process by reducing the required energy for nitrile-based cleavage in the carbonization reaction.
An interesting and novel study was reported by Lyu et al.,66 who utilized the porous structure of electrospun CA fabrics to construct moisture-induced electricity generators. The hydroxyl and carbonyl groups of CA dissociated and formed negatively charged surfaces when the nanofibers were exposed to moisture. Due to the accumulation of moisture on one side of the fabrics, an imbalanced distribution of protons occurred, and streaming potential was generated consequently (Fig. 5c). The potential was primarily impacted by the porosity and average pore size of electrospun nanofabrics that could be tuned by varying the annealing time. The generation of electricity in response to the change of the moisture content is shown in Fig. 5a and b. Water molecules moved along the 3D nanochannels with charged surfaces, generating a voltage output of up to 0.3 V and a short-circuit current density of 80 nA cm−2.
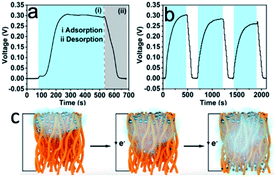 |
| Fig. 5 (a) Electricity generation as a function of moisture change based on the CA nanofiber with an annealing time of 5 min. The two shaded areas correspond to the (i) adsorption (blue zone) and (ii) desorption (grey zone) of moisture. (b) Electricity generation based on the CA nanofiber with an annealing time of 5 min in response to the change of RH (ΔRH = 85%). (c) Illustration of electrospun cellulose acetate nanofibers exposed to the moist flow (reprinted with permission,66 ACS publishing). | |
3.5 Active packaging
Cellulose and many of its derivatives, such as CMC, CA, ethyl cellulose, etc., have been regarded as safe food substances according to the U.S. Department of Agriculture and considered as food additives by the FDA regulations. Therefore, they are promising raw materials for the preparation of advanced food packaging. Zaitoon et al.67 fabricated electrospun ethyl cellulose/poly(ethylene oxide) hybrid nanofibers encapsulated with ethyl formate for the preservation of strawberries (Fig. 6). The efficacy was confirmed visually, where the strawberries treated with 2 mg ethyl formate released from electrospun nanofibers were not spoiled for up to 10 days, and the untreated strawberries showed microbial growth on day 8. Nevertheless, the stability of the prepared nanofibers was not good at 100% relative humidity, which might limit its applications under high moisture conditions. Niu et al.68 incorporated cinnamaldehyde essential oil (CEO) into electrospun zein/ethyl cellulose nanofibers for improved water resistance of food. Hydrogen bonds formed between the hydroxyl groups of ethyl cellulose and the amino groups of zein thus reduced the presence of free hydrophilic groups and improved the water-resistance remarkably, resulting in the controlled release of CEO during the storage period of fresh produce for achieving a prolonged shelf-life. At the same time, Yi et al.69 constructed electrospun anthraquinone-2-carboxylic acid grafted silk fibroin (G-SF)/CA nanofibers with antibacterial activity against E. coli, where CA contributed to the handling properties and the reinforcement of tensile strength from 34.52 cN mm−2 to 88.92 cN mm−2.
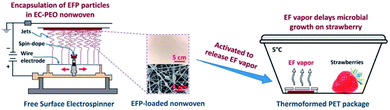 |
| Fig. 6 Ethyl formate-encapsulated electrospun ethyl cellulose/poly(ethylene oxide) nanofibers for strawberry preservation (reprinted with permission,67 Elsevier publishing). | |
3.6 Other applications
Based on the unique structure and properties of electrospun cellulose-based nanofibers, some other applications have been proposed in recent years. An interesting study was reported by Knapczyk-Korczak et al., who fabricated electrospun CA/polystyrene nanofibers for the collection of water from fog (Fig. 7).70 Due to the hydrophobicity of the CA/polystyrene matrix, water vapor was repelled from the fiber surface. The existence of CA reduced the roughness and contact angle of nanofibers, allowing an easier and free drainage pathway. Also, CA was proved to strengthen the entire matrix, which is a prominent property of water collectors.
