Preparation of luminescent chemosensors by post-functionalization of vesicle surfaces†
Received
1st November 2014
, Accepted 26th November 2014
First published on 27th November 2014
Abstract
Surface-reactive luminescent vesicles were prepared by self-assembly of phospholipids, amphiphilic maleimides and fluorophors in aqueous solution. Those preformed liposomes were functionalized with various thiolated receptor units using a thiol-click reaction. As recognition elements, a bis-Zn2+-cyclen derivative for the detection of phosphate moieties or a DNA aptamer for the specific binding of the antibiotic ampicillin were utilized. A FRET-based assay revealed the close spatial proximity of the membrane-embedded dansyl molecules with the subsequently immobilized thiols, which is the origin for the signaling mechanism of the obtained vesicular sensors. Those receptor-functionalized liposomes indicate the binding of the targets to their surface by changes of the fluorescence emission properties of the membrane co-embedded carboxyfluorescein dyes. The post-functionalization concept can also be used for molecular imprinting on vesicle surfaces. The template-guided patterning of receptors based on bis-Zn2+-cyclen resulted in fluorescent sensors suitable for the specific recognition of a bivalent peptide.
1. Introduction
Molecular recognition at biomembranes is of fundamental importance for biological processes like cell recognition, adhesion, fusion or membrane transport phenomena. Since phospholipid vesicles can be considered as simple cell mimics, non-covalent interactions at the surface of receptor-functionalized synthetic liposomes resemble recognition events at membranes as they appear in nature.1 Hence, the knowledge obtained from the investigation of artificial vesicular systems can help to gain improved insights into the correspondent processes in biology. Conversely, a better understanding of the natural prototypes can be exploited for the development of powerful devices for analytical applications. Various strategies have been established to transform the binding event at the vesicle membrane into an observable signal.2 Fluorescent assays provide high sensitivities allowing the detection of analytes present in trace amounts, whereas colorimetric formats enable the analytic readout by the naked eye.3 Typically, the signaling unit is in direct contact with the recognition element by either covalent attachment4,5 or by non-covalent coordination as in indicator-displacement assays.4,6 However, membranes are highly dynamic due to their supramolecular composition.1 Based on this, our group recently reported on a novel strategy for the modular construction of chemosensors by means of unilamellar vesicular membranes, which serve as self-assembled supporting matrix for amphiphilic metal complex receptors and fluorescent reporter dyes.7 Due to lateral phase separation, the co-embedded amphiphilic fluorophors and binding sites are expected to cluster on the surface resulting in fluorescence quenching of the amphiphilic dyes (Fig. 1). Binding of analyte molecules to the surface-accessible receptors alters the physico-chemical properties in their microenvironment and thus induces a reorganization of the membrane components. These processes influence the optical properties of the co-embedded dyes, which in turn respond with a change of their emission intensity.
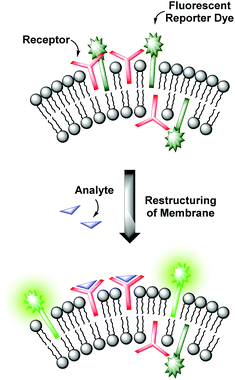 |
| Fig. 1 Sensing mechanism of receptor-functionalized fluorescent vesicles. | |
This co-embedding strategy allows an effortless preparation of sensor devices by simple mixing of different functional amphiphiles in aqueous solution. By careful variation of the binding and signaling units, their ratios and concentrations on the surface and by the choice of suitable phospholipids determining the physical properties of the membrane, vesicular sensors with specific characteristics can be designed. However, as the synthesis of the amphiphilic receptors can be quite tedious, the availability of suitable binding sites and hence the variety of addressable analytes is limited. Moreover, control over the two-dimensional receptor assembly for multipoint interactions on the surface is still a challenge.8 Its accomplishment is an essential requirement for the highly selective recognition of multivalent analytes. In order to approach these issues, we developed a more universal method, which allows the facile preparation of vesicular chemosensors in a modular fashion by means of surface “post-functionalization” via click chemistry. The surface modification of preformed liposomes is an established method for the conjugation of various ligands ranging from sugars to peptides, proteins and antibodies and has found applications in, e.g., the investigation of drug delivery systems.9 We now combine this concept of vesicle post-modification with our co-embedding strategy of functional amphiphiles into phospholipid membranes. Only groups of the vesicle membranes which are exposed to the outer side are functionalized. The resulting asymmetric membrane composition represents a much better mimic of the bilayers appearing in nature. In view of more complex receptors of biological origin, we utilized a thiol–ene click reaction10 due to the frequent occurrence of thiol groups in biomolecules. The reaction is fully biocompatible and does not, in contrast to the copper(I)-catalyzed azide–alkyne cycloaddition,11 require a metal catalyst.
