DOI:
10.1039/D0PY00919A
(Minireview)
Polym. Chem., 2020,
11, 6988-7008
Advancing the stimuli response of polymer-based drug delivery systems for ocular disease treatment
Received
25th June 2020
, Accepted 23rd September 2020
First published on 25th September 2020
Abstract
The development of efficient therapies for ocular diseases remains a significant challenge because of the static and dynamic barriers in the eye. A variety of pharmaceutical strategies have been explored to overcome these ocular physiological barriers and thereby improve therapeutic bioavailability in both anterior and posterior ocular tissues. This mini-review summarizes, analyzes, and discusses recent advances in the field of ophthalmic drug delivery systems (DDSs). Specifically, the focus is on design strategies using stimuli-responsive polymers and their applications for the treatment of prevalent ocular diseases such as dry eye, ocular infection, glaucoma, and age-related macular degeneration. The stimuli-responsive polymers are categorized according to their responses in various ocular environmental conditions (such as temperature, pH, and ions). Additionally, general strategies and methodologies for the construction of effective ophthalmic stimuli-responsive DDSs are investigated by exploiting key parameters such as the stimuli-response type, ocular biocompatibility, ocular biodegradability, drug encapsulation and release, as well as the modifiable structure of the polymers. Also discussed in this review are the interrelationships among the designed structures, properties, and functions of the stimuli-responsive DDSs and their pharmacological treatment efficacies. In summary, we believe that the recent progress in the field of stimuli-responsive DDSs constitutes a significant advance for the development of effective pharmacological treatments for eye disorders.
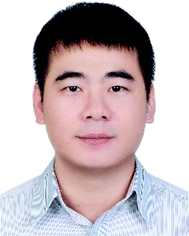 Duc Dung Nguyen | Duc Dung Nguyen received his Ph.D. in Materials Science & Engineering from National Tsing Hua University in 2012, before which he graduated from Vietnam National University (Hanoi) in 2004 with a B.S. degree in Physics. Dr Nguyen's research interest has been focused on (i) the development of polymer-based drug delivery systems for ophthalmic therapy and (ii) manipulation of carbon materials toward applications in flexible transparent electronics and contaminated water treatments (one representative work was inducted as a moonshot project by Google Solve for X in 2016). He has authored and co-authored more than 26 peer-reviewed articles with over 1100 citations. |
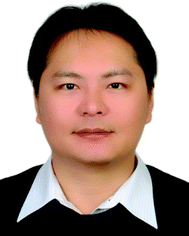 Jui-Yang Lai | Dr Jui-Yang Lai received his Ph.D. from the Department of Chemical Engineering, National Tsing Hua University, Taiwan. Since 2014, he has been a Full Professor at Chang Gung University, Taiwan. Dr Lai's primary research activities are centered on the design and development of functional biomaterials, either from natural or synthetic sources, for ophthalmic use, particularly on tissue engineering, drug delivery, and nanomedicine. His major research projects involve ocular biocompatibility assessment, corneal/retinal cell construct fabrication, topical/intraocular pharmaceutical dosage formulation, and metallic/carbon-based nanotherapeutic evaluation. Dr Lai has published more than 100 scientific papers (3000 citations, h-index = 32) and filed numerous patent applications. He actively participates in the peer review process for scientific publications (over 800 manuscripts) and also serves as a member of the editorial board of several scholarly journals. |
1. Introduction
Individuals with ocular diseases have reduced economic and educational opportunities, lower quality of life, and increased risk of death when compared with healthy individuals.1 In 2015, it was estimated that the number of people with moderate-to-severe vision impairment and blindness was approximately 252.6 million, which corresponds to 3.45% of the total 7.33 billion people living on the planet.1 Because these numbers continue to increase at accelerating rates (Fig. 1), vision disorders are becoming a leading cause of global disability. Therefore, ocular diseases are currently considered a serious global healthcare issue by the World Health Organization (WHO).
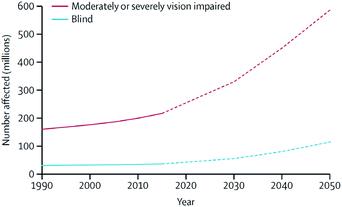 |
| Fig. 1 Global trends and predicted numbers of people suffering from moderate/severe vision impairment and blind (1990–2050). Reproduced from ref. 1 with permission from Elsevier, copyright 2017. | |
Despite the availability of effective treatments for ocular diseases, the implementation of these solutions is hampered by insufficient methods to administer drugs for long periods, increased risk of inflammation, and the high cost of ocular surgery.2 To address these challenges, considerable efforts have been focused on the development of cost-effective drug delivery systems (DDSs) that are more suitable for patients, have reduced side-effects, and are affordable for people living in developing countries.2–4 However, the efficient delivery of bioactive substances (drugs/therapeutics) to sites of pharmacological action remains a significant challenge. This can be ascribed to the complex anatomical structure of the eye, which has multiple static, dynamic, and metabolic barriers.3,4
Polymeric materials have been used as the main component in various DDSs, possibly due to their excellent properties, which include high water absorption capability, structural stability in aqueous media, excellent biocompatibility, and resemblance to living tissues.5–7 Generally, the encapsulation of drug molecules by polymeric carriers is controlled by van der Waals interactions, hydrogen bonds, π–π conjugations, electrostatic forces, or chemical linkages. However, an accurate mechanism has not been completely elucidated, and this means that empirical results are utilized to demonstrate the loading of drug molecules into polymeric carriers.8 It is worth noting that most of the common biomaterials employed in DDSs are in static regimens. This means that a driving force is required to trigger the release of drugs required for achieving therapeutic effects. In this context, the development of stimuli-responsive polymers (SRPs), which can respond to slight changes in ocular environments, has proven to be greatly beneficial for triggering the release of ophthalmic drugs in a sustained manner and provides an improved method for treating ocular diseases. In the last three years, there have been a number of review articles highlighting SRPs for various applications such as biosensors and artificial muscles9 and injectable/stimuli-responsive hydrogel-based DDSs10–12 or emphasizing on the role of degradable polymeric nanoparticles in ocular DDSs.13 However, a review that presents the design, modification, and construction of SRPs into various forms (not limited to hydrogels) for improving the uses of ophthalmic DDSs and their structural effects on the effectiveness of eye disease treatments has not been reported yet.
In this review, we summarize the recent progress in the development of SRP-based DDSs (SRP-DDSs) for the treatment of ocular diseases and present representative SRPs and the response mechanisms to physiological temperature, pH of the biological fluids, and ion composition of tears. The key roles of SRPs in the construction of efficient ophthalmic DDSs are analyzed and discussed. Moreover, we provide general requirements for SRPs and design rules to build SRP-DDSs, which allow for controlled physicochemical performances. The applications of SRP-DDS in the treatment of prevalent ocular diseases such as dry eye (DE), ocular infection (OI), glaucoma (GL), and age-related macular degeneration (MD) will be discussed with a special emphasis on the correlation among the designed structures, properties, functions, and treatment efficacies. Additionally, we present recent advances in the establishment of novel ophthalmic DDSs. These include the chemical combination of SRPs with functional biomaterials to establish DDSs with advanced functions and therapeutic effects (such as anti-inflammation and anti-oxidation). Finally, we provide concluding remarks on the design and application of SRP-DDSs for the management of ocular diseases, and we suggest perspectives on manipulating the structure–property–function relationships of stimuli-responsive materials for innovative therapeutic strategies.
2. Roles of SRPs in ophthalmic SRP-DDSs and their classification
2.1. Design rules and polymer sources for ocular SRP-DDSs
Fundamentally, SRP-based carriers for ophthalmic drugs need to encapsulate large amounts of drug molecules in a highly efficient manner. The SRPs must be ocular-biocompatible, biodegradable, and safe. They must also avoid the limitations of traditional ophthalmic carriers such as eye irritation, blurred vision, and adverse side effects. It is expected that SRPs can behave as pseudoplastic fluids with thixotropic characteristics that exhibit low fluid viscosity under high shear rate conditions. These characteristics enable drug distribution across the ocular surface upon blinking and transform to a highly viscous fluid under low shear rate conditions, thus extending the drug residence time.14,15 Depending on specific ocular diseases and administration routes, the effects of environmental stimuli (surrounding the lesion sites) will be determined, and appropriate SRPs will be exploited for the development of effective ophthalmic SRP-DDSs. Ideal properties of ophthalmic SRP-DDSs together with the possible modes of property improvement are summarized as follows:
• Stimuli response. The stability of the ocular environmental factors (such as pH, temperature, and ions) in diseased eyes should be confirmed to guarantee proper in vivo responses of intentionally used SRPs.
• Ocular biocompatibility. The selected SRPs must be compatible with the eye and ocular cells or tissues of interest (such as targeted sites or diseases) in both sol and gel phases.
• Ocular biodegradability. Since the eye is one of the smallest organs of the entire body, SRPs should be degradable in ocular environments to prevent blocking of ocular fluid flows and to avoid possible infections. If the SRPs are non-degradable, incorporation of additional biopolymers that possess good ocular biodegradability can increase the safe use of SRP-based carriers. On the other hand, the selected SRPs should be inert to ocular metabolic activities.
• Drug encapsulation and release. Phase transition characteristics of SRPs are the key factors involved in the regulation of drug loading and release. A rapid response to stimuli can lead to the encapsulation of limited drug amounts, while a slow response results in burst drug release that may cause toxic effects. SRPs with the appropriate response to ocular environments can be manipulated via synergistic combinations with other polymers (for example, combining a rapid SRP with a non-SRP or slow SRP or wrapping a slow SRP in a biopolymer matrix).
• Modifiable structure. The selected SRPs should possess chemical structures that can be easily functionalized with specific chemicals or polymers that can enhance the ophthalmic DDSs with the desired biological and physicochemical properties, thereby improving therapeutic outcomes. The ideal functional biomaterials should be capable of entrapping ophthalmic drugs (i.e., enhancing drug encapsulation efficiency), resisting dynamic ocular fluids with robust mucoadhesive performance, binding to ocular tissues of interest via targeted delivery, and even providing extra-therapeutic benefits that are not offered by currently available ophthalmic drugs.
Ophthalmic SRP-DDSs can be designed with different types of polymeric biomaterials, including natural or synthetic polymers or their combination (Fig. 2). Proteins, carbohydrates, and nucleic acids are common sources of natural polymers with intrinsically responsive properties. Recent substantial progress in polymer science and technology has resulted in the development of various synthetic polymers that mimic the stimuli response of natural biopolymers.16,17 Polyacrylamides such as poly(N-vinylcaprolactam) and poly(lysine) are representative synthetic SRPs. The SRPs in the ophthalmic DDSs can be chemically engineered in a variety of forms, including hydrogels, nanogels, micelles, particles, and films, depending on the intended purposes and administration routes. For example, to develop an SRP-DDS for the treatment of glaucomatous eyes via intracameral injection, PNIPAAm (a synthetic polymer) is covalently grafted to gelatin (a natural polymer) to form an injectable and biodegradable hydrogel solution that can transform into a gel upon a thermal change mimicking the difference between room and physiological temperatures (Fig. 3).18 Generally, SRP-DDSs developed using multiple polymeric components exhibit better drug release characteristics and improved treatment efficacies, possibly due to the synergistic effects and additional features.19,20 Considering the intriguing biological effects of inorganic nanoparticles, incorporating these inorganic nanomaterials with SRPs would be of great interest for the development of ophthalmic SRP-DDSs with new features such as remote-controlled drug delivery.21,22 However, the toxicity of nanomaterials is an obstacle that needs to be overcome because the small nanoparticle size allows for easy access to the cells and intracellular compartments, including the nucleus, which can have detrimental effects.23,24
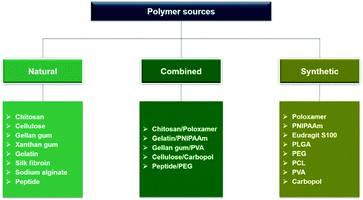 |
| Fig. 2 The flowchart outlining the polymer sources for construction of ophthalmic SRP-DDSs. | |
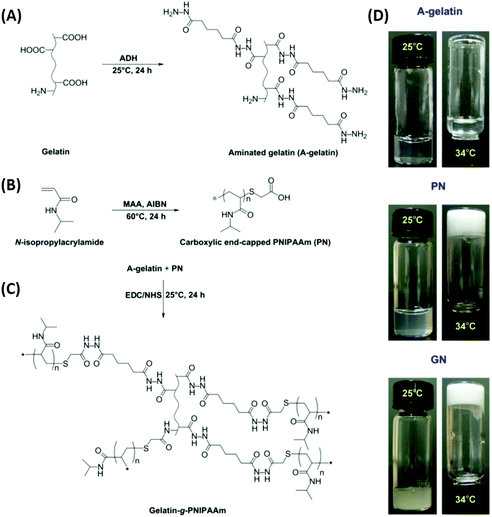 |
| Fig. 3 Schematic reaction diagrams for the development of a biodegradable thermo-sensitive hydrogel via combination of synthetic and natural polymers. Reaction scheme for (A) aminated gelatin (A-gelatin), (B) carboxylic end-capped PNIPAAm (PN), and (C) gelatin-g-PNIPAAm (GN). Typical photographs (D) demonstrate the sol–gel phase transition based on the thermal response. Reproduced from ref. 18 with permission from Elsevier, copyright 2011. | |
2.2. Classification of SRPs
Generally, the viscosity of ophthalmic formulations is manipulated to improve ocular drug bioavailability.14 This change in viscosity enhances their resistance to various physiological barriers such as lacrimal drainage, tear fluid, conjunctival blood, lymphatic clearance, choroidal blood, and lymph circulation. Emulsions and ointments have been used to increase drug retention and improve drug bioavailability on the ocular surface. Unfortunately, these drug carriers caused adverse effects such as irritation, redness, and blurriness.25 To counter these challenges, SRP-based biomaterials have been explored as alternatives. These biomaterials reduce side-effects and increase patient comfort while enhancing therapeutic effects.26,27 These SRP-based ophthalmic formulations are produced in solution form and transformed into a gelled state once they are administered to the eye. This sol–gel transition can be regulated by the ocular environmental conditions, including physiological temperature (thermo-responsive), ocular fluid pH (pH-responsive), and tear fluid ion concentration (ion-responsive). Compared to traditional/non-SRP formulations, the in situ gelling formulations can provide several advantages. These include: (i) sustained drug release, as the gel is formed once its corresponding sol is placed in ocular environments, allowing for prolonged confinement of drug molecules; (ii) protection of drug molecules from degradation, metabolism, and cellular efflux during the delivery time-course; and (iii) the use of soluble formulations that are simple and user friendly.