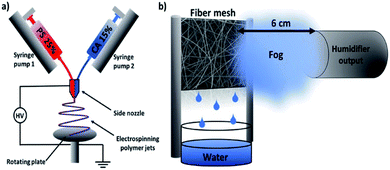 |
| Fig. 7 Schematic diagrams of electrospun cellulose acetate/polystyrene nanofibers for water harvesting (reprinted with permission,70 ACS publishing). | |
Electrospun cellulose nanofibers have played a role in energy conservation, such as dye-sensitized solar cells71 and solar thermal applications.72 Electrospun CA and deacetylated CA nanofibers were used as electrolyte membranes in dye-sensitized solar cells, which showed higher open circuit voltages (780 ± 10 mV and 780 ± 10 mV) compared to the reference cell (771 ± 9 mV).71 It was attributed to the more uniform distribution of the electrolyte within the electrospun membranes in contact with the electrode interface. At the same time, electrospun CA nanofibers loaded with thermal conductive cadmium selenide nanoparticles displayed high solar-thermal conversion potential and efficiency, which reached 40 °C under 1 sun (100 mW cm−2) illumination.72
Besides water treatment, electrospun nanofibers were also applied for air purification.73 With the aim of toluene (volatile organic compound) adsorption, CNCs were filled in electrospun polyamide 6 nanofibers to improve adsorption efficiency and mechanical strength, owing to their high hydrophilicity, large active surface area, and crystalline structure.74 In the meantime, electrospun PAN-based activated carbon and PVA nanofibers incorporated with CNCs were used for the adsorption of cyclohexane75 and filtration of particular matter.32,76 It was worth noting that various other functional fillers, such as silica nanoparticles,77 silica zeolites,78 iron oxide nanoparticles,79 and Ti3C2Tx (metal carbide),80 were doped in cellulose-based nanofibers for desalination, adsorption of ammonia vapor, tunable saturation magnetization, and electromagnetic interference shielding, respectively.
4. Conclusion and future prospects
Recent progress in cellulose-based electrospun nanofiber production and applications is summarized. In one aspect, research work focused on exploring new cellulose solvents and cellulose derivatives that are suitable for electrospinning and improving electrospinning apparatus for better fiber quality; in another, various applications in water treatment, biomaterials, sensors, electro-conductive materials, active packaging, and so on have been proposed to make full use of these renewable, biodegradable and nontoxic nanofibrous networks with a large surface area and interconnected porous structure. Different forms of cellulose (wood pulp, nanocellulose, etc.) and derivatives (CA, hydroxypropyl methylcellulose, carboxymethyl cellulose, azido-cellulose, aldehyde cellulose, ethyl cellulose, etc.) have been employed as raw materials for electrospinning. However, the optimized electrospinning conditions of all these materials have relatively low polymer concentrations in spinning solutions and low flow rates during spinning, resulting in low productivity. Therefore, future research on new solvents and cellulose derivatives is still required to address these issues and improve productivity. It is worth noting that the electrospinning technology improved largely in the past 15 years; for example, advanced electrospinning apparatuses allow the production of nanofabrics with multiple structures (aligned, multi-layered, composite, etc.). However, current research work mainly focuses on the formulations and electrospinning conditions of nanofibers, and not many novel structures have been reported. Hence, the rational design of electrospun nanofibers with better performance is expected in future research, which should be based on the understanding of the structure–property relationship and linked tightly with the targeted application.
Author contributions
Yirong Zhang: writing-original draft preparation, Cunzhi Zhang: writing-original draft preparation, and Yixiang Wang: supervision, writing-reviewing and editing.
Conflicts of interest
There are no conflicts to declare.
Acknowledgements
We would like to acknowledge financial support from the Fonds de Recherche du Quebec-Nature et Technologies (266796), Natural Sciences and Engineering Research Council of Canada (250374), Natural Sciences and Engineering Research Council of Canada Discovery Launch Supplement (250531), and McGill University Academic Startup Grant (130209). C. Z. would like to thank the China Scholarship Council (CSC No. 202006150146) for financial support.