2. Results and discussion
We first tested the applicability of the UV light-induced radical addition of thiols to electron-rich alkene moieties.12 This type of reaction would implicate the possibility to externally trigger the functionalization process by controlled exposure to UV light. However, the envisaged photoreaction is at micromolar concentrations in aqueous solution not efficient. Thus, we focused on the widely used Michael addition of thiols to maleimides.13 This reaction performs well over a large concentration range in aqueous media under benign conditions without the formation of byproducts and is commonly applied in bioconjugation.10 We recently employed this method for the construction of an aptamer-based biosensor by attachment of thiol-modified aptamers to membrane-embedded amphiphilic maleimide Mal-C16 (Fig. 2).14
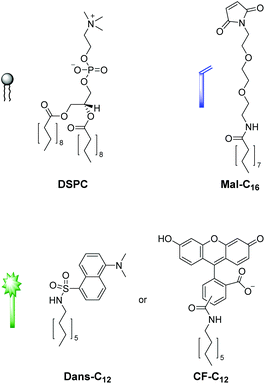 |
| Fig. 2 Structures of the functional amphiphiles used for vesicle preparation. | |
Here, we report on the development of the post-functionalization strategy of luminescent vesicle surfaces with receptor sites and demonstrate its facile and versatile applicability for the preparation of various sensors. As signaling units, either amphiphilic dansyl dye Dans-C12
15 or carboxyfluorescein CF-C12 were incorporated into membranes of small unilamellar DSPC vesicles. Fig. 3 depicts the different thiols which were used for our investigations. The syntheses of compounds Mal-C16
14 and CF-C12
7a were previously reported by us, the preparation of Cys-Trp and Zn-Cyclen2 is described in the ESI.†
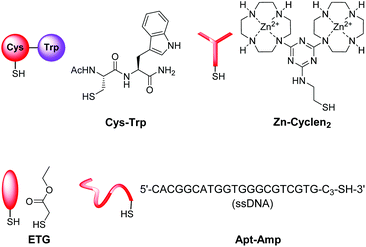 |
| Fig. 3 Thiols used for the post-functionalization of vesicle surfaces. | |
2.1 Optimization of the post-functionalization of preformed vesicles
The sensing mechanism of our vesicular sensors is based on the close spatial proximity of the binding sites to the membrane-embedded dyes. To test whether the subsequently immobilized receptor molecules fulfill this condition, we developed an assay based on the distance-dependent Förster resonance energy transfer (FRET, Fig. 4). As simple model system, we synthesized dipeptide Cys-Trp (Fig. 3). The FRET partner of tryptophan, the amphiphilic dansyl dye Dans-C12 (Förster distance: 2.1 nm),16 was co-embedded (5.0 mol%) into the membrane of DSPC vesicles together with the attachment site for thiols Mal-C16 (5.0 mol%). The preparation of the vesicles was performed in 25 mM HEPES buffer at a pH of 7.4 according to previously reported procedures (see ESI† for details). Extrusion through 100 nm polycarbonate membranes yielded vesicles V-Dans with a size distribution of around 130–150 nm (see Fig. S1, ESI†). The non-functionalized vesicles were stable and did not show significant aggregation after one day (see Fig. S2, ESI†).
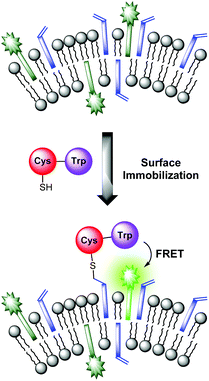 |
| Fig. 4 FRET assay proving the close spatial proximity of immobilized thiols to the membrane-embedded fluorophors. | |
Addition of Cys-Trp to V-Dans showed a time-dependent decrease of the tryptophan emission evidencing the fluorescence energy transfer and a considerable increase of the dansyl signal (Fig. 5).
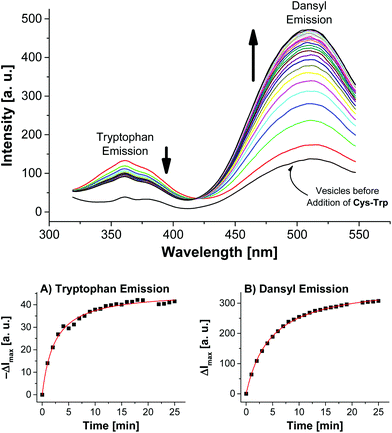 |
| Fig. 5 Top: Time-dependent fluorescence spectra of vesicles V-Dans after addition of 3.0 mol% of peptide Cys-Trp (λex = 280 nm). Bottom: course of surface reaction as evidenced by the emission signals of either the tryptophan or dansyl dye. | |
Apart from the contribution of the resonance energy transfer from the tryptophan moiety, the extraordinarily strong increase of the dansyl signal must be attributed to two additional independent effects: (i) It is well documented that the fluorescence emission of dansyl dyes is quenched by adjacent maleimides via photoinduced electron transfer (PET), which proceeds from the excited state of the fluorophor to the maleimide.17 After conjugation of thiols to the electron-deficient double bond of the maleimide π-system of Mal-C16, this PET process is interrupted resulting in a dansyl fluorescence quantum yield enhancement. (ii) Furthermore, by the altered physico-chemical properties of the local environment upon surface functionalization, the optical properties of the membrane-embedded fluorophors change causing an increase in emission intensity. A fluorescence increase of V-Dans during the surface reaction with a non-tryptophan-containing thiol like ETG (ethyl thioglycolate, Fig. 3) confirms this interpretation (see Fig. S3, ESI†). The observations clearly show that the thiol is covalently attached to the membrane-embedded maleimide Mal-C16 and the membrane-bound thiols in turn are in close proximity to the co-embedded fluorophors. Membranes without Mal-C16 show a much higher initial dansyl emission intensity and no time-dependent change of the emission signal of either fluorophors, the tryptophan or dansyl dye, occurred after addition of Cys-Trp (see Fig. S5, ESI†). The same observation was made for vesicles V-Dans whose surface-accessible maleimides were saturated with ETG prior to the addition of Cys-Trp (see Fig. S4, ESI†). The experiments demonstrate that non-functionalized, maleimide-containing vesicles V-Dans can also be regarded as simple self-assembled sensors for thiols.18