2.2.1. Thermo-responsive polymers.
Thermo-responsive polymers, the most widely investigated SRP materials, are characterized by a phase transition within a specific temperature range in response to the formation of intermolecular hydrogen bonds, hydrophobic interactions, and physical entanglements of polymer chains.28 Considering that the physiological temperatures of the eye are typically in the range of 32–35 °C,29 the ophthalmic use of thermo-responsive polymers with phase transition temperatures around room temperature (∼25 °C) is allowed, i.e., SRP-DDSs can be stored at ambient temperature. The development of SRP-DDSs based on the thermal gradient between the ambient and ocular environment temperatures will be beneficial for the pharmacological treatment of ocular diseases.
The most popular thermo-responsive polymer that is currently in use is poly(N-isopropylacrylamide) (PNIPAAm), which has a reversible coil-to-globule transition in aqueous solutions at its lower critical solution temperature (LCST) of 32 °C.30 The hydrophilic and hydrophobic groups present in this thermo-sensitive polymer are responsible for the phase transition. Below the LCST, PNIPAAm is water-soluble, while above this temperature, the polymer forms inter/intra-chain associations that result in the polymer being insoluble in aqueous media.26 Interestingly, the LCST of PNIPAAm, mainly determined by the hydrogen bonding between water and monomer units (such as N–H or C
O linkages), can be tailored by combining with either hydrophilic or hydrophobic monomers.31 Considering that PNIPAAm is a non-biodegradable polymer, proper incorporation with biodegradable biomaterials is needed to ensure the safe use of the resultant DDSs.
Biodegradable and thermo-responsive polymers have been developed by incorporating chitosan, which is a polysaccharide derived from chitin by alkaline hydrolysis, into polyol-phosphate chains. The thermo-responsive chitosan is prepared by adding β-glycerophosphate (GP) to a chitosan solution, resulting in a mixture with a reversible sol–gel phase transition between both ambient and physiological temperatures.32 The phase transition temperature is independent of the molecular weight of chitosan but is strongly affected by the GP concentration. The gelling mechanism for the chitosan/polyol-phosphate systems can be explained by the formation of a hydration protective layer around chitosan backbone chains via weak intermolecular interactions. As the temperature increases, the polyol protective layer is damaged and allows the polymer to interact through stronger bonds (such as hydrophobic interactions), resulting in gelation.33 Although the polyol-phosphate/chitosan biomaterial is one of the most widely investigated thermo-sensitive polymers, it has some disadvantages, including low mechanical strength and a slow temperature response. To address these, improvements such as synergistic combinations with other biomaterials are required.34
Biodegradable thermo-responsive materials can also be constructed using copolymerization methods. Poloxamers, commercially known as Pluronic®, are synthetic triblock copolymers comprising a hydrophobic block of poly(propylene oxide) (PPO) sandwiched by two hydrophilic blocks of poly(ethylene oxide) (PEO). The triblock structure (PEO–PPO–PEO) thus endows the thermo-sensitive copolymers with amphiphilic properties.35 This amphiphilic nature enables the poloxamer macromolecules to aggregate into micelles, which contain a hydrophobic core and a hydrophilic shell, in aqueous solutions. At sufficient concentrations, poloxamer aqueous solutions exhibit thermo-responsive phase transition behavior via polymer desolvation, micelle aggregation, and network entanglement.36 Pluronic F-127, which consists of ethylene oxide (70%), is a poloxamer with low viscosity (below 4 °C) and it is able to form a semi-solid gel at body temperature.37,38 Several types of poloxamer products have been approved by the Food and Drug Administration (FDA) and are used in various pharmaceutical formulations because of their good biocompatibility and biodegradability. In addition to poloxamers, alternative triblock copolymers built from hydrophilic poly(ethylene glycol) (PEG) and hydrophobic poly(lactic acid-co-glycolic acid) (PLGA) have been extensively investigated. Aqueous PLGA–PEG–PLGA has been reported to be in solution form at temperatures between 2 and 15 °C and it transforms into a gel at physiological temperatures.39 These thermo-responsive copolymers are developed with adjustable degradation rates because the molar ratios between the two monomers are varied. This characteristic makes them great candidates for the development of SRP-DDSs with controlled drug release profiles. Additionally, both PEG and PLGA are approved by the FDA, which means that the PLGA–PEG–PLGA thermo-responsive polymers are bound to be highly biocompatible.
2.2.2. pH-Responsive polymers.
pH-Responsive polymers are polyelectrolytes containing weakly acidic or basic groups that accept or donate protons in response to changes in the pH of the surrounding media. These changes in pH are responsible for the sol–gel phase transitions. Considering that the pH of the ocular surface in the normal eye is neutral (pH 7.4) and that a variety of pathological conditions can alter this pH value, the use of pH-responsive polymers in ophthalmic DDSs is of great interest.40 Carbopol, the most widely investigated pH-responsive polymer in ophthalmic DDSs, is a poly(acrylic acid) (PAA) polymer that exhibits sol states at a pH lower than 5.5 and transforms into gels at higher pH values. The occurrence of this pH-induced sol–gel phase transition is attributed to electrostatic repulsion and osmotic forces within the backbone chain.41 Moreover, carbopol is reported to possess highly mucoadhesive properties due to the strong interactions between the polymer and mucin via electrostatic forces, hydrogen bonds, hydrophobic conjugation, and interdiffusion.42 These properties demonstrate that carbopol has the potential for various practical applications on the ocular surface. A major limitation of carbopol is its acidic nature, which causes irritation and toxicity to the eye.43 Accordingly, a strategy for reducing the amount of carbopol without the elimination of sol–gel transition behavior is urgently needed for the development of effective carbopol-based DDSs. In addition to carbopol, chitosan (because of its cationic nature) can be dissolved in acidic aqueous media in the sol state and it transforms into the gel state when neutralized, exhibiting a sol–gel phase transition at a pH of 6.2.32 This phenomenon suggests that aqueous chitosan solutions can be gelled quickly upon contact with ocular environments whose pH values are greater than or equal to 6.2.40
2.2.3. Ion-responsive polymers.
Ion-responsive polymers are materials that can change their shapes in response to ionization/deionization of functional groups on polymeric chains. Given that the tear fluid is composed of various cations such as Na+, Mg2+, and Ca2+, ion-responsive polymer solutions, when in contact with the eye, can be transformed into gel states via ionic bonds created within the polymeric backbone chain.44,45 The most extensively investigated ion-responsive polymer for the development of ophthalmic DDSs is gellan gum (commercially known as Gelrite®). Gellan gum is an anionic linear polysaccharide that consists of repeating units of tetrasaccharide that comprise two units of D-glucose and one unit of D-glucuronic acid and L-rhamnose. The hydroxyl and carboxylic groups allow possible interactions with other polymers via hydrogen bonds and electrostatic forces.46 An aqueous solution of gellan can transform into a clear gel when interacting with typical cations found in the tear fluid. It has been shown that the slightly viscous gellan gum solutions (concentrations <1%) could change to highly viscous states when interacting with cations at a physiological level, and this reaction does not require more than 10–20% of the ions found in the tear fluid.47 In addition to gellan, xanthan gum is another popular ion-responsive polymer employed for ocular DDSs. Xanthan gum comprises pentasaccharide repeating units of mannose, glucose, and glucuronic acid.48
3. Pharmacological treatment of ocular diseases using SRP-DDSs
3.1. Overview
The effective delivery of ophthalmic drugs is a challenging issue to date because of the static, dynamic, and metabolic barriers of the eye.3,4 Static barriers are physical impediments that drug molecules must pass through, and these include the cornea, conjunctiva, and sclera. The clearance actions caused by both tear and intraocular fluids are typical dynamic barriers that prevent the delivery of drugs to target sites. Metabolic barriers in the eye, such as esterases and carbonic anhydrase enzymes, can reduce effective therapeutic activity by deactivating drugs. Consequently, these ocular barriers (Fig. 4A) result in short pre-corneal residence times and low drug concentrations at pharmacological sites of action.
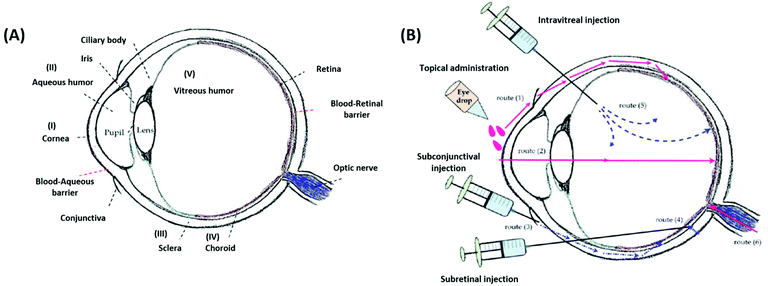 |
| Fig. 4 Schematic illustrations of the eye anatomy with various ocular barriers (A) and administration approaches (B) for the treatment of different ocular diseases. Reproduced from ref. 13 with permission from MDPI, copyright 2018. | |
The human eye is a tiny organ weighing approximately 7.5 g with a globe diameter of about 24 mm in adults. The eye has a sophisticated structure and is composed of various tissues with different characteristics. Malfunction of any of these tissues can result in vision loss or blindness.49 There is a wide range of pathologies that can affect the eye, and these include local injuries or the manifestation of diabetes mellitus.50 Low drug bioavailability (due to physiological barriers) is a major issue for the delivery of ophthalmic drugs via conventional formulations. Consequently, frequent drug administrations are required to maintain the therapeutic effects in target tissues, and thereby they may induce adverse effects.51 Therefore, the development of SRP-DDSs with advanced functions is expected to produce ophthalmic formulations that can prolong the drug release and provide additional therapeutic effects for improving patient comfort and enhancing drug bioavailability while reducing overall treatment costs.
It is worth noting that the multiple physiological barriers (to the drug) have a strong influence on the route of administration selected.52 Typical administration routes for ophthalmic formulations are topical, intraocular, and periocular (Fig. 4B). Topical administration is the most widely used noninvasive method because of the ease of use and patient compliance. However, it is limited by low drug bioavailability due to dynamic barriers (such as tear fluid turnover and reflex blinking) as well as static barriers (such as the cornea and conjunctiva).13 Therefore, this noninvasive route of administration is commonly employed to treat eye diseases that occur in the anterior rather than the posterior segment of the eye. To achieve greater drug delivery efficiencies at lesion sites located in the posterior segment, intraocular injection or implantation is commonly used. However, the requirements for repeated puncture or surgery can cause several side effects such as bacterial infection, endophthalmitis, hemorrhage, and retinal detachment, in addition to patient inconvenience.53,54 Periocular administrations, including subconjunctival and subtenon injections, are alternatives that allow formulations to bypass the superficial tear fluid and conjunctival barriers. These routes can leave ophthalmic formulations within the spaces between tissue layers.50 Moreover, periocular injections can deliver drugs to the posterior segment through the anterior segment, and they are particularly appropriate for poorly water-soluble drugs such as steroids.55 However, these periocular routes also have several limitations, including invasiveness, patient discomfort, or hemorrhage. Therefore, understanding the nature of ocular barriers in association with specific target tissues and administration routes is vital for the establishment of advanced SRP-DDSs toward efficient management of ocular diseases.
In the following subsections, recent applications of SRP-DDSs to administer pharmacotherapies for prevalent ocular diseases (such as DE, OI, GL, and MD) that occur in both the anterior and posterior segments of the eye are summarized (Table 1) and discussed. The relationship between the treatment efficacies and the designed structures, properties, and functions of SRP-DDSs is also emphasized.