References
- J. Xue, T. Wu, Y. Dai and Y. Xia, Chem. Rev., 2019, 119, 5298–5415 CrossRef CAS PubMed.
- Y. Zhang, F. Wang and Y. Wang, Mater. Today Commun., 2021, 27, 102272 CrossRef CAS.
- S. Lee, S. Franklin, F. A. Hassani, T. Yokota, M. O. G. Nayeem, Y. Wang, R. Leib, G. Cheng, D. W. Franklin and T. Someya, Science, 2020, 370, 966–970 CrossRef CAS PubMed.
- X. Sun, L. Bai, J. Li, L. Huang, H. Sun and X. Gao, Carbon, 2021, 182, 11–22 CrossRef CAS.
- J. Yan, Y. Wang, Y. Zhang, S. Xia, J. Yu and B. Ding, Adv. Mater., 2021, 33, e2007525 CrossRef PubMed.
- K. S. Han, S. Lee, M. Kim, P. Park, M. H. Lee and J. Nah, Adv. Funct. Mater., 2019, 29, 1903633 CrossRef.
- C. Chen, W. Zhang, H. Zhu, B.-G. Li, Y. Lu and S. Zhu, Nano Res., 2020, 14, 1465–1470 CrossRef.
- Q. Xia, C. Chen, Y. Yao, J. Li, S. He, Y. Zhou, T. Li, X. Pan, Y. Yao and L. Hu, Nat. Sustainability, 2021, 4, 627–635 CrossRef.
- M. Beaumont, P. Jusner, N. Gierlinger, A. W. T. King, A. Potthast, O. J. Rojas and T. Rosenau, Nat. Commun., 2021, 12, 2513 CrossRef CAS PubMed.
- M. C. Li, Q. Wu, R. J. Moon, M. A. Hubbe and M. J. Bortner, Adv. Mater., 2021, 33, e2006052 CrossRef PubMed.
- R. M. O'Dea, J. A. Willie and T. H. Epps, ACS Macro Lett., 2020, 9, 476–493 CrossRef.
- S. R. Djafari Petroudy, S. Arjmand Kahagh and E. Vatankhah, Carbohydr. Polym., 2021, 251, 117087 CrossRef CAS PubMed.
- K. Liu, Z. Huang, J. Dai, Y. Jiang, G. Yang, Y. Liu, C. Lin, Y. Lv and M. Liu, Chem. Eng. J., 2020, 382, 122775 CrossRef CAS.
- Q. Cao, M. Zhu, J. Chen, Y. Song, Y. Li and J. Zhou, ACS Appl. Mater. Interfaces, 2020, 12, 1210–1221 CrossRef CAS PubMed.
- T. Pirzada, Z. Ashrafi, W. Xie and S. A. Khan, Adv. Funct. Mater., 2019, 30, 1907359 CrossRef.
- S. Hell, K. Ohkawa, H. Amer, A. Potthast and T. Rosenau, Nanomaterials, 2020, 10, 671 CrossRef CAS PubMed.
- X. Miao, J. Lin and F. Bian, J. Bioresour. Bioprod., 2020, 5, 26–36 CrossRef.
- N. Tang, Y. Li, J. Ge, Y. Si, J. Yu, X. Yin and B. Ding, ACS Appl. Mater. Interfaces, 2020, 12, 31852–31862 CrossRef CAS PubMed.
- S. Zheng, M. Du, W. Miao, D. Wang, Z. Zhu, Y. Tian and L. Jiang, Adv. Funct. Mater., 2018, 28, 1800832 CrossRef.
- M. A. Teixeira, M. C. Paiva, M. T. P. Amorim and A. H. P. Felgueiras, Nanomaterials, 2020, 10, 557 CrossRef CAS PubMed.