In contrast to dansyl dyes, carboxyfluorescein derivatives do not show PET processes in presence of maleimides in the membrane. To further study the effect (ii), we prepared vesicles V-CF with embedded carboxyfluorescein CF-C12, which showed a time-dependent increase of the fluorescence signal after addition of thiol ETG indicating membrane restructuring processes upon surface functionalization (see Fig. S6, ESI†). By non-linear curve fitting, the second-order rate constants of thiol–maleimide conjugations on the vesicle membranes were determined (Table 1). The rate constants are all in the same order of magnitude. Small deviations along with different combinations of employed thiol and membrane composition can be explained by the course of the thiol–maleimide addition via thiolate anions. Their concentration is directly related to the pH of the reaction medium and to the pKa value of the corresponding thiol. These pKa values in turn are influenced by both the chemical nature of the thiol and the physico-chemical properties in their environment, i.e., by the individual properties of the particular vesicle–water interface.19 Noteworthy, considerably basic conditions drastically enhance the nucleophilicity, however, also accelerate the oxidation of thiols to disulfides. Therefore, the pH value of 7.4 in our vesicular systems poses a good compromise between reaction rate and thiol stability. Compared to literature known rate constants from homogeneous systems (600–8850 M–1 s–1 at pH = 7.0),20 the lower rate constants of about one order of magnitude at the liposomal surfaces are probably due to the lower diffusion rates of vesicles.21
Table 1 Rate constants for vesicle functionalization reactions based on the signal changes of the co-embedded fluorophors
Entry |
Vesicles |
Thiol |
k
obs [M–1 s–1] |
1 |
V-Dans
|
Cys-Trp
|
315 |
2 |
V-Dans
|
ETG
|
108 |
3 |
V-CF
|
ETG
|
455 |
2.2. Preparation of chemosensors for phosphates by post-functionalization of vesicles with Zn-Cyclen2
Next, we utilized the established conditions for the membrane attachment of thiolated species for the preparation of a simple chemosensor. As phosphates are ubiquitous in nature, they pose important targets for analytical devices. Recently, our group reported on the preparation of vesicular chemosensors for phosphates based on amphiphilic derivatives of bis-Zn2+-cyclen complexes.4,7a Those receptors are known to bind to phosphates with nanomolar affinities.22 To demonstrate the proof of principle of our post-functionalization approach and to show its applicability for sensor preparation in analogy to our previous investigations, we synthesized the thiolated bis-Zn2+-cyclen receptor Zn-Cyclen2 (Fig. 3) and immobilized it on maleimide-modified vesicles V-CF. For that purpose, we added a sub-stoichiometric amount of the thiol (0.8 equivalents)23 with respect to the surface-accessible concentration of Mal-C16 in order to avoid the separation of excess Zn-Cyclen2 after vesicle functionalization. Fluorescence measurements revealed a remarkable initial drop of the emission signal (see Fig. S7, ESI†) upon addition of Zn-Cyclen2 followed by a further time-dependent decrease of the fluorescence intensity indicating the progress of the surface reaction (Fig. 6).
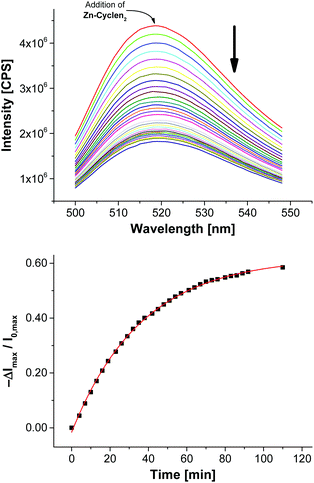 |
| Fig. 6 Time-dependent fluorescence response showing the functionalization progress of vesicles V-CF with 2.0 mol% of Zn-Cyclen2. | |
Compared to the surface reaction of vesicles V-CF with ETG, the functionalization with Zn-Cyclen2 was considerably slower (Table 2, entry 1). We explain this with the occurrence of multiple equilibria diminishing the concentration of free Zn-Cyclen2: (i) The strong complexation abilities of thiol groups towards metal centers like zinc cations are supposed to result in intermolecular Zn2+–SH coordination.24 (ii) Furthermore, the considerable instant quenching of the fluorescence signal after addition of the receptor indicates electrostatic interactions of the bis-Zn2+-cyclen head groups with the negatively charged membrane-embedded carboxyfluorescein derivatives. To further verify these assumptions, we varied the amount of receptor Zn-Cyclen2 since the proposed equilibria are expected to be shifted towards the coordinated species at higher concentrations of Zn-Cyclen2 (entries 2 and 4). Additionally, we performed the surface reaction in the presence of pyrophosphate (PPi, entries 3 and 5) due to its ability to compete for the Zn2+-centered coordination site of the receptor and thus to increase the concentration of free Zn-Cyclen2 thiol functions. The results presented in Table 2 show two obvious trends which confirm our hypothesis: An enhancement of the reaction rate in the presence of PPi and a deceleration of the reaction along with higher thiol concentrations.
Table 2 Rate constants for the functionalization of vesicles V-CF with different amounts of Zn-Cyclen2 in absence or presence of PPi (for fluorescence emission data, see Fig. S7 and S8, ESI)
Entry |
Zn-Cyclen2
|
PPib |
k
obs [M–1 s–1] |
Equivalents with respect to surface-accessible amount of Mal-C16.
Equivalents with respect to total amount of Zn-Cyclen2.
|
1 |
0.8 eq. (2.0 mol%) |
— |
82 |
2 |
1.0 eq. (2.5 mol%) |
— |
78 |
3 |
1.0 eq. (2.5 mol%) |
2.0 eq. |
322 |
4 |
2.0 eq. (5.0 mol%) |
— |
31 |
5 |
2.0 eq. (5.0 mol%) |
2.0 eq. |
202 |
The addition of disulfide precursor of Zn-Cyclen2 (compound 7 in Scheme S2, ESI†) to V-CF leads to a strong decrease of the emission signal supporting the proposed coordinative interaction of the Zn2+-complex with CF-C12. However, as expected no further time-dependent decrease was observed due to the absence of free thiol groups required for the surface attachment (see Fig. S9, ESI†).
After completion of the functionalization process of V-CF with Zn-Cyclen2, the obtained vesicles V-CF-Zn were ready for use as luminescent sensors for phosphate species. Titration of the analyte PPi resulted in a strong increase of their fluorescence emission signal (Fig. 7).