Table 1 Summary of stimuli-responsive polymer-based drug delivery systems (SRP-DDSs) for the treatment of ocular diseases
Polymer |
Condition-responsive manner |
Drug |
Administration route |
Action mechanism |
Delivery/therapeutic performance |
Ref. |
Dry eye (DE) |
Elastin-like polypeptide |
Temperature |
Lacritin |
Intralacrimal |
Tear secretion-promoting |
Sustain drug release for 24 h inside the lacrimal gland (12 fold greater as compared to the free drug). Increase tear secretion by approx. 1.2 fold (compared to the free drug) |
61
|
Chitosan/poloxamer |
Temperature |
Insulin |
Conjunctival |
Tear secretion-promoting/tissue-healing promoting |
Sustain drug release (2.5 fold longer than free drug solution). Increase tear secretion by 1.4 fold (compared to free drug solution). Recover tear fluid volume and corneal thickness to normal conditions |
62
|
Gelatin/PNIPAAm |
Temperature |
Epigallocatechin gallate |
Conjunctival |
Antiinflammatory/antioxidative |
Increase antiinflammatory and antioxidant activities by approx. 4 fold (compared to the free drug). Alleviate disease progression for 3 days by a single instillation |
63
|
Eudragit S100 |
pH |
Cyclosporine |
Corneal/conjunctival |
Antiinflammatory |
Sustain drug release (1.75 fold longer than a non-pH-sensitive formulation) |
65
|
Gellan gum/HPMC |
Ion |
Vitamin A/E |
Conjunctival |
Antiinflammatory/antioxidative |
Possess similar physicochemical properties to the tear film (e.g., pH of 7.6, viscosity of 4.0 mPa s, surface tension of 53.4 mN m−1, etc.). Extend the ocular residence time of the formulation |
67
|
Ocular infection (OI) |
Chitosan/β-glycerolphosphate |
Temperature |
Moxifloxacin/gentamicin |
Corneal |
Antibacterial |
Decrease intervention times by greater than 4 fold as compared to the marketed drug |
74
|
Pluronic/HPMC |
Temperature |
Sertaconazole |
Corneal |
Antifungal |
Suppress bacterial growth at a concentration of 2.5 μg mL−1 at which free drug concentrations are insufficient to exhibit any inhibitory effects |
77
|
PLGA/PEG |
Temperature |
Voriconazole |
Subconjunctival |
Antifungal |
Sustain drug release to the cornea and sclera at concentrations above the minimum inhibitory concentration for up to 48 h |
79
|
Chitosan/poloxamer |
Temperature |
Tobramycin |
Conjunctival |
Antibacterial |
Increase drug bioavailability in aqueous humor by approx. 8 fold (compared to the marketed drug). Sustain therapeutic concentration over 24 h (single dose) |
80
|
Chitosan/poloxamer |
Temperature |
Neomycin/betamethasone |
Conjunctival |
Antibacterial/antifungal |
Sustain the release of drugs for over 10 h and inhibit microbial growth for 7 days |
81
|
Chitosan/gelatin |
Temperature |
Levofloxacin |
Conjunctival |
Antibacterial |
Extend drug release and prolong antibacterial inhibitory effects over 7 days |
82
|
Pluronic |
Temperature |
Cefuroxime |
Intravitreal |
Antifungal |
Sustain drug release in the simulated vitreous humor up to one week |
83
|
PEGDA/PNIPAAm |
Temperature |
Vancomycin |
Subconjunctival |
Antibacterial |
Sustain drug release for 2 weeks. Lower infection scores after 24 h compared to treatment using drug solution |
84
|
HPMC/sodium alginate |
pH |
Levofloxacin |
Conjunctival |
Antibacterial |
Sustain antibacterial activity for more than 24 h |
87
|
Chitosan/gellan gum/PVA |
pH/temperature/ion |
Besifloxacin |
Conjunctival |
Antibacterial/antifungal |
Sustain drug release into aqueous humor for 12 h (3 fold longer than the marketed drug) |
89
|
Gelrite |
Ion |
Moxifloxacin |
Conjunctival |
Antibacterial |
Increase drug bioavailability in aqueous humor by 6 fold (compared to the marketed drug). Treat effectively the corneal infection after 4 days |
90
|
Gellan gum |
Ion |
Levofloxacin |
Conjunctival |
Antibacterial |
Increase therapeutic effective time by 2 fold (compared to the marketed drug) |
27
|
Gelrite |
Ion |
Levofloxacin |
Conjunctival |
Antibacterial |
Sustain inhibitory activity against S. aureus. Decrease intervention times by 4 fold (compared to the marketed drug) |
93
|
Gellan gum |
Ion |
Terbinafine |
Conjunctival |
Antifungal |
Increase drug bioavailability in aqueous humor by 2.67-fold (compared to the marketed drug) |
94
|
Gellan gum/κ-carrageenan |
Ion |
Econazole-α-cyclodextrin |
Conjunctival |
Antifungal |
Increase retention time on the ocular surface by approx. 2.5 fold (compared to the marketed drug) |
95
|
Glaucoma (GL) |
Gelatin/PNIPAAm |
Temperature |
Pilocarpine |
Intracameral |
IOP-reducing |
Sustain drug release and lower elevated IOP to normal values for 56 days |
99
|
Chitosan/PNIPAAm |
Temperature |
Pilocarpine |
Intracameral |
IOP-reducing |
Sustain drug release and lower elevated IOP to normal values for over 63 days |
100
|
PLGA/PEG/PNIPAAm |
Temperature |
Brimonidine |
Subconjunctival |
IOP-reducing |
Reduce 55 intervention times while providing comparable efficacies in reducing IOP for 28 days (single time administration equal to 56 instillation times of marketed eye drops) |
101
|
Gelatin/chitosan/β-glycerolphosphate |
Temperature |
Latanoprost |
Subconjunctival |
IOP-reducing |
Sustain drug release over 28 days and lower elevated IOP to normal for 31 days by single dose administration |
103
|
Gelatin/chitosan/glycerolphosphate |
Temperature |
Latanoprost |
Conjunctival |
IOP-reducing |
Sustain drug release and increase IOP reduction efficiency by 7 fold (compared to the marketed drug) |
104
|
Elastin/silk fibroin |
Temperature |
Timolol |
Conjunctival |
IOP-reducing |
Sustain drug release and increase IOP reduction efficiency by 2 fold (compared to the marketed drug) |
105
|
Poloxamer |
Temperature |
Timolol |
Conjunctival |
IOP-reducing |
Increase IOP reduction efficiency by 2 fold (compared to the marketed drug) |
106
|
PLGA/PEG/PLGA |
Temperature |
Brimonidine |
Corneal/conjunctival |
IOP-reducing |
Sustain drug release for 168 h and increase IOP reduction efficiency by approx. 5 fold (compared to marketed eye drops) |
107
|
PLGA/PEG/PLGA |
Temperature |
Cyclosporine A |
Subconjunctival |
IOP-reducing/scar-inhibiting |
Lower elevated IOP and maintain at normal values for over 70 days |
108
|
PEG/PCL/PEG |
Temperature |
Bevacizumab |
Intracameral |
IOP-reducing/bleb survival-promoting |
Increase effective time of IOP reduction by approx. 4.7 fold. Maintain subconjunctival space at larger sizes after 28 days of surgery. Show negligible amounts of fibroblasts, hyperplasia and new collagen tissue deposition |
109
|
Pluronic |
Temperature |
SB-505124 |
Subconjunctival |
Scar-inhibiting/bleb survival-promoting |
Sustain drug release for 10 h without initial burst. No severe complications are observed after 4 days of glaucoma filtration surgery |
110
|
PTMC/Pluronic/PTMC |
Temperature |
Mitomycin C |
Subconjunctival |
Scar-inhibiting/bleb survival-promoting |
Sustain drug release for more than 2 weeks and maintain mean blebs at larger sizes for over 28 days (compared to the free drug) |
111
|
Carbopol/HPMC |
pH |
Dorzolamide |
Conjunctival |
IOP-reducing |
Increase IOP reduction efficiency by approx. 6 folds (compared to marketed eye drops) |
112
|
Carbopol/HPMC |
pH |
Brimonidine |
Conjunctival |
IOP-reducing |
Increase IOP reduction efficiency by >2 fold (compared to the marketed drug) |
113
|
Gellan gum |
Ion |
Brinzolamide |
Conjunctival |
IOP-reducing |
Sustain drug release and increase IOP reduction time by 3 fold (compared to the marketed drug) |
114
|
Gellan gum |
Ion |
Brinzolamide |
Conjunctival |
IOP-reducing |
Sustain drug release and lower elevated IOPs for 48 h (compared to 6 h effectiveness by marketed eye drops) |
115
|
Gellan gum/xanthan gum, HPMC |
Ion |
Acetazolamide |
Corneal |
IOP-reducing |
Increase IOP reduction efficiencies by greater than 2 fold (compared to marketed eye drops) |
116
|
Macular degeneration (MD) |
PEG/PPG/PCL |
Temperature |
Bevacizumab/Aflibercept |
Intravitreal |
Antiangiogenic |
Sustain drug release for 40 days (compared to <10 days by the free drug). Inhibit angiogenesis in vivo over a period of at least 28 days |
121
|
PEG/PLGA/PEG |
Temperature |
Triamcinolone acetonide |
N/A |
Antiangiogenic |
Sustain drug release to 250 h from 5 h (drug only). Reduce VEGF expression by 43.5% as compared to 1.5% by the free drug |
122
|
PEG/PLLA/PNIPAAm |
Temperature |
Aflibercept |
N/A |
Antiangiogenic |
Sustain drug release over 6 months |
123
|
PEG/PSHU |
Temperature |
Bevacizumab |
Intravitreal |
Antiangiogenic |
Sustain drug release for over 9 weeks with concentrations approx. 5-fold higher in the eye (compared to the free drug) |
124
|
PEG/polyester polyol |
Temperature |
Bevacizumab/Aflibercept |
Intravitreal |
Antiangiogenic |
Sustain drug release over 183 days. No abnormality in the retinal structure/function is found after drug administration |
125
|
Gellan gum |
Ion |
Lutein |
Conjunctival |
Antioxidative |
Decrease the apoptosis rate of retinal cells approx. 6 fold (compared to the drug solution). Reduce elevated ROS levels in diseased retina to normal |
126
|
3.2. Dry eye syndrome
DE is the most frequent ophthalmic and multifactorial disease of both the tear film and the ocular surface, and it can lead to blurry vision, irritation, light sensitivity, and inflammation.56 The prevalence of DE can result in a low quality of life and impose a significant economic burden on patients. In the United States, approximately $55 billion is spent annually to treat this chronic ocular surface disease.57 Clinically, DE is diagnosed by tear fluid volume measurement, tear fluid breakup time, corneal staining, osmolarity, and symptom-based questionnaires. Anti-inflammatory molecules, ocular lubricants, artificial tears, and secretagogues are used as eye drops to treat DE.58 Employment of punctal plugs, which increase tear fluid retention on the ocular surface, has also been suggested as another treatment method for DE.59
3.2.1. Treatment based on thermo-responsive polymers.
Elastin-like polypeptides (ELPs) are inspired by the human tropoelastin protein and they exhibit an inverse phase transition behavior that can transform them from aqueous solutes into self-assembled nano/micro-particles as the temperature is varied from below to above their transition temperatures.60 Recently, ELPs have been used as thermo-responsive carriers to improve the DE treatment efficacy of lacritin, a protein exhibiting prosecretory activity in the lacrimal gland and mitogenic activity at the corneal epithelium.61 The ophthalmic formulation reveals the thermal response of the ELPs while maintaining the prosecretory efficacy of lacritin, as confirmed by its ability to stimulate the secretion of β-hexosaminidase by primary rabbit lacrimal gland acinar cells. Moreover, intra-lacrimal injection of the thermo-responsive formulation enhanced tear fluid secretion in a non-obese diabetic mouse model of autoimmune dacryoadenitis (Fig. 5). The enhanced prosecretory effect can be ascribed to the extended-release performance of lacritin from the thermo-responsive ELP-based DDS that forms a depot inside the lacrimal gland for 24 h, which is 12-fold greater than that of the intra-lacrimally injected therapeutic protein (Table 1).61 Mixed polymer solutions of chitosan and poloxamers are another example of ophthalmic thermo-responsive DDSs that enhance DE treatment efficacy via sustaining the drug release.62 The ocular bioavailability of topically administered insulin is considerably elevated in the lacrimal gland and eyeball of experimental rats when delivered by in situ thermal gelling chitosan/poloxamer carriers. Moreover, the topical administration of insulin-loaded chitosan/poloxamer formulations normalized the tear fluid volume, corneal thickness, and corneal cell morphology, demonstrating that this ophthalmic thermo-responsive formulation is a promising candidate for DE treatment. In addition to SRPs or SRP blends, covalent copolymers have also been exploited as thermo-responsive DDSs. For instance, a gelatin-graft-PNIPAAm (GN) copolymer, synthesized via carbodiimide coupling chemistry, was proven to be capable of encapsulating epigallocatechin gallate (EGCG) without any apparent changes in its phase transition temperature, biodegradability, and biocompatibility.63 Owing to its thermo-responsive nature, the in situ gelling GN copolymer allowed for a sustained release of EGCG for 3 days without deteriorating the potent anti-inflammatory and antioxidant effects of the drug. Pharmacological treatment applied using a single dose instillation of the EGCG-loaded GN carriers outperformed those using both EGCG and artificial tear solutions in alleviating DE in a rabbit model.63 This confirms the important role of the thermo-responsive copolymer in prolonging the release of EGCG via the in situ gelling behavior on the diseased ocular surface.
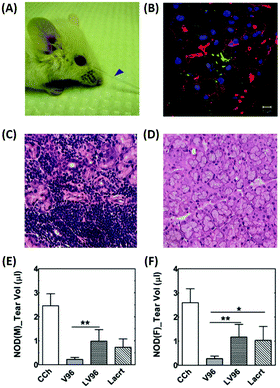 |
| Fig. 5 Intralacrimal injection of an ophthalmic formulation consisting of lacritin and thermo-responsive elastin-like polypeptides for enhancement of tear secretion in a mouse model of DE. (A) Representative pictures showing tear secretion after an intra-lacrimal injection; collected tear volume (blue arrow). (B) Lacrimal glands injected with the formulation were collected after infusion and visualized using immunofluorescence to identify lacritin. Green: anti-lacritin antibody; red: actin stained using Rho-phalloidin; blue: nucleus stained with DAPI. Scale bar: 10 μm. (C and D) Representative H&E staining images of mouse lacrimal glands. (C) Severe lymphocytic infiltration was observed in male mice. (D) Female NOD mice exhibited a normal morphology. (E and F) Quantification of tear volume displaying a significant enhancement of tear secretion by the formulation; (E) male and (F) female mice. Reproduced from ref. 61 with permission from Elsevier, copyright 2014. | |
3.2.2. Treatment based on pH-responsive polymers.
Eudragit S-100, a pH-responsive copolymer composed of methacrylic acid and methylmethacrylate, with a characteristic dissolution behavior at a pH > 7.0, has been explored as a nanocarrier for cyclosporine (an effective drug for the treatment of chronic DE syndrome).64 Specifically, the cyclosporine-loaded nanoparticles, synthesized via a quasi-emulsion solvent diffusion technique, were incorporated into a contact lens matrix to enhance sustained drug release at the ocular surface.65 The drug-loaded nanoparticles embedded in contact lenses did not show any leached or released drug in packaging solutions with a pH value below 7. To trigger the drug release, patients can remove the SRP-DDS from the packaging solution, wash with saline (pH = 7.4), and wear on the dry eyes, where the pH of tear fluid is higher than 7.0.66 The tear fluid diffuses into the contact lens matrix and dissolves the drug-loaded nanoparticles, consequently inducing the release of cyclosporine into the tear film of the diseased ocular surface. In vivo studies showed that the drug-laden contact lens SRP-DDS sustained the release of cyclosporine for 14 days, which is 1.75× longer than that with the DDS that did not incorporate the pH-responsive polymer nanocarriers.65 These results suggest that the use of pH-responsive polymeric materials combined with nanoparticles aid in preventing drug leakage during storage (in the packaging solution) and maintaining the optical transparency of the contact lens while at the same time extending drug release for the effective and straightforward treatment of DE.