- T. Lu, J. Cui, Q. Qu, Y. Wang, J. Zhang, R. Xiong, W. Ma and C. Huang, ACS Appl. Mater. Interfaces, 2021, 13, 23293–23313 CrossRef CAS PubMed.
- M. Zhang, W. Ma, J. Cui, S. Wu, J. Han, Y. Zou and C. Huang, J. Hazard. Mater., 2020, 383, 121152 CrossRef CAS PubMed.
- D. Shu, P. Xi, B. Cheng, Y. Wang, L. Yang, X. Wang and X. Yan, Int. J. Biol. Macromol., 2020, 162, 1536–1545 CrossRef CAS PubMed.
- T. Jayani, B. Sanjeev, S. Marimuthu and S. Uthandi, Carbohydr. Polym., 2020, 250, 116965 CrossRef CAS PubMed.
- N. Olaru, N. Anghel, P. Pascariu and G. Ailiesei, J. Appl. Polym. Sci., 2019, 136, 47772 CrossRef.
- W. Chen, H. Ma and B. Xing, Int. J. Biol. Macromol., 2020, 158, 1342–1351 CrossRef CAS PubMed.
- P. M. Silva, C. Prieto, J. M. Lagarón, L. M. Pastrana, M. A. Coimbra, A. A. Vicente and M. A. Cerqueira, Food Hydrocolloids, 2021, 118, 106761 CrossRef CAS.
- J. P. Dreyer, R. I. Stock, L. G. Nandi, I. C. Bellettini and V. G. Machado, Carbohydr. Polym., 2020, 236, 115991 CrossRef CAS PubMed.
- A. Wali, Y. Zhang, P. Sengupta, Y. Higaki, A. Takahara and M. V. Badiger, Carbohydr. Polym., 2018, 181, 175–182 CrossRef CAS PubMed.
- B. Niu, L. Zhan, P. Shao, N. Xiang, P. Sun, H. Chen and H. Gao, Int. J. Biol. Macromol., 2020, 142, 592–599 CrossRef CAS PubMed.
- H. Zhang, Y. Liu, S. Cui, Y. Zhou, J. Hu, J. Ma and Y. Liu, Cellulose, 2020, 27, 8695–8708 CrossRef CAS.
- Q. Zhang, Q. Li, T. M. Young, D. P. Harper and S. Wang, ACS Sustainable Chem. Eng., 2019, 7, 8706–8714 CrossRef CAS.
- D. K. Patel, S. D. Dutta, J. Hexiu, K. Ganguly and K. T. Lim, Int. J. Biol. Macromol., 2020, 162, 1429–1441 CrossRef CAS PubMed.
- X. Wang, W. Cheng, D. Wang, X. Ni and G. Han, J. Membr. Sci., 2019, 575, 71–79 CrossRef CAS.
- W. Xu, B. Xin and X. Yang, Cellulose, 2020, 27, 3789–3804 CrossRef CAS.
- B. Araldi da Silva, R. de Sousa Cunha, A. Valério, A. De Noni Junior, D. Hotza and S. Y. Gómez González, Eur. Polym. J., 2021, 147, 110283 CrossRef CAS.
- R. Protz, A. Lehmann, J. Ganster and H. P. Fink, Carbohydr. Polym., 2021, 251, 117027 CrossRef CAS PubMed.
- J. Garemark, X. Yang, X. Sheng, O. Cheung, L. Sun, L. A. Berglund and Y. Li, ACS Nano, 2020, 14, 7111–7120 CrossRef CAS PubMed.
- X. Chen, X. Chen, X.-M. Cai, S. Huang and F. Wang, ACS Sustainable Chem. Eng., 2018, 6, 2898–2904 CrossRef CAS.
- H. Tu, M. Zhu, B. Duan and L. Zhang, Adv. Mater., 2021, 33, 2000682 CrossRef CAS PubMed.
- C. Zhou and Y. Wang, J. Appl. Polym. Sci., 2021, 138, 51255 CrossRef CAS.