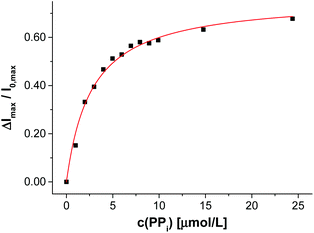 |
| Fig. 7 Fluorescence response upon titration of PPi to functionalized vesicles V-CF-Zn. | |
For control experiments, non-functionalized vesicles were prepared by either omitting Zn-Cyclen2 or by addition of Zn-Cyclen2 to vesicles lacking the membrane-embedded attachment site Mal-C16. Titration of PPi did not show a significant response of the fluorescence signal in those cases (for titration data, see Fig. S10, ESI†). These results proved that the membrane attachment of the receptor molecules is an essential requirement for the sensing mechanism of the PPi analyte molecules.
We examined different phosphate-containing analytes in their binding affinities to vesicles V-CF-Zn and found binding constants which are in good agreement with literature values demonstrating the proof of concept (Table 3). The absence of a fluorescence emission change upon titration of sulfate as a dianionic control analyte confirmed the specificity of V-CF-Zn towards phosphate anions.
Table 3 Binding affinities of Zn-Cyclen2-functionalized vesicles V-CF-Zn to different analytes (for titration data, see Fig. S11, ESI)
Entry |
Analyte |
lg Ka |
Referencea |
Refers to vesicular sensor systems obtained by co-embedding of amphiphilic bis-Zn2+-cyclen receptors into the membrane.
|
1 |
PPi |
6.3 |
6.27a |
2 |
D-Fructose 1,6 bisphosphate |
6.0 |
6.14 |
3 |
Phosphorylated hexapeptide |
5.6 |
5.926a |
Pep-P-His (Fig. 8) |
4 |
SO42– |
No response |
— |
Next, we investigated the dependency of the fluorescence response on the concentration of membrane-embedded fluorophors and immobilized receptor molecules (see Fig. S12, ESI†). Best signal to noise ratios were obtained with increasing amounts of both dye and receptor. These results are consistent with our previous observations and support our mechanistic hypothesis of receptor–dye patches on the vesicle surface, which reorganize upon formation of receptor–analyte complexes.7a
2.3 Molecular imprinting of vesicle surfaces
Our investigations with the Zn-Cyclen2 receptor revealed that the post-functionalization technique is well suited for the preparation of luminescent chemosensors in a modular fashion. Table 3 shows that the obtained binding constants of the different phosphates are almost equal. However, for the development of powerful analytical devices being able to discriminate between structurally related analytes, well-defined multipoint interactions between hosts and guests are essential. That means, the arrangement of the recognition elements on the vesicle surface must be complementary to the position of the binding units at the envisaged targets. Molecular imprinting is the classic technique in polymer science to achieve this aim.25 In this connection, a molecule is utilized as a stamp to create its negative print in a suitable matrix. Recently, our group reported on two different strategies towards the preparation of highly specific, multi-receptor chemosensors. Both approaches are based on dynamic, template-guided recruitment of amphiphilic receptors on fluid vesicle membranes followed by fixation of the assemblies either by cooling the membrane down below the vesicular phase transition temperature26a,b or by photopolymerization of the membrane-embedded receptor arrays.26c A post-functionalization strategy can also be used for the molecular imprinting of defined two-dimensional receptor assemblies since the maleimide-decorated vesicles can be regarded as reactive matrices for template-assisted covalent functionalization. A fundamental prerequisite for that approach is the rigidity of the vesicle membrane preventing the lateral redistribution of the receptors after surface attachment. Hence, DSPC membranes with a phase transition temperature of 54 °C27 represent an ideal scaffold for that purpose. Fig. 8 illustrates the principle of molecular imprinting by vesicle post-functionalization. In detail, membrane-attached receptor–template complexes direct the surface fixation of additional receptor molecules by coordinative interactions in spatially defined arrangements. Subsequent separation of the templates leads to an imprinted vesicle surface with patterns of binding sites.
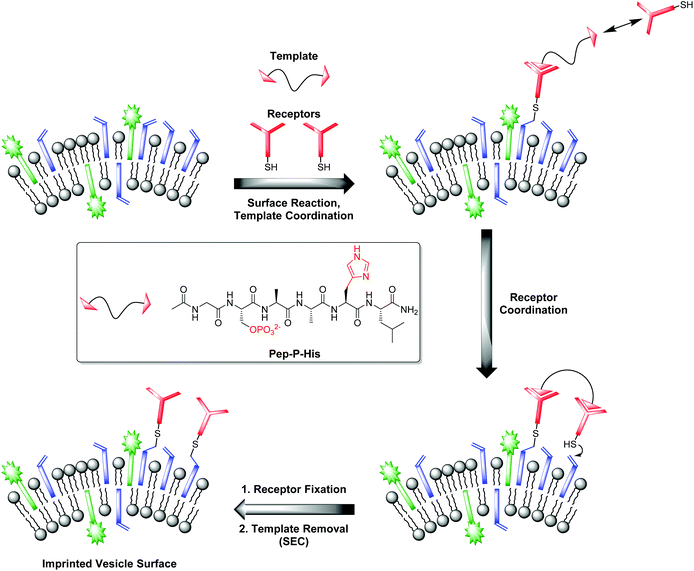 |
| Fig. 8 Principle of vesicle surface imprinting by template-assisted post-functionalization and bivalent peptide Pep-P-His used as model template. | |
Complex Zn-Cyclen2 is known to bind besides phosphates also to histidine moieties.28 As template for our imprinting studies, we therefore utilized bivalent hexapeptide Pep-P-His (Fig. 8) being able to form a ternary complex with two receptor molecules. We incubated a 1
:
2 mixture of the peptide and the thiolated receptor Zn-Cyclen2 with vesicles V-CF (0.4 equivalents of receptor with respect to surface-accessible maleimide) and removed the template molecules by size-exclusion chromatography (SEC) after completion of the surface functionalization. For comparison, vesicle surfaces were post-functionalized with Zn-Cyclen2 in absence of the bivalent peptide, but treated identically apart from that. Rebinding studies of the two obtained vesicle samples revealed a significantly different course of the fluorescence emission responses (Fig. 9). While the imprinted vesicles displayed a fluorescence decrease upon titration of up to 0.5 equivalents of peptide Pep-P-His, the non-imprinted samples showed a steady increase. After further peptide addition, the sign of the fluorescence signal change reversed in case of the imprinted vesicles and the curve progression approximated that of the non-imprinted ones.