3.2.3. Treatment based on ion-responsive polymers.
Considering that DE is associated with alteration and instability of the tear film, the principal method of management is to restore the tear film characteristics. Artificial tears have been used extensively for the treatment of mild to moderate DE and can be combined with other pharmacotherapies to cure severe DE syndrome. A large variety of artificial tear formulations with different components are currently available for purchase. However, most of these artificial tear formulations require repeated administrations due to short ocular residence time, as their composition is not similar to that of the human tear.67,68 To address these issues, gellan gum, an in situ gelling polymer, was combined with an artificial tear formulation consisting of liposomes, vitamins A and E, and hydroxypropyl methylcellulose (HPMC).68 Gellan gum plays a critical role in extending the ocular residence time of the artificial tear formulation by providing it with the characteristic of in situ gelling in response to contact with ions (ion-responsiveness). The addition of an ion-responsive polymer did not affect the physiological properties of the artificial tear formulation (which are a pH of 7.6, a viscosity of 4.0 mPa s, and a surface tension of 53.4 mN m−1), but enhanced the ocular retention time of the formulation. This strategy, based on the use of ion-responsive polymers in artificial tears, would be of immense benefit for the management of mild to moderate DE syndrome.
3.3. Ocular infection
Although the eye is relatively impermeable to microorganisms, ocular infections can quickly develop when exposed to various factors such as trauma, surgery, age, dryness of the eye, wearing of contact lenses, and prolonged use of topical steroids.69 Common ocular infections such as keratitis, conjunctivitis, and endophthalmitis, if left untreated, can induce the destruction of ocular structures and result in vision loss and blindness.70 To remedy this, conventional treatment approaches for ocular infections include topical instillation and intraocular injection of antimicrobial drugs, and in some cases, oral or intravenous antibiotics.71
3.3.1. Treatment based on thermo-responsive polymers.
Topical administration of antibiotics is a well-established therapy for microbial keratitis.72 However, it requires the continuous delivery of eye drops every 15 min for a period of up to 48 h. This is a major challenge for drug delivery and increases patient discomfort.73 To address this, chitosan/β-glycerolphosphate was investigated as a thermo-responsive polymeric carrier for the sustained delivery of antibiotics while also serving as an occlusive dressing that protects damaged corneas.74 Moxifloxacin and gentamicin released from the in situ thermal gelling chitosan/β-glycerolphosphate carrier exhibited antibacterial effects comparable to drug solutions alone. This result suggests that the inclusion of the antibiotics in the thermo-responsive polymer does not hinder their antibacterial treatment efficacy. Importantly, the thermo-responsive polymer allowed for the sustained release of antibiotics over 4 h, while drug solutions alone lasted for less than 1 h.
To achieve a more effective SRP-DDS, additional polymeric components are generally incorporated into the thermo-responsive polymers of interest.75,76 Improved mechanical properties can be obtained by blending hydroxypropylmethylcellulose (HPMC) with Pluronic F127 (PF127), which is a thermo-responsive copolymer that lacks satisfactory mechanical strength (thereby leading to rapid dissolution and burst release).77 The resultant PF127/HPMC thermo-responsive carrier allowed for the sustained release of sertaconazole, a potent imidazole derivative showing a wide spectrum of antifungal activities. The formulation suppressed bacterial growth at a concentration of 2.5 μg mL−1, possibly due to the increased drug penetration into the fungal cell wall to inhibit ergosterol synthesis. This is in contrast to the free drugs which do not exhibit any inhibitory effects at this concentration. Also, triblock PLGA–PEG–PLGA thermo-responsive copolymers are shown to be attractive candidates for the protection of the encapsulated drug from rapid degradation and burst release.78 Subconjunctival injection of a voriconazole-loaded triblock copolymer was able to deliver a sustained release of the drug to the cornea at concentrations above 0.5 μg g−1 (the target minimum inhibitory concentration for Aspergillus sp.) for up to 48 h.79 Similarly, a combination of a poloxamer and chitosan led to an approximately 8-fold increase of tobramycin in the aqueous humor (compared to the drug solution alone) and a sustained therapeutic concentration over 24 h with a single dose.80 The mixed chitosan/poloxamer has also been reported to promote the sustained release of neomycin/betamethasone for over 10 h and inhibit microbial growth for 7 days.81 The designed formulations show promise for the development of effective therapies for the treatment of conjunctivitis.
In addition to physical blending and copolymerization,82,83 the chemical cross-linking method was also employed to improve the drug delivery performance of the single thermo-responsive polymer. Recently, PNIPAAm cross-linked with poly(ethylene glycol) diacrylate (PEG-DA) has been investigated as a thermo-responsive DDS for the delivery of prophylactic vancomycin (VAN) used to treat S. aureus-induced endophthalmitis (Fig. 6 and Table 1).84 The PEG-DA/PNIPAAm copolymer released VAN at a steady rate for 2 weeks before reaching a plateau regimen after 3 weeks. The conjunctivas and corneas of animals receiving the VAN-loaded PEG-DA/PNIPAAm formulations were effectively treated as verified by the eyes without observable signs of infection (such as clouding of the cornea and redness in the conjunctiva) throughout the follow-up period of 72 h. These results were further confirmed by the results of histopathological sections of the vitreous body. Animals receiving a bolus VAN injection exhibited cell infiltration and disorganized tissue layers, which is an indication of infection.85 Subconjunctival injection of VAN-loaded PEG-DA–PNIPAAm thermo-responsive copolymers revealed that few inflammatory cells migrated into the vitreous body. Also, the layer structures appeared as normal, demonstrating the efficient management of S. aureus-induced endophthalmitis by the developed formulation.
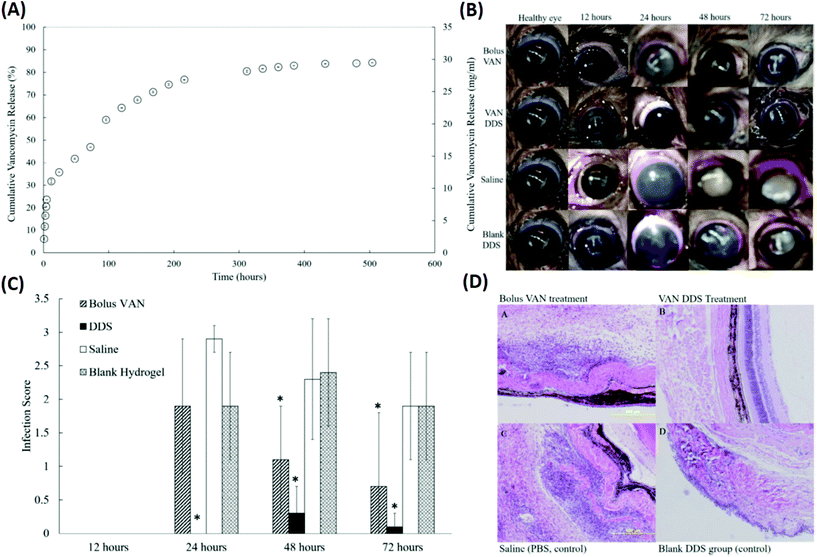 |
| Fig. 6 Development of a VAN-loaded PEG-DA/PNIPAAm thermo-responsive polymer for the treatment of S. aureus induced endophthalmitis. (A) A cumulative release profile of VAN from the PNIPAAm–PEG-DA thermo-responsive polymer. (B) Representative eyes for all four treatment groups (bolus VAN, VAN-loaded DDS, saline-loaded DDS, and blank DDS) at 12, 24, 48, and 72 hours. (C) Infection scores for all treatment groups at 12, 24, 48, and 72 hours after injection. (D) Representative histopathological sections from enucleated specimens stained with hematoxylin–eosin indicating a vitreous structure for rats in each experimental group 48 hours after infection: (a) bolus VAN group showing an infiltrated vitreous and disorganized tissue structure; (b) VAN-loaded DDS group showing a mostly non-infiltrated vitreous and normal, organized tissue structure; (c) saline group showing an infiltrated vitreous disorganized tissue layers; and (d) blank DDS group showing infiltrated vitreous and disorganized tissue layers. Reproduced from ref. 84 with permission from the Association for Research in Vision and Ophthalmology, copyright 2019. | |
3.3.2. Treatment based on pH-responsive polymers.
The development of a polymeric carrier that can sustain the release of levofloxacin at the physiological pH range of the eye is of special importance. It is noting that levofloxacin is a popular fluoroquinolone antibiotic used for the treatment of ocular infections and is freely soluble in aqueous solutions at neutral pH.86 One strategy for developing this type of polymeric carrier is to blend sodium alginate and HPMC polymer solutions at different ratios. This results in the formation of pH-responsive polymers with gelling characteristics in response to changes in pH (from acidic to neutral/basic conditions). The levofloxacin-loaded sodium alginate/HPMC formulation was a solution at pH 4.7 but immediately transformed into a gel (at pH 7.4) when instilled onto the eyes.87 Furthermore, the formulation revealed no changes in physical appearance, pH-responsiveness, optical transparency, and drug content after exposure to autoclave sterilization. Levofloxacin was sustainably released from the alginate/HPMC gel system for 24 h while the eye drop solution was completely released after 6 h. The nonirritant nature of the pH-responsive gelling polymer was demonstrated using histopathological assessments and the lack of ocular irritation seen in the treated cornea. This treatment approach for ocular infections is seen as successful because of the effective antibacterial activity against E. coli and S. aureus, as well as minimal degradation of the alginate/HPMC blend.
Furthermore, the combination of a pH-responsive polymer with other SRP types (temperature-responsive and ion-responsive polymers) is an alternative strategy that can be used to improve SRP-DDSs. To encapsulate and release the drug, besifloxacin (which is a topical synthetic fluoroquinolone specifically developed for the topical treatment of ophthalmic infections), a combination of chitosan (temperature- and pH-responsive), polyvinyl alcohol (PVA), and gellan gum (ion-responsive) offered several advantages (such as higher gel stability and better gel strength) over the single SRPs.88,89 The results indicated that the besifloxacin-loaded chitosan/gellan gum/PVA solution was able to transform into a gel in physiological environments. Ex vivo permeation studies showed that the developed formulation enhanced drug retention at the corneal surface. The drug concentration in the aqueous humor was low when the drug was administered as a solution. In contrast, the in situ gelling formulations delivered drugs at high concentrations (105.28 ± 3.35 ng mL−1) for at least 12 h, implying that there was a sustained release profile.89
3.3.3. Treatment based on ion-responsive polymers.
Gellan gum-based in situ gelling materials are considered to be the most promising delivery systems for increasing ocular bioavailability.90,91 The formed gels are transparent and elastic, and do not irritate the eye, which allow for a continuous supply of drug molecules for extended periods without compromising patient comfort.92 Recently, ophthalmic formulations made from a combination of gellan gum and levofloxacin hemihydrate (LFH) have been utilized as ion-responsive polymers to provide sustained drug release for the treatment of bacterial conjunctivitis.27,93
The LFH-loaded gellan gum formulation exhibited a rapid in vitro gelling time (<15 s) and extended in vitro drug release (18–24 h), and was stable for at least 6 months (at 25 °C and 40% relative humidity). In vitro drug release studies implied that the LFH was maintained above a minimum inhibitory concentration (1000 ng mL−1) for 8–12 h, which signifies a remarkable increase in the prolongation of drug activity. Pharmacokinetic studies indicated that the ion-responsive polymer increased the effective therapeutic time two-fold when compared to marketed eye drops such as Levotop PF®.27 The results demonstrated a promising method for both improving LFH delivery to the eye (for the treatment of bacterial conjunctivitis) and increasing patient comfort (by reducing the need for frequent administration). Additionally, gellan gum was also exploited as an in situ gelling carrier for terbinafine hydrochloride-loaded nanoemulsion (NE), which is used for the treatment of fungal keratitis.94 The in situ NE/gellan gum gels were transparent, pseudoplastic, mucoadhesive, and had slower zero-order drug release rates when compared with the corresponding NEs. The sterilized in situ NE gel did not irritate the rabbit eyes and regulated the release rate of terbinafine hydrochloride in the rabbit aqueous humor while improving the ocular drug bioavailability. Mixtures of gellan gum and kappa-carrageenan (a seaweed polysaccharide) have been shown to be effective ion-responsive gelling materials for improving the release kinetics (by increasing drug retention 2.5×) of econazole.95
3.4. Glaucoma
Glaucoma is the second leading cause of blindness globally and is projected to affect 111.8 million people worldwide by 2040.96 In the US, approximately $748 million per year is spent treating glaucoma-related diseases for people aged 65 years and older.97 High intraocular pressure (IOP) is considered to be the primary cause of the disease, and lowering the elevated IOP is the only clinical approach that can prevent the progression of glaucoma (which leads to vision loss).96 Currently, pharmaceutical and surgical therapies are employed to reduce the elevated IOP in glaucoma, and the latter leads to high costs for the patient along with discomfort. Therefore, the preferred treatment modalities include pharmaceutical therapies applied using eye drops (such as timolol maleate, brimonidine, pilocarpine, and latanoprost). The reason is that they aid with patient compliance and are easy to use (without the need for assistance by therapists or clinicians). To overcome the low bioavailability of the eye drops, ongoing efforts have been directed towards the development of ophthalmic DDSs. These systems can prolong the pharmacological activity of drug molecules and reduce the need for frequent medical interventions, thereby minimizing side effects.