- N. Peng, D. Huang, C. Gong, Y. Wang, J. Zhou and C. Chang, ACS Nano, 2020, 14, 16169–16179 CrossRef CAS PubMed.
- M. E. Pasaoglu and I. Koyuncu, Chemosphere, 2021, 269, 128710 CrossRef CAS PubMed.
- Y. Wang and L. Chen, ACS Appl. Mater. Interfaces, 2014, 6, 1709–1718 CrossRef CAS PubMed.
- D.-N. Phan, H. Lee, B. Huang, Y. Mukai and I.-S. Kim, Cellulose, 2019, 26, 1781–1793 CrossRef CAS.
- A. A. Hamad, M. S. Hassouna, T. I. Shalaby, M. F. Elkady, M. A. Abd Elkawi and H. A. Hamad, Int. J. Biol. Macromol., 2020, 151, 1299–1313 CrossRef CAS PubMed.
- H. Y. Choi, J. H. Bae, Y. Hasegawa, S. An, I. S. Kim, H. Lee and M. Kim, Carbohydr. Polym., 2020, 234, 115881 CrossRef CAS PubMed.
- D. Zhang, W. Xu, J. Cai, S.-Y. Cheng and W.-P. Ding, Int. J. Biol. Macromol., 2020, 149, 459–466 CrossRef CAS PubMed.
- P. Pascariu, N. Olaru, A. Rotaru and A. Airinei, Nanomaterials, 2020, 10, 1873 CrossRef CAS PubMed.
- W. Lu, C. Duan, Y. Zhang, K. Gao, L. Dai, M. Shen, W. Wang, J. Wang and Y. Ni, Carbohydr. Polym., 2021, 258, 117676 CrossRef CAS PubMed.
- D. Shu, P. Xi, B. Cheng, Y. Wang, L. Yang, X. Wang and X. Yan, Int. J. Biol. Macromol., 2020, 162, 1536–1545 CrossRef CAS PubMed.
- H. P. Karki, L. Kafle, D. P. Ojha, J. H. Song and H. J. Kim, Sep. Purif. Technol., 2019, 210, 913–919 CrossRef CAS.
- W. Wang, J. Lin, J. Cheng, Z. Cui, J. Si, Q. Wang, X. Peng and L.-S. Turng, J. Hazard. Mater., 2020, 385, 121582 CrossRef CAS PubMed.
- G. Ramanathan, L. S. Seleenmary Sobhanadhas, G. F. Sekar Jeyakumar, V. Devi, U. T. Sivagnanam and P. Fardim, Biomacromolecules, 2020, 21, 2512–2524 CrossRef CAS PubMed.
- H. Farahani, A. Barati, M. Arjomandzadegan and E. Vatankhah, Int. J. Biol. Macromol., 2020, 162, 762–773 CrossRef CAS PubMed.
- S. Ahmadian, M. Ghorbani and F. Mahmoodzadeh, Int. J. Biol. Macromol., 2020, 162, 1555–1565 CrossRef CAS PubMed.
- T. Wang, H. Ke, S. Chen, J. Wang, W. Yang, X. Cao, J. Liu, Q. Wei, R. A. Ghiladi and Q. Wang, Mater. Sci. Eng., C, 2021, 118, 111502 CrossRef CAS PubMed.
- A. Allafchian, H. Hosseini and S. M. Ghoreishi, Int. J. Biol. Macromol., 2020, 163, 1780–1786 CrossRef CAS PubMed.
- A. Hivechi, S. H. Bahrami and R. A. Siegel, Mater. Sci. Eng., C, 2019, 94, 929–937 CrossRef CAS PubMed.
- J. P. Dreyer, R. I. Stock, L. G. Nandi, I. C. Bellettini and V. G. Machado, Carbohydr. Polym., 2020, 236, 115991 CrossRef CAS PubMed.
- A. Petropoulou, S. Kralj, X. Karagiorgis, I. Savva, E. Loizides, M. Panagi, T. Krasia-Christoforou and C. Riziotis, Sci. Rep., 2020, 10, 367 CrossRef CAS PubMed.