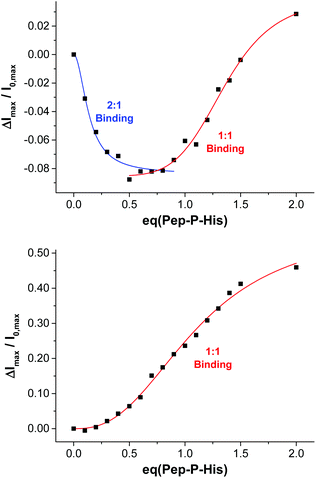 |
| Fig. 9 Rebinding isotherms of imprinted (top) and non-imprinted (bottom) vesicles V-CF-Zn upon addition of Pep-P-His. | |
This divergent behavior can be rationalized by different binding modes in the two cases. We hypothesize that the geometric confinement of the functional amphiphiles on the vesicle surface upon the formation of 2
:
1 complexes results in a further quenching of the co-embedded fluorophors. Gradual transition into 1
:
1 binding mode after addition of more than 0.5 equivalents of the peptide is assumed to provide a higher translational freedom of the membrane-embedded compounds leading to an emission intensity increase of the dye molecules.29 Due to the superposition of two opposing effects in the case of imprinted vesicles, the overall fluorescence response is much lower compared to that of the non-imprinted samples. The macroscopic binding constants in the two cases obtained by Hill fitting of the titration curves are lg
Ka = 7.1 for the imprinted vesicles (based on the addition of up to 0.8 equivalents of Pep-P-His) versus 5.1 for the control experiment.30 However, due to the superposition of two diverging signal responses, the apparent affinity constant for the multipoint binding is likely to be underestimated.
Variation of the receptor concentration indicated that the success of vesicle surface patterning is dependent on the proportion of added receptor molecules and membrane-embedded maleimides. At an almost equimolar ratio (≈0.8 equivalents of receptor with respect to surface-accessible maleimide), the different binding behavior of imprinted and non-imprinted samples is much less pronounced (see Fig. S13, ESI†). We explain this result with the fact that here, approximately all surface-accessible maleimide molecules bear a receptor unit, i.e., the receptor assembly simply reflects the random assembly of the membrane-embedded Mal-C16. Thus, no external control over their spatial distribution is possible and the receptors cannot attain an appropriate geometric arrangement for multipoint binding to the analyte. Conversely, when immobilizing the receptor in sub-stoichiometric amounts, only membrane-embedded maleimides whose mutual distances are compatible to the formation of ternary receptor–template complexes are functionalized. The other maleimides remain unmodified and thereby do not impede the 2
:
1 binding on the vesicle surface.
2.4 Vesicle functionalization with an aptamer for ampicillin
In living systems, multipoint interactions dominate the molecular recognition phenomena and provide for remarkably high specificities. Thus, to explore the full potential of our technique, we next extended the vesicular post-functionalization strategy to more complex binding units of biological origin. Among those, oligonucleotide-based aptamers represent one of the most exciting and promising class of receptors. Since they are developed by an evolutionary process of repeated selection and amplification steps, aptamers exhibit very high binding affinities and specificities to their targets ranging from small organics to proteins and pathogens.31 In many cases, structurally related analytes can be discriminated on basis of subtle differences such as the presence or absence of methyl or hydroxyl groups. Compared to other biological recognition elements like antibodies, aptamers display some remarkable advantages including low immunogenicity, small size, easy production and modification by established solid-phase synthesis methods and therefore inexpensive commercial availability. Moreover, they are characterized by a high stability and convenient handling. Fluorescence-based methods for the detection of aptamer–target binding typically rely on covalent modification of the oligonucleotides with luminescent reporter moieties.32 Another established technique involves hybridization of the aptamers with accordingly labeled complementary oligonucleotide strands and their subsequent displacement by the analyte molecules. This requirement of covalent attachment of fluorophors can make such assays laborious and costly. Label-free strategies on the other hand, e.g., based on intercalation of a fluorescent dye into a double helix domain, are often limited in their applicability as they depend on particular properties of the aptamers such as specific folding modes upon complex formation with the target. In contrast to these analytical methods, the signaling mechanism of our vesicular sensors is generally supposed to originate rather from an analyte-induced redistribution of charges at the liposome–water interface than on defined conformational changes of the recognition sites. Therefore, aptamer-functionalized vesicles with co-embedded reporter dyes potentially represent versatile systems for the detection of aptamer–target interactions. The modular nature of such aptasensors based on self-assembly and subsequent membrane modification allows for their easy preparation and facile optimization of their composition. Recently, we reported on the post-functionalization of luminescent vesicles with the thrombin-binding aptamer resulting in liposomal sensors, which signal the binding of the protein analyte to their surface.14 To demonstrate the universal applicability of this approach for the preparation of various aptasensors, we utilized a DNA-based aptamer developed by Song et al. which specifically binds to the antibiotic ampicillin (Fig. 10) with an affinity constant of lg
Ka = 8.0.33
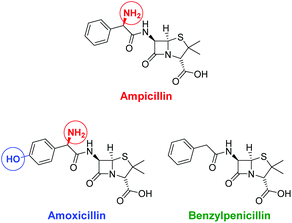 |
| Fig. 10 Structures of the antibiotic ampicillin and of related control antibiotics amoxicillin and benzylpenicillin; structural differences are marked with colored circles. | |
For the selection of the ampicillin aptamer, the authors used TRIS buffer with added salts (50 mm NaCl, 5 mm KCl, 5 mm MgCl2). The presence of cations such as K+ or Mg2+ is important to stabilize the folded analyte binding conformations of some aptamers. On the other hand, it is well explored that in particular bivalent cations can induce aggregation or even fusion of negatively charged vesicles (as it is the case for V-CF) by neutralizing their surface charge and thus by decreasing their electrostatic repulsion barrier.34 In our case, it turned out that non-functionalized vesicles V-CF are indeed unstable and aggregate under the original buffer conditions. For that reason, we screened different buffer systems with respect to both vesicle stability and emission response of aptamer-functionalized vesicles after addition of ampicillin (see ESI† for details). Buffer systems without added salts did not result in significant fluorescence responses, whereas very high salt concentrations induced vesicle aggregation as determined by dynamic light scattering. HEPES buffer with added KCl (5 mm) and MgCl2 (5 mm) represented the best compromise. The surface functionalization of maleimide-containing vesicles V-CF was performed with thiol-modified oligonucleotide Apt-Amp (Fig. 3). A time-dependent change of the emission signal again displayed the progress of the aptamer-immobilization on the vesicular membrane (Fig. 11).