3.4.1. Treatment based on thermo-responsive polymers.
Among various thermo-responsive polymers, PNIPAAm is considered to be the most promising candidate for the development of SRP-DDSs. However, the major limitations of these polymers are their non-biodegradability and poor drug release characteristics.98 A general strategy to address these issues is chemically or physically combining PNIPAAm with a biodegradable polymer(s). An example of this is the formulation of an injectable thermo-responsive polymer, using a carbodiimide-mediated coupling reaction to graft PNIPAAM segments onto the backbone chains of gelatin, a natural biodegradable polymer that is also biocompatible.18 Pilocarpine (an antiglaucoma agent) was mixed with the PNIPAAm/gelatin copolymer solution at 25 °C, and the resultant solution was injected into the anterior chamber of glaucomatous eyes. The drug-loaded PNIPAAm/gelatin solution was rapidly transformed into a gel in the anterior chamber (whose temperature is approximately 34 °C) as a result of the thermo-responsive nature of the polymer (Fig. 7). The gradual biodegradation of gelatin within the polymer allowed for a sustained release of pilocarpine and lowered the high IOP to normal levels for 14 days (after a single dose). It is worth noting that the PNIPAAm/gelatin copolymer was synthesized by the carbodiimide-mediated coupling of PNIPAAm brushes on the aminated gelatin backbone networks. Thus, a variation in the amination degree of gelatin would result in considerable changes in the structure–property relationship of the thermo-responsive copolymer. Using adipic acid dihydrazide, the amination degree of gelatin could be optimized in response to a fixed feeding amount of PNIPAAm. This led to the formation of an SRP-DDS with an enhanced ability to sustain drug release and an improvement in glaucoma treatment efficacy (effective for 56 days after a single-dose intracameral injection).99 These results implied that the amination degree of gelatin played a pivotal role in establishing the structure–property–function relationship of the thermo-responsive copolymer, and knowledge of these relationships was critical for the development of effective SRP-DDSs for glaucoma treatment.
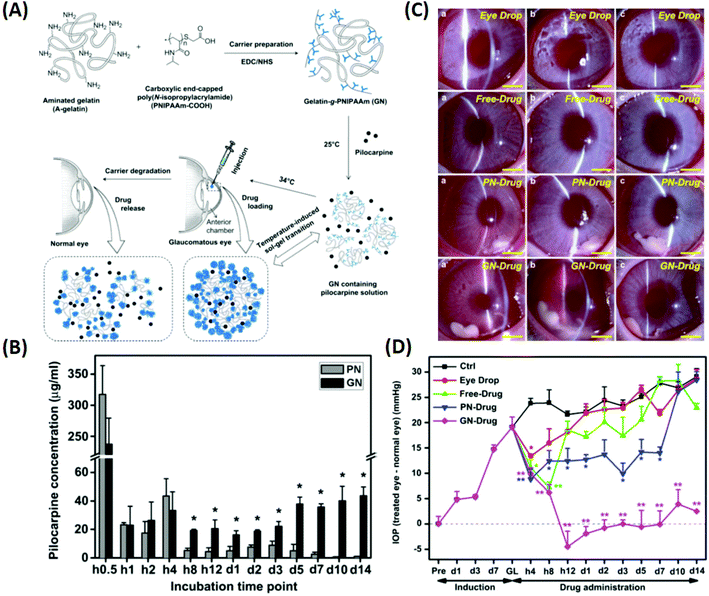 |
| Fig. 7 Illustration of the synthesis of pilocarpine-loaded PNIPAAm/gelatin and its application as an injectable, biodegradable, and thermo-responsive formulation for glaucoma treatment. (A) Schematic representation of intracameral administration of pilocarpine for glaucoma therapy using biodegradable in situ forming delivery systems composed of gelatin-g-PNIPAAm. (B) Time-course of released pilocarpine concentration. (C) Representative time-course slit-lamp biomicroscopy images of rabbit eyes in the following test groups: eye drop, free-drug, PN-drug, and GN-drug. (a) 4 h, (b) 3 days, and (c) 2 weeks after pilocarpine administration. Scale bars: 5 mm. (D) Measurements of (a) IOP after pilocarpine administration for the following test groups: eye drop, free-drug, PN-drug, and GN-drug. Glaucoma (GL) animals receiving no drug served as control groups (Ctrl). Follow-up time point: preoperation (Pre); hour (h); day (d). Reproduced from ref. 18 with permission from Elsevier, copyright 2011. | |
Another critical factor in the design of PNIPAAm-based DDSs is the selection of appropriate biodegradable polymers or suitable synthetic processes. Combining chitosan (a slowly biodegradable polymer) with PNIPAAm enhanced the biodegradation resistance of the resultant thermo-responsive copolymer, thereby prolonging the release profile of pilocarpine over 63 days.100 Free radical polymerization of NIPAAm monomers in the presence of PEG is another effective method used to formulate a thermo-responsive copolymer that can sustain the release of brimonidine. A single time administration of this formulation reduced the high IOP for 28 days which is equivalent to 56 instillations of marketed eye drops over the same period.101
Naturally derived polymers are preferred for the development of ophthalmic SRP-DDSs, probably owing to their excellent biodegradability, good biocompatibility, and recognizable moieties that support cellular activities.102 In light of these advantages, a thermo-responsive injectable gelatin/chitosan/β-glycerophosphate carrier was developed as an SRP-DDS for latanoprost to regulate high IOP in glaucoma.103 Rheological analyses implied that the gelation temperature of the latanoprost-loaded polymeric carrier was approximately 32 °C with a gel formation time of less than 1 min at 37 °C. After subconjunctival injection of the thermo-responsive polymer solution loaded with latanoprost (500 μg mL−1), the elevated IOP decreased within 8 days and remained at normal levels for the next 31 days (Table 1). Histological analysis did not reveal any signs of inflammation at the end of the follow-up period (60 days). The results suggested that the thermo-responsive gelatin/chitosan/β-glycerophosphate copolymer would have potential uses for efficient management of glaucoma. This thermo-responsive copolymer was further evaluated as a carrier for the administration of eye drops noninvasively. After topical instillation of the latanoprost-loaded thermo-responsive copolymer, the elevated IOP in glaucomatous eyes was considerably reduced within 7 days and maintained at normal levels for the subsequent 21 days.104 Alternatively, self-assembling elastin-like and silk-elastin-like polymers can be used as thermo-responsive polymers for the sustained release of antiglaucoma agents (such as timolol maleate). These formulations can be instilled in the conjunctival sac of the eye without irritation or inducing tear fluid turnover, and they cause a 2-fold increase in the IOP reduction efficiency (compared to the drug solution alone), thereby maximizing the potential utility of these SRP-DDSs.105
In addition to natural (co)polymers, synthetic copolymers such as poloxamers, PLGA–PEG–PLGA, and PEG–PLC–PEG have been intensively investigated for the development of ophthalmic SRP-DDSs. Topical instillation of a timolol maleate-loaded poloxamer allowed for the sustained release of timolol maleate, thereby improving the IOP reduction efficiency 2-fold compared to that by the administration using the drug solution alone.106 The PLGA–PEG–PLGA thermo-responsive copolymer was reported to be capable of extending the release of brimonidine for 168 h, consequently enhancing the IOP reduction efficiency 5-fold.107 Furthermore, the amphiphilic PLGA–PEG–PLGA copolymers effectively solubilized cyclosporine A in water because of the formation of polymeric micelles. Subconjunctival administration of the cyclosporine A-loaded PLGA–PEG–PLGA thermo-responsive copolymer solution inhibited scarring of the filtering bleb, maintained low IOP, and reduced complications. This demonstrated that the thermo-responsive DDS is more effective than the application of a local treatment using a solution of mitomycin C (an antifibrotic agent).108
Similarly, intracameral administration of a bevacizumab-loaded PEG–PLC–PEG thermo-responsive copolymer solution was able to increase the effective time of IOP reduction approximately 4.7×. Additionally, it allowed for the maintenance of the subconjunctival space at the appropriate size 28 days after glaucoma filtration surgery, and displayed negligible levels of fibroblast hyperplasia and new collagen tissue deposition.109 Also, Pluronic and the poly(trimethylene carbonate)/Pluronic/poly(trimethylene carbonate) polymer mixture have been utilized as thermo-responsive polymer mixtures for the long-term regulation of postoperative proliferation and maintenance of bleb filtering with minimal complications after glaucoma filtration surgery.110,111 These results demonstrate another potential use of SRP-DDSs based on thermo-responsive polymers for glaucoma surgery.
3.4.2. Treatment based on pH-responsive polymers.
The development of ophthalmic DDSs based on pH-responsive polymers is another effective approach used to treat chronic diseases such as glaucoma via the enhancement of the low drug bioavailability associated with eye drops administered to the ocular surface. Carbopol, a typical pH-responsive material, has been exploited as the key component for the ophthalmic delivery of antiglaucoma agents.112,113 To avoid eye irritation that might be caused by the acidic nature of carpobol, HPMC was added as a viscosity-enhancing agent. Although HPMC is a thermo-sensitive polymer, its LCST is approximately 50 °C; therefore, any application of this polymer at a temperature below 50 °C will not be affected by its thermo-responsive nature (ocular surface temperature is far below the LCST of HPMC). A 32 full factorial design was employed to prepare in situ gel formulations using different concentrations of carbopol and HPMC. Using this design, the optimal concentration for both polymers (carbopol and HPMC) to form as pH-responsive in situ gelling carriers for dorzolamide was found to be 0.1%.112 At this concentration, the carbopol/HPMC solution flowed freely under ambient conditions and formed a viscous gel on the ocular surface. In vitro and in vivo studies showed that this pH-responsive polymer retained dorzolamide on the eye better than the drug solution, as shown by an approximately 6-fold enhancement in IOP reduction efficiency. Additionally, this pH-responsive polymer DDS has been reported to be capable of promoting the sustained release of brimonidine tartrate.113 Experimental data demonstrated that the optimized formulation possessed gelling capability under physiological conditions and improved drug bioavailability when compared with the marketed eye drop formulation (seen from the 2-fold increase in IOP reduction efficiency). Therefore, the newly developed pH-responsive polymers are promising candidates that can be used as ophthalmic formulations to prolong the therapeutic effect of conventional eye drops. This can be credited to their capability to reduce the elevated IOP of the glaucomatous eye while reducing the number of required interventions and improving patient comfort.
3.4.3. Treatment based on ion-responsive polymers.
The most extensively investigated ion-responsive polymer is gellan gum, a natural and high molecular weight polysaccharide secreted by Pseudomonas elodea. Gellan gum has been explored to create ion-responsive ophthalmic formulations containing brinzolamide (a non-competitive, effective, and very specific carbonic anhydrase inhibitor) used for the treatment of glaucoma.114 Previous studies indicated that the optimal concentration of gellan gum was 0.25% w/v, and the solution was quickly converted into a flowing gel when mixed with simulated tear fluid, consequently allowing for the release of brinzolamide in a controlled manner. Moreover, the topical administration of the brinzolamide-loaded gellan gum onto the eye was proven to be safe. Pharmacodynamic studies suggested that the developed ion-responsive formulation effectively increased the IOP reduction efficiency 3-fold as compared to drug solution. More recently, gellan gum has been used as an ion-responsive in situ gelling agent for ophthalmic formulations consisting of brinzolamide, dimethyl sulfoxide, polyoxyl 35 castor oil, and polysorbate 80.115 The developed formulation transformed into a gel when topically instilled onto the conjunctival sac of the eye, thereby extending the residence time (up to 16–24 h). Single-dose instillations of the ion-responsive ophthalmic formulation provided the same therapeutic benefits when compared with treatment using 3–4 instillations of the commercially available product Azopt® (brinzolamide 1.0% w/v, Alcon Laboratories, USA). The tested formulations were tolerated, and they reduced the IOP from between 25 and 28 mmHg to 12 and 14 mmHg. Furthermore, a remarkable increase in the area under the change in IOP from the baseline vs. time curve and a longer mean residence time (7.4 to 17.7 h) were also achieved for the test formulations, which were longer than those of the marketed Azopt® suspension (4.9 h).115 It has also been reported that mixing gellan gum with other biopolymers such as xanthan gum and HPMC significantly enhanced the mucoadhesive force of gellan gum-based formulations while maintaining their ion-responsive properties. Formulations based on mixtures of gellan gum/xanthan gum and gellan gum/HPMC exhibited higher therapeutic efficacies and more prolonged IOP-reduction effects in comparison with marketed eye drops. Additionally, the gellan gum/xanthan gum provided superior advantages over the gellan/HPMC and was thus considered as a promising ion-responsive polymeric formulation for topical administration of Acetazolamide.116
3.5. Age-related macular degeneration
MD is a progressive chronic disease of the central retina that results in vision deficits.117 Worldwide, it affects 10% of people older than 65 years and more than 25% of people older than 75 years.118 At the late stages of the disease, vision loss usually occurs because of neovascular MD (wet type) and geographic atrophy (dry type). Currently, the standard treatment for MD is the intravitreal injection of medications capable of suppressing the vascular endothelial growth factor (VEGF). Commercial anti-VEGF drugs for the intravitreal treatment of MD are bevacizumab (Avastin), ranibizumab (Lucentis), and aflibercept (Eylea/Zaltrap). However, the efficacy of these drugs is limited by clearance action governed by intraocular fluids, leading to shorter drug half-lives on the order of 7 days.119,120 Therefore, it is highly imperative to develop ophthalmic DDSs that can sustain the delivery of anti-VEGF drugs and maintain their anti-angiogenic activity in the vitreous cavity, thereby reducing frequent intravitreal injections.