- R. Jia, W. Tian, H. Bai, J. Zhang, S. Wang and J. Zhang, Nat. Commun., 2019, 10, 795 CrossRef CAS PubMed.
- S. Ahmadian-Fard-Fini, D. Ghanbari, O. Amiri and M. Salavati-Niasari, Carbohydr. Polym., 2020, 229, 115428 CrossRef CAS PubMed.
- M. Kim, H. Lee, M. Kim and Y. C. Park, Nanomaterials, 2021, 11, 222 CrossRef CAS PubMed.
- J. Wu, Z. Du, P. Xiong and Z. Cai, Cellulose, 2020, 27, 6353–6366 CrossRef CAS.
- Q. Lyu, B. Peng, Z. Xie, S. Du, L. Zhang and J. Zhu, ACS Appl. Mater. Interfaces, 2020, 12, 57373–57381 CrossRef CAS PubMed.
- A. Zaitoon, L.-T. Lim and C. Scott-Dupree, Food Hydrocolloids, 2021, 112, 106313 CrossRef CAS.
- B. Niu, L. Zhan, P. Shao, N. Xiang, P. Sun, H. Chen and H. Gao, Int. J. Biol. Macromol., 2020, 142, 592–599 CrossRef CAS PubMed.
- S. Yi, Y. Wu, Y. Zhang, Y. Zou, F. Dai and Y. Si, ACS Sustainable Chem. Eng., 2020, 8, 16775–16780 CrossRef CAS.
- J. Knapczyk-Korczak, J. Zhu, D. P. Ura, P. K. Szewczyk, A. Gruszczyński, L. Benker, S. Agarwal and U. Stachewicz, ACS Sustainable Chem. Eng., 2021, 9, 180–188 CrossRef CAS.
- J. J. Kaschuk, K. Miettunen, M. Borghei, E. Frollini and O. J. Rojas, Cellulose, 2019, 26, 6151–6163 CrossRef CAS.
- N. Angel, S. N. Vijayaraghavan, F. Yan and L. Kong, Nanomaterials, 2020, 10, 1329 CrossRef CAS PubMed.
- D. Lv, G. Tang, L. Chen, M. Zhang, J. Cui, R. Xiong and C. Huang, ACS Appl. Polym. Mater., 2020, 2, 5686–5697 CrossRef CAS.
- E. Buyukada-Kesici, E. Gezmis-Yavuz, D. Aydin, C. E. Cansoy, K. Alp and D. Y. Koseoglu-Imer, Mater. Sci. Eng., B, 2021, 264, 114953 CrossRef CAS.
- R. Awad, A. Haghighat Mamaghani, Y. Boluk and Z. Hashisho, Chem. Eng. J., 2021, 410, 128412 CrossRef CAS.
- J. Cui, Y. Wang, T. Lu, K. Liu and C. Huang, J. Colloid Interface Sci., 2021, 597, 48–55 CrossRef CAS PubMed.
- N. Dizge, E. Shaulsky and V. Karanikola, J. Membr. Sci., 2019, 590, 117271 CrossRef CAS.
- A. Ojstršek, D. Fakin, S. Hribernik, T. Fakin, M. Bračič and M. Kurečič, Carbohydr. Polym., 2020, 236, 116071 CrossRef PubMed.
- G. Papaparaskeva, M. M. Dinev, T. Krasia-Christoforou, R. Turcu, S. A. Porav, F. Balanean and V. Socoliuc, Nanomaterials, 2020, 10, 517 CrossRef CAS PubMed.
- C. Cui, C. Xiang, L. Geng, X. Lai, R. Guo, Y. Zhang, H. Xiao, J. Lan, S. Lin and S. Jiang, J. Alloys Compd., 2019, 788, 1246–1255 CrossRef CAS.
Footnote |
† These authors contributed equally to this work. |
|
This journal is © The Royal Society of Chemistry 2021 |