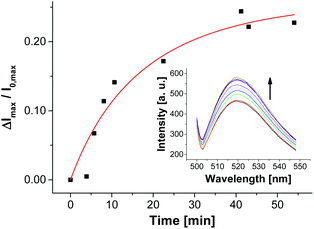 |
| Fig. 11 Fluorescence response showing the immobilization progress of vesicles V-CF with aptamer Apt-Amp (1.0 mol%). | |
In contrast to the post-functionalization of V-CF with receptor Zn-Cyclen2, the fluorescence signal increased during the surface reaction. We believe that the presence of the highly negatively charged oligonucleotides at the vesicle surface partially displaces the likewise negatively charged carboxyfluorescein molecules from the clusters of the functional amphiphiles. As a consequence, a reduction of the fluorophor self-quenching occurs. Binding of ampicillin to the surface-immobilized aptamers is thought to induce a more compact conformation of the aptamer strands. Therefore, a higher negative charge density at the vesicle membrane is generated, which in turn enforces more dye molecules to be squeezed out of their membrane domains. This process gives rise to a significant further increase of the emission signal (Fig. 12).35
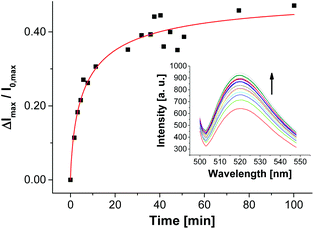 |
| Fig. 12 Time-dependent change of fluorescence emission of aptamer-functionalized vesicles V-CF-Amp (2.0 mol% of Apt-Amp) after addition of ampicillin (0.40 μM). | |
The time-dependent fluorescence spectra depicted in Fig. 12 show a rather slow emission response of V-CF-Amp upon addition of ampicillin. We assume that the aptamer-functionalized vesicles are more prone to salt-induced aggregation than the non-functionalized ones due to their higher surface charge.34 This assumption is supported by investigations of the vesicular size distribution, which revealed a significant cross linking of the vesicles after functionalization with the oligonucleotide (see Fig. S15, ESI†).36 This process in turn reduces the accessibility of the surface-bound aptamers for ampicillin. Binding of the analyte molecules to their membrane receptors, however, seems to partially reverse the aggregation as indicated by a subsequent decrease of the size distribution maximum of the vesicles.37
Variation of the aptamer concentration and addition of ampicillin to the functionalized vesicles gave best emission responses of almost 50% with 2.0 mol% of oligonucleotides immobilized on the surface (Fig. 13). These conditions having approximately equimolar amounts of the receptor molecules with respect to the membrane-embedded fluorophors on the outer vesicle provide highest sensitivities, which is in accordance with our results from Zn-Cyclen2-functionalized vesicles.
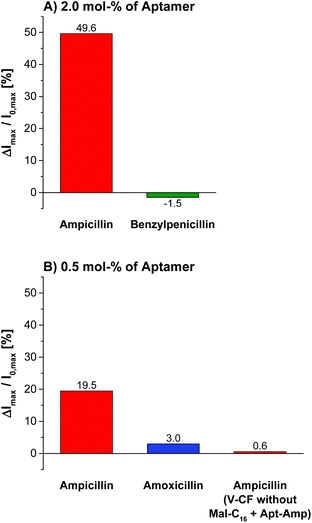 |
| Fig. 13 Comparison of relative fluorescence emission responses of V-CF-Amp, functionalized with different amounts of the aptamer, and of a mixture of maleimide-lacking vesicles V-CF and Apt-Amp after addition of ampicillin, benzylpenicillin or amoxicillin (0.40 μM in each case). | |
The specificity of the aptasensors V-CF-Amp towards ampicillin was illustrated by addition of the structurally related antibiotics benzylpenicillin or amoxicillin, which showed no significant fluorescence responses of the membrane-embedded carboxyfluorescein dyes (Fig. 13). Vesicles lacking the amphiphilic attachment site for thiols Mal-C16 were prepared and incubated with the aptamer. Addition of ampicillin to that sample did not lead to a change of the emission. This observation proves that the analyte signaling depends on the interactions with the membrane-conjugated aptamers.