3.5.1. Treatment based on thermo-responsive polymers.
Recently, multi-block copolymers have been explored as thermo-responsive materials for the delivery of anti-VEGF drugs such as bevacizumab and aflibercept towards the treatment of MD.121 The copolymer comprises a PEG hydrophilic segment, a poly(propylene glycol) (PPG) hydrophobic segment, and a biodegradable poly(ε-caprolactone) segment (Fig. 8). To achieve the optimal in situ gelling formulation for extended anti-VEGF release, the PEG
:
PPG ratio was varied at a fixed PCL amount. In other words, the sol–gel transition temperature could be tuned by varying the PEG
:
PPG ratio, thus regulating the release of anti-VEGF drugs. The formulation with a PEG
:
PPG ratio of 4
:
1 was found to be the optimal choice for sustaining the release of bevacizumab and aflibercept up to 40 days. Therapeutic delivery of anti-VEGF drugs by the developed copolymer was evaluated using an ex vivo choroidal sprouting model, demonstrating that the gelling formulations induced a considerable reduction in the relative sprouting percentage by greater than 80% when compared with the gel alone (Fig. 8). Furthermore, a persistent rabbit retinal neovascularization model was employed to determine the therapeutic efficacy of the drug-loaded PEG–PPG–PCL thermo-responsive polymers. It showed that vascular leakage could be suppressed for at least 28 days by intravitreal injection of the thermo-responsive formulation (Fig. 8). These results suggested that the released anti-VEGF drug from the PEG–PPG–PCL thermo-responsive polymer was active and able to suppress angiogenesis in vivo for at least 28 days, displaying a potential treatment of MD. Other multi-block polymer systems such as PLGA–PEG–PLGA and PEG–PLLA–PNIPAAm have recently been employed as promising candidates for the development of novel thermo-responsive DDSs as therapeutic strategies for MD (Table 1).122,123
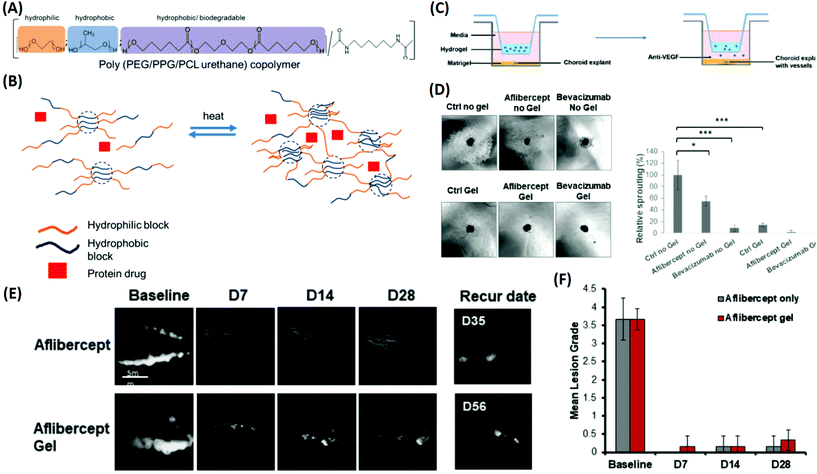 |
| Fig. 8 Development of a multi-block thermo-responsive copolymer for protein/anti-VEGF drugs and its applications for inhibiting choroidal vessel sprouting in an ex vivo model and suppressing vessel leakage in a retinal neovascularisation rabbit model. (A) Schematic of copolymer composition by functions. (B) Protein drug mixed with the copolymer; when heated, the multi-block copolymer is able to generate crosslinks and transform into a gel to encapsulate the protein drug. (C) Schematic of the choroidal sprouting experiment. Choroidal explant harvested from mice was embedded in Matrigel and cultured in medium. Thermo-responsive copolymer alone and thermo-responsive copolymer with Aflibercept and Bevacizumab were placed in trans-wells and were placed on top of explants. The presence of pores allowed free exchange between the upper chamber (containing the copolymer ± anti-VEGF) and lower chamber (containing choroidal explants). (D) Images of sprouting from choroidal explants were taken after 4 days of co-culture; quantification of percentage sprouting was performed. (E) Fluorescence angiography graph displays the leakage of fluorescein at the baseline. Treatment with Aflibercept alone and Aflibercept encapsulated in a 20% thermo-responsive copolymer suppressed vessel leakage, and did not recur for more than 28 days. (F) Clinical grading of fluorescence leak intensity by lesion grade, observed until 28 days. Reproduced from ref. 121 with permission from the Royal Society of Chemistry, copyright 2019. | |
Poly(ethylene glycol)/poly-(serinol hexamethylene urethane) (PEG/PSHU), synthesized by coupling the hydrophilic PEG block with a hydrophobic PSHU counterpart, has been reported to be capable of sustaining the release of bevacizumab in the posterior segment of the eye.124 The PEG/PSHU thermo-responsive polymer solution was effortlessly injected, well-tolerated in vivo, and non-cytotoxic. Bevacizumab was sustainably released for over 9 weeks at drug concentrations that were 4.7 times greater than those detected in the eyes receiving bolus bevacizumab injections. The increased bioavailability of bevacizumab for prolonged time periods suggested that the in situ formed gel protected the drug from degradation. Although the thermo-responsive in situ gelling formulation was administered inside the eye, the gel exerted no effects on IOP, and no histological inflammation was observed. In another study, a thermo-responsive polymer, prepared via functionalization of PEG with polyester polyol (PP), was able to provide a sustained release profile of bevacizumab for over 183 days.125 The PEG/PP thermo-responsive polymer solution did not exhibit toxic effects in human and rat cells in vitro. Also, at 1-month post-injection, no changes in IOP, and no alterations in the retinal function and structure were observed. The in situ gelling formulation inside the eye did not cause any inflammation, gliosis, or apoptosis in the retina. Therefore, these results serve as the basis for exploring the use of thermo-responsive polymers in the treatment of MD via the effective delivery of anti-VEGF drugs.
3.5.2. Treatment based on ion-responsive polymers.
Intravitreal administration is considered to be the gold standard for treating patients with MD. However, it is painful for the patient, associated with a high risk for ocular infection, and induces hemorrhage, retinal detachment, and many other complications.126 Additionally, MD is a disease that occurs in the posterior segment of the eye, which raises more obstacles for treatment using noninvasive methods. Therefore, it is necessary to develop new treatment strategies to not only prevent MD progression at an early stage but also to deliver therapeutics to target sites in the retinal region. A major risk factor that plays a pivotal role in the pathological process of MD is oxidative stress.127 To address this, developing ophthalmic formulations that can scavenge excessive oxygen species and protect the retina from oxidative stress damage is of significant importance for the treatment of MD. One example of this is the ion-responsive ophthalmic formulation, consisting of lutein (an antioxidant), stearyl penetratin (a cell-penetrating agent), a nanoemulsion (a water-soluble enhancer), and gellan gum (an in situ gelling agent).128 The developed formulations can protect retinal cells from the damage caused by hydrogen peroxide and suppress intracellular reactive oxygen species.
When topically instilled into the conjunctival sac, the formulation solution transformed immediately into the gel state, prolonging the retention time of lutein. Additionally, this gel exerted no irritation and no toxicity to the eye. With the assistance of the penetrating peptide, lutein was quickly delivered to the posterior segment of the eye and distributed in the retinal region. In a dry MD mouse model, the therapy based on topical administration of the ion-responsive formulation effectively protects the retinal structure, while suppressing oxidative stress and inhibiting cell apoptosis. This ion-responsive formulation holds great promise for the treatment of MD and other fundus diseases.
4. Beyond sustained drug release
To develop ophthalmic SRP-DDSs with improved therapeutic outcomes, it is imperative that we not only increase drug bioavailability at lesion sites but also provide additional therapeutic effects. When pharmacologically treating diseases occurring on the ocular surface, anchoring the responsive formulation onto the mucous layer of the tear (which is the biological entity of interest) is an effective approach. This allows for the extension of drug residence time at the pre-corneal region and also enhancement of drug permeation through the underlying corneal epithelium. To this end, the lectin helix pomatia agglutinin (HPA), a protein/glycoprotein of non-immunological origin which has distinct carbohydrate-binding specificities, has been covalently bound to the PNIPAAm/gelatin thermo-responsive copolymer via a carbodiimide-mediated coupling reaction.129 The role of the lectin HPA in the ternary material system is to enhance the adhesive capabilities of the thermo-responsive copolymer by mediating binding to carbohydrates on the mucus or epithelial cell surface.130 The HPA-functionalized thermo-responsive copolymer exhibited similar in situ gelling characteristics compared with the non-functionalized counterpart when placed in contact with the ocular surface. Because of highly mucoadhesive properties, the functionalized thermo-responsive gel strongly binds to the mucus layer of the tear film, which prevents the gel from draining out by the tear fluid and blinking action of the eye. In contrast, the thermo-responsive PNIPAAm/gelatin carrier without the lectin HPA showed poor mucoadhesive properties, resulting in faster drug clearance. In a rabbit model of DE, topical instillation of the HPA-functionalized PNIPAAm/gelatin carrier loaded with EGCG was demonstrated to effectively repair corneal epithelial defects. This was done by attenuating cellular inflammation, oxidative stress, and cell apoptosis for 14 days post-treatment while the drug-loaded PNIPAAm/gelatin system sustained therapeutic effects for less than 5 days.129
A favorable SRP-DDS for the treatment of ocular diseases must not only possess a sustained drug release profile but also inhibit related risk factors (such as inflammation and oxidative stress) that are not treated by the encapsulated drug. In this context, polyamidoamine (PAMAM) dendrimers have been explored in PNIPAAm/gelatin thermo-responsive carriers because they are biodegradable and regulate the inflammatory response.131 This study showed that the drug release profile and anti-inflammatory effects were strongly influenced by the generation of PAMAM dendrimers. Due to the specific structure of the third generation of the PAMAM dendrimers, such as sufficiently large interior cavities and an adequate number of amine surface groups, the synergistic effects in the PNIPAAm–gelatin copolymer can be optimized. This process endows the SRP-DDS with extended drug release profiles (greater than 80 days) and potent anti-inflammatory activities against nitric oxide, interleukin-6, phospho-p38, and prostaglandin E2. The one-time intracameral injection of the optimal thermo-responsive polymer coloaded with pilocarpine and ascorbic acid resulted in the effective mitigation of progressive glaucoma by simultaneously suppressing inflammation and stimulating the regeneration of stromal collagen and retinal laminin. In another study, the incorporation of 4-hydroxy-3,5-dimethoxybenzoic acid (an antioxidant) with a PNIPAAm/chitosan thermo-responsive copolymer endowed the responsive carrier with strong antioxidant activities without deterioration of its drug encapsulation ability or release efficiency.132 Specifically, the study demonstrated the development of an advanced SRP-DDS to treat glaucomatous optic neuropathy, which is mainly associated with three main risk factors, including ocular hypertension, oxidative stress, and neuronal degeneration (Fig. 9). The backbone chains of biodegradable chitosan are covalently bonded with PNIPAAm segments and the benzoic acid derivative molecules, and this allows for the establishment of injectable, thermo-responsive, and antioxidant carriers. Pharmacological treatment relied on a single intracameral injection of the SRP-DDS coloaded with pilocarpine and RGFP966, which remarkably alleviated the chronic disease for an extended period (greater than 70 days).
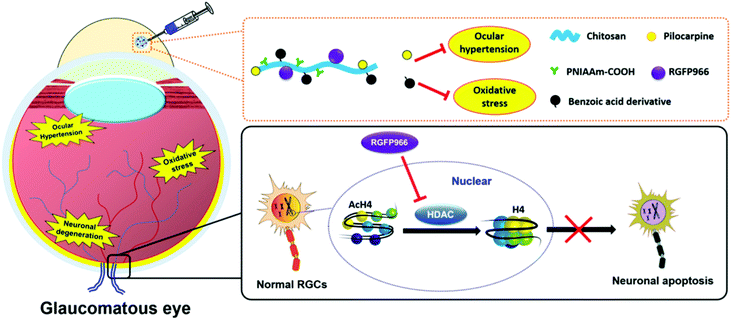 |
| Fig. 9 Schematic illustration of a pharmacological treatment of glaucomatous optic neuropathy using a benzoic acid derivative-modified PNIPAAm/chitosan copolymer as a therapeutic SRP-DDS. Reproduced from ref. 132 with permission from Elsevier, copyright 2019. | |
Noninvasive delivery of ophthalmic drugs to the interior parts of the eye for effective pharmacotherapy of intraocular diseases remains a great challenge because of the presence of ocular physiological barriers. To overcome these obstacles, the SRP-based carriers must be capable of penetrating through the cornea, delivering ophthalmic drugs to targeted intraocular tissues and lesion sites, as well as sustaining pharmacological activity. In this regard, an advanced SRP-DDS consisting of chitosan, ZM241385 (targeting agent), and hollow ceria nanoparticles (therapeutic carrier) has been rationally designed.133 For this SRP-DDS, chitosan, a pH-responsive polymer capable of opening epithelial tight junctions, played the dual role of facilitating the nanocarriers to penetrate through the cornea and sustaining the drug release.134,135 Single topical instillation of the developed formulation onto experimental glaucomatous eyes effectively mitigated disease progression for 7 days while using commercial eye drops had an effective treatment time of approximately 4 h. This significant enhancement in effective treatment time is possibly due to improved intraocular drug delivery (characterized by a 250-fold increase in drug bioavailability) and the intrinsic therapeutic activity of the nanocarriers. These findings suggest a new strategy for using SRPs to develop advanced ophthalmic formulations for the efficient management of ocular diseases that occur within the inner segments of the eye.
5. Conclusions
A large number of design strategies have been proposed to overcome the physiological barriers of the eye in order to improve drug bioavailability while reducing medical intervention times and decreasing the adverse effects. In this mini-review, recent advances in the development of stimuli-responsive polymer-based drug delivery systems for the treatment of ocular diseases are discussed. Firstly, typical stimuli-responsive polymers employed in ophthalmic formulations were presented and analyzed based on their responses to ocular environmental conditions such as temperature, pH, and ion composition. Also, basic information on the stimuli-response mechanisms of these polymers is provided. Guidance on design strategies and methodologies to develop effective ophthalmic stimuli-responsive drug delivery systems is provided, and critical factors such as stimuli response type, ocular biocompatibility, ocular biodegradability, drug encapsulation, and release, and chemical structure of polymers are discussed. The review also recommends polymers derived from both natural and synthetic sources as well as their combinations for use in the development of stimuli-responsive drug delivery systems. Importantly, applications of stimuli-responsive polymer-based drug delivery systems for the treatment of prevalent ocular diseases such as dry eye, ocular infection, glaucoma, and age-related macular degeneration were systematically analyzed and summarized (Table 1). Also highlighted is the chemical combination of the stimuli-responsive polymers with functional biomaterials to establish novel drug delivery systems with advanced functions and multiple therapeutic effects for better treatment efficacies. Our purpose in this review was to attract attention to the structure–property–function relationships of stimuli-responsive materials for innovative therapeutic strategies and thereby serve to fill the spaces between the perspectives on the chemistry and biology of polymers as well as materials engineering. This review is also expected to motivate biomedical scientists to view stimuli-responsive polymers from a materials perspective and materials researchers to consider the health and biomedical implications of designing stimuli-responsive polymers for drug delivery systems. A promising direction for future studies can be focused on structural modification/manipulation of stimuli-responsive polymers with functional biomaterials of interest. For example, to develop topical eye drops using stimuli-responsive polymers, one should consider that the mucous layer of the tear film is the first static biological entity to interact with the produced formulations. Considering the presence of glycoproteins in the mucous layer, the functional biomaterials of interest can be designed/selected to possess thiol groups, which allow the formation of disulfide bonds with cysteine-rich subdomains of the glycoproteins, consequently bestowing the stimuli-responsive polymers with high muco-adhesion performance (i.e., enhancing drug bioavailability on the pre-corneal region).136 For treating diseases occurring in the innermost parts of the eye such as macular degeneration, chemical grafting of stimuli-responsive polymers with biomaterials that can enhance the transport of designed formulations to the retina and target pharmacological sites of action (e.g., drusen) would be of immense benefit for improved pharmacotherapies. Addition of such prospective features to stimuli-responsive polymers can be achieved by proper exploitation of retinal tissue-penetrating/targeting agents such as cell penetrating peptides and protein complement component 3. However, in addition to advancing the functions of the stimuli-responsive polymers, issues involving the safety, stability, sterility, and purity of the developed ophthalmic formulations need to be thoroughly considered from early stages during the development.
Conflicts of interest
There are no conflicts to declare.
Acknowledgements
This work was supported by the grants MOST107-2221-E-182-058-MY3, MOST107-2314-B-182-016-MY3, and MOST109-2811-E-182-503 from the Ministry of Science and Technology of Republic of China.
References
- R. R. A. Bourne, S. R. Flaxman, T. Braithwaite, M. V. Cicinelli, A. Das, J. B. Jonas, J. Keeffe, J. H. Kempen, J. Leasher, H. Limburg, K. Naidoo, K. Pesudovs, S. Resnikoff, A. Silvester, G. A. Stevens, N. Tahhan, T. Y. Wong and H. R. Taylor, Lancet Global Health, 2017, 5, e888–e897 CrossRef.
- F. J. Cabrera, D. C. Wang, K. Reddy, G. Acharya and C. S. Shin, Drug Discovery Today, 2019, 24, 1679–1684 CrossRef CAS.
- D. Huang, Y. S. Chen and I. D. Rupenthal, Adv. Drug Delivery Rev., 2018, 126, 96–112 CrossRef CAS.
- J. J. Kang-Mieler, K. M. Rudeen, W. Liu and W. F. Mieler, Eye, 2020, 34, 1371–1379 CrossRef CAS.
- X. Zhao, J. Si, D. Huang, K. Li, Y. Xin and M. Sui, J. Controlled Release, 2020, 323, 565–577 CrossRef CAS.
- Z. Ni, H. Yu, L. Wang, D. Shen, T. Elshaarani, S. Fahad, A. Khan, F. Haq and L. Teng, J. Mater. Chem. B, 2020, 8, 1555–1575 RSC.
- Q. A. Besford, F. Cavalieri and F. Caruso, Adv. Mater., 2020, 32, 1904625 CrossRef CAS.
- J. F. Quinn, M. R. Whittakera and T. P. Davis, Polym. Chem., 2017, 8, 97–126 RSC.
- M. Wei, Y. Gao, X. Lia and M. J. Serpe, Polym. Chem., 2017, 8, 127–143 RSC.
- K. Wang and Z. Han, J. Controlled Release, 2017, 268, 212–224 CrossRef CAS.
- D. Lin, L. Lei, S. Shi and X. Li, Macromol. Biosci., 2019, 19, 1900001 CrossRef.
- C. R. Lynch, P. P. D. Kondiah, Y. E. Choonara, L. C. du Toit, N. Ally and V. Pillay, Front. Bioeng. Biotechnol., 2020, 8, 228 CrossRef.
- C. H. Tsai, P. Y. Wang, I. C. Lin, H. Huang, G. S. Liu and C. L. Tseng, Int. J. Mol. Sci., 2018, 19, 2830 CrossRef.
- A. A. Al-Kinani, G. Zidan, N. Elsaid, A. Seyfoddin, A. W. G. Alani and R. G. Alany, Adv. Drug Delivery Rev., 2018, 126, 113–126 CrossRef CAS.
- D. Shastri, L. Patel and R. Parikh, J. Young Pharm., 2010, 2, 116–120 CrossRef CAS.
- S. Merino, C. Martín, K. Kostarelos, M. Prato and E. Vázquez, ACS Nano, 2015, 9, 4686–4697 CrossRef CAS.
- D. C. Ferreira Soares, C. M. R. Oda, L. O. F. Monteiro, A. L. B. de Barros and M. L. Tebaldi, J. Drug Targeting, 2019, 27, 355–366 CrossRef CAS.
- J. Y. Lai and A. C. Hsieh, Biomaterials, 2012, 33, 2372–2387 CrossRef CAS.
- H. J. Kim, K. Zhang, L. Moore and D. Ho, ACS Nano, 2014, 8, 2998–3005 CrossRef CAS.
- K. A. Soliman, K. Ullah, A. Shah, D. S. Jones and T. R. R. Singh, Drug Discovery Today, 2019, 24, 1575–1586 CrossRef CAS.
- J. J. Giner-Casares, M. Henriksen-Lacey, M. Coronado-Puchau and L. M. Liz-Marzan, Mater. Today, 2016, 19, 19–28 CrossRef CAS.
- P. Thoniyot, M. J. Tan, A. A. Karim, D. J. Young and X. J. Loh, Adv. Sci., 2015, 2, 1400010 CrossRef.
- W. H. De Jong and P. J. Borm, Int. J. Nanomed., 2008, 3, 133–149 CrossRef CAS.
- M. Kanapathipillai, A. Brock and D. E. Ingber, Adv. Drug Delivery Rev., 2014, 79–80, 107–118 CrossRef CAS.
- A. Patel, K. Cholkar, V. Agrahari and A. K. Mitra, World J. Pharmacol., 2013, 2, 47–64 CrossRef.
- C. Jumelle, S. Gholizadeh, N. Annabi and R. Dana, J. Controlled Release, 2020, 321, 1–22 CrossRef CAS.
- H. Bhalerao, K. B. Koteshwara and S. Chandran, AAPS PharmSciTech, 2019, 20, 272 CrossRef.
- M. Sponchioni, U. Capasso Palmiero and D. Moscatelli, Mater. Sci. Eng., C, 2019, 102, 589–605 CrossRef CAS.
- J. J. Maller, S. S. George, R. P. Viswanathan, P. B. Fitzgerald and P. Junor, J. Biomed. Opt., 2016, 21, 26001 CrossRef.
- A. Choi, K. D. Seo, H. Yoon, S. J. Han and D. S. Kim, Biomater. Sci., 2019, 7, 2277–2287 RSC.
- R. Singh, S. A. Deshmukh, G. Kamath, S. K. R. S. Sankaranarayanan and G. Balasubramanian, Comput. Mater. Sci., 2017, 126, 191–203 CrossRef CAS.
- A. Chenite, C. Chaput, D. Wang, C. Combes, M. D. Buschmann, C. D. Hoemann, J. C. Leroux, B. L. Atkinson, F. Binette and A. Selmani, Biomaterials, 2000, 21, 2155–2161 CrossRef CAS.
- S. Supper, N. Anton, N. Seidel, M. Riemenschnitter, C. Schoch and T. Vandamme, Langmuir, 2013, 29, 10229–10237 CrossRef CAS.
- H. Huang, X. Qi, Y. Chen and Z. Wu, Saudi Pharm. J., 2019, 27, 990–999 CrossRef CAS.
- E. Russo and C. Villa, Pharmaceutics, 2019, 11, 671 CrossRef CAS.
- U. D. Laddha and H. S. Mahajan, Int. J. Adv. Pharm., 2017, 6, 31–40 CAS.
- H. Almeida, M. H. Amaral, P. Lobao and J. M. S. Lobo, Expert Opin. Drug Delivery, 2013, 10, 1223–1237 CrossRef CAS.
- Y. Wu, Y. Liu, X. Li, D. Kebebe, B. Zhang, J. Ren, J. Lu, J. Li, S. Du and Z. Liu, Asian J. Pharm. Sci., 2019, 14, 1–15 CrossRef.
- P. Wang, W. Chu, X. Zhuo, Y. Zhang, J. Gou, T. Ren, H. He, T. Yin and X. Tang, J. Mater. Chem. B, 2017, 5, 1551–1565 RSC.
- P. A. Faccia, F. M. Pardinia and J. I. Amalvy, J. Drug Delivery Sci. Technol., 2019, 51, 45–54 CrossRef CAS.
- P. Gupta, K. Vermani and S. Garg, Drug Discovery Today, 2002, 7, 569–579 CrossRef CAS.
- G. Rajoria and A. Gupta, Am. J. PharmTech Res., 2012, 2, 24–53 Search PubMed.
- A. Dubey and P. Prabhu, Int. J. Pharm. Invest., 2014, 4, 112–118 CrossRef CAS.
- I. D. Rupenthal, C. R. Green and R. G. Alany, Int. J. Pharm., 2011, 411, 78–85 CrossRef CAS.
- A. Almeida, M. H. Amaral, P. Lobao and J. M. Lobo, Drug Discovery Today, 2014, 19, 400–412 CrossRef.
- K. Reed, A. Li, B. Wilson and T. Assamoi, J. Ocul. Pharmacol. Ther., 2016, 32, 574–582 CrossRef CAS.
- S. K. Kushwaha, P. Saxena and A. Rai, Int. J. Pharm. Invest., 2012, 2, 54–60 CrossRef CAS.
- F. García-Ochoa, V. E. Santos, J. A. Casas and E. Gómez, Biotechnol. Adv., 2000, 18, 549–579 CrossRef.
- K. Hosoya, M. Tomi and M. Tachikawa, Expert Opin. Drug Delivery, 2011, 8, 1571–1587 CrossRef CAS.
- S. Awwad, A. H. A. Mohamed Ahmed, G. Sharma, J. S. Heng, P. T. Khaw, S. Brocchini and A. Lockwood, Br. J. Pharmacol., 2017, 174, 4205–4223 CrossRef CAS.
- L. D. Barber, S. C. Pflugfelder, J. Tauber and G. N. Foulks, Ophthalmology, 2005, 112, 1790–1794 CrossRef.
- R. Gaudana, H. K. Ananthula, A. Parenky and A. K. Mitra, AAPS J., 2010, 12, 348–360 CrossRef CAS.
- A. Bochot and E. Fattal, J. Controlled Release, 2012, 161, 628–634 CrossRef CAS.
- T. J. Shah, M. D. Conway and G. A. Peyman, Clin. Ophthalmol., 2018, 12, 2223–2235 CrossRef CAS.
- D. Ghate, W. Brooks, B. E. McCarey and H. F. Edelhauser, Invest. Ophthalmol. Visual Sci., 2007, 48, 2230–2237 CrossRef.
- J. A. Clayton, N. Engl. J. Med., 2018, 378, 2212–2223 CrossRef CAS.
- J. Yu, C. V. Asche and C. J. Fairchild, Cornea, 2011, 30, 379–387 CrossRef.
- A. Guzman-Aranguez, B. Fonseca, G. Carracedo, A. Martin-Gil, A. Martinez-Aguila and J. Pintor, Eye Contact Lens, 2016, 42, 280–288 CrossRef.
- Z. A. Karcioglu and J. C. Fleming, Am. J. Ophthalmol., 2008, 145, 586–587 CrossRef.
- J. Dhandhukia, I. Weitzhandler, W. Wang and J. A. MacKay, Biomacromolecules, 2013, 14, 976–985 CrossRef CAS.
- W. Wang, A. Jashnani, S. R. Aluri, J. A. Gustafson, P. Y. Hsueh, F. Yarber, R. L. McKown, G. W. Laurie, S. F. Hamm-Alvarez and J. A. MacKay, J. Controlled Release, 2015, 199, 156–167 CrossRef CAS.
- E. L. C. Cruz-Cazarim, M. S. Cazarim, A. T. Ogunjimi, R. Petrilli, E. M. Rocha and R. F. V. Lopez, Eur. J. Pharm. Biopharm., 2019, 140, 1–10 CrossRef CAS.
- L. J. Luo and J. Y. Lai, Sci. Rep., 2017, 7, 9380 CrossRef.
- J. W. Yoo, N. Giri and C. H. Lee, Int. J. Pharm., 2011, 403, 262–267 CrossRef CAS.
- F. A. Maulvi, H. H. Choksi, A. R. Desai, A. S. Patel, K. M. Ranch, B. A. Vyas and D. O. Shah, Colloids Surf., B, 2017, 157, 72–82 CrossRef CAS.