3. Conclusion
In summary, we have demonstrated that preformed, maleimide-decorated luminescent vesicles can be easily functionalized at the surface with different receptors by nucleophilic thiol addition. It was shown that the immobilized compounds may range from small organic molecules to peptides and metal complexes, such as bis-Zn2+-cyclen, and to even more complex structures like oligonucleotides. The membrane-embedded fluorophors respond to the presence of the receptor units and to the formation of host–guest complexes on the vesicle surface with changing emission properties. We demonstrated potential applications of the concept including the detection of simple phosphates with surface-attached Zn-Cyclen2 or the specific sensing of ampicillin by an aptamer. Moreover, we showed that the post-functionalization strategy allows external control over the two-dimensional distribution of the receptors by assistance of multivalent templates. Here, significant differences between imprinted and non-imprinted samples were obtained in the fluorescence output of rebinding titrations. Due to the highly modular approach, our presented strategy allows an easy, fast and inexpensive access to a broad variety of supramolecular indicators with tailor-made properties. The non-functionalized vesicles can be prepared in advance. Since many heterobifunctional reagents for the in situ introduction of thiol groups to amines are commercially available (e.g., Traut's reagent38a or SATA38b), our method is not only limited to thiol-containing host molecules, but can be readily extended to recognition elements based on other peptides, oligonucleotides or complex macromolecules. Hence, the development of novel customized bioanalytical devices may be facilitated.
Acknowledgements
A. M. thanks the Bayerische Eliteförderung for a research fellowship.
Notes and references
-
(a) J. Voskuhl and B. J. Ravoo, Chem. Soc. Rev., 2009, 38, 495–505 RSC
;
(b) K. Ariga, H. Ito, J. P. Hill and H. Tsukube, Chem. Soc. Rev., 2012, 41, 5800–5835 RSC
.
-
(a) K. A. Edwards and A. J. Baeumner, Talanta, 2006, 68, 1421–1431 CrossRef CAS PubMed
;
(b) Q. Liu and B. J. Boyd, Analyst, 2013, 138, 391–409 RSC
.
- R. Martínez-Máñez and F. Sancenón, Chem. Rev., 2003, 103, 4419–4476 CrossRef PubMed
.
- B. Gruber, S. Stadlbauer, K. Woinaroschy and B. König, Org. Biomol. Chem., 2010, 8, 3704–3714 CAS
.
- T. W. Bell and N. M. Hext, Chem. Soc. Rev., 2004, 33, 589–598 CAS
.
- B. T. Nguyen and E. V. Anslyn, Coord. Chem. Rev., 2006, 250, 3118–3127 CrossRef CAS
.
-
(a) B. Gruber, S. Stadlbauer, A. Späth, S. Weiss, M. Kalinina and B. König, Angew. Chem., Int. Ed., 2010, 49, 7125–7128 (
Angew. Chem.
, 2010
, 122
, 7280–7284
) CrossRef CAS PubMed
;
(b) S. Banerjee, M. Bhuyan and B. König, Chem. Commun., 2013, 49, 5681–5683 RSC
.
-
(a) M. Mammen, S.-K. Choi and G. M. Whitesides, Angew. Chem., Int. Ed., 1998, 37, 2754–2794 (
Angew. Chem.
, 1998
, 110
, 2908–2953
) CrossRef
;
(b) H. Jung, A. D. Robison and P. S. Cremer, J. Struct. Biol., 2009, 168, 90–94 CrossRef CAS PubMed
;
(c) A. Grochmal, E. Ferrero, L. Milanesi and S. Tomas, J. Am. Chem. Soc., 2013, 135, 10172–10177 CrossRef CAS PubMed
.
-
(a) L. Nobs, F. Buchegger, R. Gurny and E. Allémann, J. Pharm. Sci., 2004, 93, 1980–1992 CrossRef CAS PubMed
;
(b) V. P. Torchilin, Nat. Rev. Drug Discovery, 2005, 4, 145–160 CrossRef CAS PubMed
;
(c) F. S. Hassane, B. Frisch and F. Schuber, Bioconjugate Chem., 2006, 17, 849–854 CrossRef CAS PubMed
;
(d) R. I. Jølck, L. N. Feldborg, S. Andersen, S. M. Moghimi and T. L. Andresen, Adv. Biochem. Eng./Biotechnol., 2011, 125, 251–280 CrossRef
.
-
(a) C. E. Hoyle and C. N. Bowman, Angew. Chem., Int. Ed., 2010, 49, 1540–1573 (
Angew. Chem.
, 2010
, 122
, 1584–1617
) CrossRef CAS PubMed
;
(b) C. E. Hoyle, A. B. Lowe and C. N. Bowman, Chem. Soc. Rev., 2010, 39, 1355–1387 RSC
.
- V. D. Bock, H. Hiemstra and J. H. van Maarseveen, Eur. J. Org. Chem., 2006, 51–68 CrossRef CAS
.
- C. E. Hoyle, T. Y. Lee and T. Roper, J. Polym. Sci., Part A: Polym. Chem., 2004, 42, 5301–5338 CrossRef CAS
.
-
(a) B. Frisch, C. Boeckler and F. Schuber, Bioconjugate Chem., 1996, 7, 180–186 CrossRef CAS PubMed
;
(b) P. Schelte, C. Boeckler, B. Frisch and F. Schuber, Bioconjugate Chem., 2000, 11, 118–123 CrossRef CAS
;
(c) R. J. Pounder, M. J. Stanford, P. Brooks, S. P. Richards and A. P. Dove, Chem. Commun., 2008, 5158–5160 RSC
.
- A. Müller and B. König, Chem. Commun., 2014, 50, 12665–12668 RSC
.
- Y. Kitano, Y. Nogata, K. Matsumura, E. Yoshimura, K. Chiba, M. Tada and I. Sakaguchi, Tetrahedron, 2005, 61, 9969–9973 CrossRef CAS
.
- P. Wu and L. Brand, Anal. Biochem., 1994, 218, 1–13 CrossRef CAS PubMed
.
- J. Guy, K. Caron, S. Dufresne, S. W. Michnick, W. G. Skene and J. W. Keillor, J. Am. Chem. Soc., 2007, 129, 11969–11977 CrossRef CAS PubMed
.