- S. P. Phadatare, M. Momin, P. Nighojkar, S. Askarkar and K. K. Singh, Adv. Pharm., 2015, 2015, 704946 Search PubMed.
- S. Pflugfelder, G. Geerling, S. Kinoshita, M. A. Lemp, J. McCulley, D. Nelson, G. N. Novack, J. Shimazaki and C. Wilson, Ocul. Surf., 2007, 5, 163–178 CrossRef.
- D. Acar, I. T. Molina-Martínez, M. Gómez-Ballesteros, M. Guzmán-Navarro, J. M. Benítez-Del-Castillo and R. Herrero-Vanrell, Contact Lens Anterior Eye, 2018, 41, 93–96 CrossRef.
- M. Teweldemedhin, H. Gebreyesus, A. H. Atsbaha, S. W. Asgedom and M. Saravanan, BMC Ophthalmol., 2017, 17, 212 CrossRef.
- Y. Shimizu, H. Toshida, R. Honda, A. Matsui, T. Ohta, Y. Asada and A. Murakami, Clin. Ophthalmol., 2013, 7, 695–702 Search PubMed.
- R. P. Kowalski and D. K. Dhaliwal, Expert Rev. Anti-Infect. Ther., 2005, 3, 131–139 CrossRef CAS.
- M. Daniell, R. Mills and N. Morlet, Br. J. Ophthalmol., 2003, 87, 1167 CrossRef CAS.
- T. P. O'Brien, Eye, 2003, 17, 957–974 CrossRef.
- S. Mohammed, G. Chouhan, O. Anuforom, M. Cooke, A. Walsh, P. Morgan-Warren, M. Jenkins and F. de Cogan, Mater. Sci. Eng., C, 2017, 78, 203–209 CrossRef CAS.
- H. O. Ammar, H. A. Salama, M. Ghorab and A. A. Mahmoud, Drug Dev. Ind. Pharm., 2010, 36, 1330–1339 CrossRef CAS.
- R. Barse, C. Kokare and A. Tagalpallewar, J. Drug Delivery Sci. Technol., 2016, 33, 66–74 CrossRef CAS.
- N. Tavakoli, S. Taymouri, A. Saeidi and V. Akbari, Pharm. Dev. Technol., 2019, 24, 891–901 CrossRef CAS.
- M. A. Ward and T. K. Georgiou, Polymers, 2011, 3, 1215–1242 CrossRef CAS.
- M. Mora-Pereira, E. M. Abarca, S. Duran, W. Ravis, R. J. McMullen Jr., B. M. Fischer, Y. H. P. Lee and A. A. Wooldridge, BMC Vet. Res., 2020, 16, 115 CrossRef CAS.
- S. Khan, S. Warade and D. J. Singhavi, J. Ocul. Pharmacol. Ther., 2018, 34, 287–297 CrossRef CAS.
- S. Deepthi and J. Jose, Int. Ophthalmol., 2019, 39, 1355–1366 CrossRef CAS.
- Y. H. Cheng, Y. F. Chang, Y. C. Ko and C. J. Liu, J. Biomed. Mater. Res., Part B, 2020, 108, 8–13 CrossRef CAS.
- S. Sapino, E. Peira, D. Chirio, G. Chindamo, S. Guglielmo, S. Oliaro-Bosso, R. Barbero, C. Vercelli, G. Re, V. Brunella, C. Riedo, A. M. Fea and M. Gallarate, Nanomaterials, 2019, 9, 1461 CrossRef CAS.
- E. Dosmar, W. Liu, G. Patel, A. Rogozinski, W. F. Mieler and J. J. Kang-Mieler, Transl. Vision Sci. Technol., 2019, 8, 53 CrossRef.
- S. Lefèvre, M. Saleh, L. Marcellin, A. Subilia, T. Bourcier, G. Prévost and F. Jehl, Antimicrob. Agents Chemother., 2012, 56, 2485–2492 CrossRef.
- G. Li, L. Xu, M. Jiang and X. Wu, Drug Dev. Ind. Pharm., 2020, 46, 673–681 CrossRef CAS.
- P. Jain, C. P. Jaiswal, M. A. Mirza, M. K. Anwer and Z. Iqbal, Drug Dev. Ind. Pharm., 2020, 46, 50–56 CrossRef CAS.
- M. H. Chang and H. B. Fung, Clin. Ther., 2010, 32, 454–471 CrossRef CAS.
- S. S. Imam, S. N. A. Bukhari and A. Ali, Artif. Cells, Nanomed., Biotechnol., 2018, 46, 959–967 CrossRef CAS.
- H. M. El-Laithy, D. I. Nesseem, A. A. El-Adly and M. Shoukry, Arch. Pharmacal Res., 2011, 34, 1663–1678 CrossRef CAS.
- K. Y. Janga, A. Tatke, N. Dudhipala, S. P. Balguri, M. M. Ibrahim, D. N. Maria, M. M. Jablonski and S. Majumdar, J. Pharmacol. Exp. Ther., 2019, 370, 814–822 CrossRef CAS.
- U. Kotreka, V. Davis and M. Adeyeye, PLoS One, 2017, 12, 1–19 CrossRef.
- O. Saher, D. M. Ghorab and N. M. Mursi, Pharm. Dev. Technol., 2016, 21, 600–610 CrossRef CAS.
- S. A. Tayel, M. A. El-Nabarawi, M. I. Tadros and W. H. Abd-Elsalam, Int. J. Pharm., 2013, 443, 293–305 CrossRef CAS.
- V. Díaz-Tomé, A. Luaces-Rodríguez, J. Silva-Rodríguez, S. Blanco-Dorado, L. García-Quintanilla, J. Llovo-Taboada, J. Blanco-Méndez, X. García-Otero, R. Varela-Fernández, M. Herranz, M. Gil-Martínez, M. Jesús Lamas, M. González-Barcia, F. J. Otero-Espinar and A. Fernández-Ferreiro, J. Pharm. Sci., 2018, 107, 1342–1351 CrossRef.
- Y. C. Tham, X. Li, T. Y. Wong, H. A. Quigley, T. Aung and C. Y. Cheng, Ophthalmology, 2014, 121, 2081–2090 CrossRef.
- W. S. Lambert, B. J. Carlson, A. E. van der Ende, G. Shih, J. N. Dobish, D. J. Calkins and E. Harth, Transl. Vision Sci. Technol., 2015, 4, 1 CrossRef.
- F. Dong, I. Firkowska-Boden, M. M. L. Arras and K. D. Jandt, RSC Adv., 2017, 7, 3720–3726 RSC.
- L. J. Luo and J. Y. Lai, Mater. Sci. Eng., C, 2019, 98, 897–909 CrossRef CAS.
- L. J. Luo, C. C. Huang, H. C. Chen, J. Y. Lai and M. Matsusaki, Carbohydr. Polym., 2018, 197, 375–384 CrossRef CAS.
- M. V. Fedorchak, I. P. Conner, J. S. Schuman, A. Cugini and S. R. Little, Sci. Rep., 2017, 7, 8639 CrossRef.
- S. Chatterjee, P. C. Hui and C. W. Kan, Polymers, 2018, 10, 480 CrossRef.
- Y. H. Cheng, K. H. Hung, T. H. Tsai, C. J. Lee, R. Y. Ku, A. W. H. Chiu, S. H. Chiou and C. J. L. Liu, Acta Biomater., 2014, 10, 4360–4366 CrossRef CAS.
- Y. H. Cheng, T. H. Tsai, Y. Y. Jhan, A. W. H. Chiu, K. L. Tsai, C. S. Chien, S. H. Chiou and C. J. L. Liu, Carbohydr. Polym., 2016, 144, 390–399 CrossRef CAS.
- A. Fernández-Colino, D. A. Quinteros, D. A. Allemandi, A. Girotti, S. D. Palma and F. J. Arias, Mol. Pharm., 2017, 14, 4498–4508 CrossRef.
- Y. Zeng, J. Chen, Y. Li, J. Huang, Z. Huang, Y. Huang, X. Pan and C. Wu, Life Sci., 2018, 212, 80–86 CrossRef CAS.
- J. Sun, Y. Lei, Z. Dai, X. Liu, T. Huang, J. Wu, Z. P. Xu and X. Sun, ACS Appl. Mater. Interfaces, 2017, 9, 7990–7999 CrossRef CAS.
- J. Sun, X. Liu, Y. Lei, M. Tang, Z. Dai, X. Yang, X. Yu, L. Yu, X. Sun and J. Ding, J. Mater. Chem. B, 2017, 5, 6400–6411 RSC.
- Q. Han, Y. Wang, X. Li, R. Peng, A. Li, Z. Qian and L. Yu, J. Mater. Sci. Mater. Med., 2015, 26, 225 CrossRef.
- V. Sutariya, N. Miladore, W. Geldenhuys, D. Bhatia, D. Wehrung and H. Nakamura, Pharm. Dev. Technol., 2013, 18, 957–962 CrossRef CAS.
- L. Xi, T. Wang, F. Zhao, Q. Zheng, X. Li, J. Luo, J. Liu, D. Quan and J. Ge, PLoS One, 2014, 9, e100632 CrossRef.
- M. Kouchak, M. Mahmoodzadeh and F. Farrahi, AAPS PharmSciTech, 2019, 20, 210 CrossRef.
- R. K. Barse, A. A. Tagalpallewar, C. R. Kokare, J. P. Sharma and P. K. Sharma, Drug Dev. Ind. Pharm., 2018, 44, 800–807 CrossRef CAS.
- J. Sun and Z. Zhou, Drug Des., Dev. Ther., 2018, 12, 383–389 CrossRef CAS.
- H. Bhalerao, K. B. Koteshwara and S. Chandran, AAPS PharmSciTech, 2020, 21, 69 CrossRef.
- N. Morsi, M. Ibrahim, H. Refai and H. El Sorogy, Eur. J. Pharm. Sci., 2017, 104, 302–314 CrossRef CAS.
- L. S. Lim, P. Mitchell, J. M. Seddon, F. G. Holz and T. Y. Wong, Lancet, 2012, 379, 1728–1738 CrossRef.
- W. M. Al-Zamil and S. A. Yassin, Clin. Interventions Aging, 2017, 12, 1313–1330 CrossRef CAS.
- S. J. Bakri, M. R. Snyder, J. M. Reid, J. S. Pulido, M. K. Ezzat and R. J. Singh, Ophthalmology, 2007, 114, 2179–2182 CrossRef.
- T. U. Krohne, N. Eter, F. G. Holz and C. H. Meyer, Am. J. Ophthalmol., 2008, 146, 508–512 CrossRef CAS.
- K. Xue, X. Zhao, Z. Zhang, B. Qiu, Q. S. W. Tan, K. H. Ong, Z. Liu, B. H. Parikh, V. A. Barathi, W. Yu, X. Wang, G. Lingam, W. Hunziker, X. Su and X. J. Loh, Biomater. Sci., 2019, 7, 4603–4614 RSC.
- A. Hirani, A. Grover, Y. W. Lee, Y. Pathak and V. Sutariya, Pharm. Dev. Technol., 2016, 21, 61–67 CrossRef CAS.
- W. Liu, B. S. Lee, W. F. Mieler and J. J. Kang-Mieler, Curr. Eye Res., 2019, 44, 264–274 CrossRef CAS.
- B. M. Rauck, T. R. Friberg, C. A. M. Mendez, D. Park, V. Shah, R. A. Bilonick and Y. Wang, Invest. Ophthalmol. Visual Sci., 2014, 55, 469–476 CrossRef CAS.
- A. Balachandra, E. C. Chan, J. P. Paul, S. Ng, V. Chrysostomou, S. Ngo, R. Mayadunne and P. van Wijngaarden, Drug Delivery, 2019, 26, 343–353 CrossRef CAS.
- R. N. Mitra, R. Gao, M. Zheng, M. J. Wu, M. A. Voinov, A. I. Smirnov, T. I. Smirnova, K. Wang, S. Chavala and Z. Han, ACS Nano, 2017, 11, 4669–4685 CrossRef CAS.
- X. Gu, S. G. Meer, M. Miyagi, M. E. Rayborn, J. G. Hollyfield, J. W. Crabb and R. G. Salomon, J. Biol. Chem., 2003, 278, 42027–42035 CrossRef CAS.
- Y. Ge, A. Zhang, R. Sun, J. Xu, T. Yin, H. He, J. Gou, J. Kong, Y. Zhang and X. Tang, Expert Opin. Drug Delivery, 2020, 17, 603–619 CrossRef CAS.
- L. J. Luo, D. D. Nguyen and J. Y. Lai, Mater. Sci. Eng., C, 2020, 115, 111095 CrossRef CAS.
- C. M. Lehr, J. Controlled Release, 2000, 65, 19–29 CrossRef CAS.
- D. D. Nguyen, L. J. Luo and J. Y. Lai, Adv. Healthcare Mater., 2019, 8, 1900702 CrossRef CAS.
- L. J. Luo, D. D. Nguyen and J. Y. Lai, J. Controlled Release, 2020, 317, 246–258 CrossRef CAS.
- L. J. Luo, D. D. Nguyen and J. Y. Lai, Biomaterials, 2020, 243, 119961 CrossRef CAS.
- T. H. Yeh, L. W. Hsu, M. T. Tseng, P. L. Lee, K. Sonjae, Y. C. Ho and H. W. Sung, Biomaterials, 2011, 32, 6164–6173 CrossRef CAS.
- J. Jiao, X. Li, S. Zhang, J. Liu, D. Di, Y. Zhang, Q. Zhao and S. Wang, Mater. Sci. Eng., C, 2016, 67, 26–33 CrossRef CAS.
- J. Y. Lai, L. J. Luo and D. D. Nguyen, Chem. Eng. J., 2020, 402, 126190 CrossRef CAS.
|
This journal is © The Royal Society of Chemistry 2020 |