- For examples of optical probes for thiol detection, see: X. Chen, Y. Zhou, X. Peng and J. Yoon, Chem. Soc. Rev., 2010, 39, 2120–2135 RSC
.
- M. P. Lutolf, N. Tirelli, S. Cerritelli, L. Cavalli and J. A. Hubbell, Bioconjugate Chem., 2001, 12, 1051–1056 CrossRef CAS PubMed
.
- J. Li, Q. Xu, D. M. Cortes, E. Perozo, A. Laskey and A. Karlin, Proc. Natl. Acad. Sci. U. S. A., 2002, 99, 11605–11610 CrossRef CAS PubMed
.
- P. Schelté, C. Boeckler, B. Frisch and F. Schuber, Bioconjugate Chem., 2000, 11, 118–123 CrossRef
.
-
(a) T. Koike, S. Kajitani, I. Nakamura, E. Kimura and M. Shiro, J. Am. Chem. Soc., 1995, 117, 1210–1219 CrossRef CAS
;
(b) E. Kimura, S. Aoki, T. Koike and M. Shiro, J. Am. Chem. Soc., 1997, 119, 3068–3076 CrossRef CAS
;
(c) S. Aoki, M. Zulkefeli, M. Shiro, M. Kohsako, K. Takeda and E. Kimura, J. Am. Chem. Soc., 2005, 127, 9129–9139 CrossRef CAS PubMed
.
- With respect to a total concentration of Mal-C16 of 5.0 mol% and therefore a surface-accessible concentration of approximately 2.5 mol%, 0.8 equivalents of Zn-Cyclen2 equate to 2.0 mol% relating to the total amphiphile concentration.
- S. Lacerda, M. P. Campello, I. C. Santos, I. Santos and R. Delgado, Polyhedron, 2007, 26, 3763–3773 CrossRef CAS
.
- G. Wulff, Angew. Chem., Int. Ed. Engl., 1995, 34, 1812–1832 (
Angew. Chem.
, 1995
, 107
, 1958–1979
) CrossRef CAS
.
-
(a) B. Gruber, S. Balk, S. Stadlbauer and B. König, Angew. Chem., Int. Ed., 2012, 51, 10060–10063 (
Angew. Chem.
, 2012
, 124
, 10207–10210
) CrossRef CAS PubMed
;
(b) S. Balk and B. König, J. Inclusion Phenom. Macrocyclic Chem., 2014 DOI:10.1007/s10847-014-0442-2
;
(c) S. Banerjee and B. König, J. Am. Chem. Soc., 2013, 135, 2967–2970 CrossRef CAS PubMed
.
- M. Ueno, S. Katoh, S. Kobayashi, E. Tomoyama, S. Ohsawa, N. Koyama and Y. Morita, J. Colloid Interface Sci., 1990, 134, 589–592 CrossRef CAS
.
- A. Grauer, A. Riechers, S. Ritter and B. König, Chem. – Eur. J., 2008, 14, 8922–8927 CrossRef CAS PubMed
.
- In our previously reported dynamic imprinting approaches,26 we observed a steady increase of the signal in both cases, however, with the different binding affinities being reflected in different slopes of the binding isotherms. We explain this with the dynamic nature of the receptor recruitment process in that case providing a diverse arrangement of the fluorophor molecules relative to the receptor assemblies.
- The lower value of lg
Ka in case of non-imprinted vesicles compared to that from Table 3 might be attributed to an incomplete separation of the template peptide by the SEC in that case. However, as the exactly identical conditions for SEC were applied for both the imprinted vesicles and the non-imprinted control solutions, the relative change of the binding affinities has to be considered.
-
(a) S. D. Jayasena, Clin. Chem., 1999, 45, 1628–1650 CAS
;
(b) C. K. O'Sullivan, Anal. Bioanal. Chem., 2002, 372, 44–48 CrossRef PubMed
.
-
(a) E. J. Cho, J.-W. Lee and A. D. Ellington, Annu. Rev. Anal. Chem., 2009, 2, 241–264 CrossRef CAS PubMed
;
(b) R. E. Wang, Y. Zhang, J. Cai, W. Cai and T. Gao, Curr. Med. Chem., 2011, 18, 4175–4184 CrossRef CAS PubMed
.
- K.-M. Song, E. Jeong, W. Jeon, M. Cho and C. Ban, Anal. Bioanal. Chem., 2012, 402, 2153–2161 CrossRef CAS PubMed
.
- S. Nir, J. Bentz, J. Wilschut and N. Düzgünes, Prog. Surf. Sci., 1983, 13, 1–124 CrossRef CAS
.
- The surface-immobilized Zn-Cyclen2 complexes, on the other hand, are supposed to electrostaticly interact with the membrane-embedded carboxyfluorescein molecules resulting in a partial quenching of their fluorescence emission.
- Vesicle aggregation based on specific intermolecular interactions between surface-attached oligonucleotides is unlikely due to the absence of complementary sequences in the immobilized aptamer required for hybridization.
- We did not face that aggregation behavior in our previously reported studies regarding the vesicular thrombin-aptasensor.14 There, the presence of Mg2+ was not required for the conformational stabilization of the oligonucleotides. As a result, a dynamic and quick fluorescence response over a wide concentration range was observed.
-
(a) R. Jue, J. M. Lambert, L. R. Pierce and R. R. Traut, Biochemistry, 1978, 17, 5399–5406 CrossRef CAS PubMed
;
(b) R. Julian, S. Duncan, P. D. Weston and R. Wrigglesworth, Anal. Biochem., 1983, 132, 68–73 CrossRef
.
Footnote |
† Electronic supplementary information (ESI) available: Experimental details, synthesis of compounds, preparation of functionalized vesicles, emission spectra, binding curves and optimization studies. See DOI: 10.1039/c4ob02327g |
|
This journal is © The Royal Society of Chemistry 2015 |