DOI:
10.1039/C3BM60079C
(Paper)
Biomater. Sci., 2013,
1, 736-744
Comparative study of guanidine-based and lysine-based brush copolymers for plasmid delivery†
Received 21st March 2013, Accepted 16th April 2013
First published on 25th April 2013
Abstract
Polyethylenimine (PEI), one of the most frequently used polycations for non-viral nucleic acid delivery, exhibits good transfection efficiency to cultured cells but generally has to be used in restricted concentration ranges due to high cytotoxicity. We recently reported a family of HPMA-co-oligolysine brush copolymers that show nucleic acid delivery efficiencies approaching that of PEI. Guanidine-containing polymers have been reported in some systems to be more effective at cellular delivery of cargo than their primary-amine analogs. The goal of this work is to investigate the effect of guanidinylation on gene transfer ability of HPMA-co-oligolysine copolymers. Several parameters were evaluated: arginine versus homoarginine monomers, oligopeptide length, and charge density within the peptide. Using reversible addition-fragmentation chain transfer (RAFT) polymerization, a series of six copolymers were synthesized containing the cationic peptides K10, R10, K5, and (GK)5. Lysine-containing copolymers were functionalized with guanidine by reaction with O-methylisourea to generate an additional five homoarginine-based copolymers. All eleven copolymers readily condensed into small polyplexes (<250 nm) and remained stable in physiological salt conditions. The best performing copolymers provided more efficient gene transfection with less associated cytotoxicity than PEI. Reducing the number of charge centers (from 10 to 5) further reduced toxicity while retaining comparable transfection efficiency to PEI.
Introduction
The Tat peptide is a basic, arginine-rich domain of the 86 amino acid Tat protein from HIV-1 that is a critical motif for cellular uptake.1 The importance of the arginine groups, and more specifically the guanidine groups, in mediating intracellular delivery has been reported by the Sugiura and Wender groups.2,3 While these peptides were initially thought to be cell-penetrating by passing through the cell membrane directly into the cytoplasm, Richard et al. showed that arginine-rich peptides enter cells by endocytosis.4 There have been several arginine-based carriers developed for intracellular nucleic acid delivery in the past decade.5–9 These carriers have demonstrated efficient transfection of plasmid DNA and siRNA both in vitro and in vivo.Lysine is another amino acid often used in synthetic gene delivery systems due to its positive charge at neutral pH. Poly-(L)-lysine (PLL) was one of the earliest polycations used for non-viral gene transfer to mammalian cells.10 There have been several comparisons in the literature of cationic transfection reagents based on lysine versus arginine, and the relative transfection efficiency between the two types of carriers appears to be highly structure dependent. For example, Pouton et al. showed that polyarginine polymers transfect cells with plasmids much more poorly than polylysine polymers.11 In contrast, branched oligoarginine-based peptides generally delivered plasmid to K562 cells better than their branched oligolysine counterparts.12 In addition, poly(amido amine) (PAMAM) dendrimers modified with L-arginine show higher plasmid transfection efficiency than PAMAM dendrimers modified with L-lysine.13
Our group recently reported a family of peptide-based polycations synthesized by RAFT copolymerization of oligolysine macromonomers with HPMA (hydroxypropylmethacrylamide).14–16 The comb structure of these copolymers afforded much higher transfection efficiency than linear poly(L)lysine. We optimized the oligolysine polymers by varying the oligolysine monomer content, the oligolysine length, and the total molecular weight of the copolymers. We found that polymers with 20 mol% oligolysine, containing either K5 or K10, and of polymer with degree of polymerization (DP) of 150 or 190 provided high transfection efficiency.15 We have further demonstrated that multiple peptide entities, such as targeting ligands or endosomal release sequences, can be readily incorporated into these materials to further improve gene delivery efficiencies.14,17
In this work, we synthesize a panel of HPMA-co-peptide copolymers with comb-like architecture to test the effect of charge moiety (amine vs. guanidine) on cell transfection efficiency and cytotoxicity. Guanidinylated polymers were synthesized by both polymerization of oligoarginine monomers prepared by solid phase peptide synthesis (SPPS) and by post-polymerization guanidinylation of HPMA-co-oligolysine copolymers with O-methylisourea; the latter approach yields homoarginine copolymers. The effect of comb length (pentamer or decamer peptides), peptide density, and charge density in individual peptides on gene transfection efficiency and cytotoxicity was also investigated. From this work, several HPMA-co-peptide copolymers that surpass branched polyethylenimine (bPEI) in transfection efficiency without additional cytotoxicity were identified.
Materials and methods
Materials
N-(2-Hydroxypropyl)methacrylamide (HPMA) was purchased from Polysciences (Warrington, PA), VA-044 initiator from Wako Chemicals USA (Richmond, VA), reagents used in solid phase peptide synthesis from Apptec (Louisville, KY), O-benzotriazole-N,N,N′,N′-tetramethyl-uronium-hexafluoro-phosphate) from Advanced Chem Tech (Louisville, KY), N-succinimidyl methacrylate from TCI America (Portland, OR), and all other chemicals including O-methylisourea and bPEI (average Mw ∼ 25
000 by LS, average Mn ∼ 10
000 by GPC) from Sigma-Aldrich (St. Louis, MO). The chain transfer agent ethyl cyanovalerictrithiocarbonate (ECT) was generously provided by Anthony Convertine (University of Washington). All cell culture reagents were purchased from Cellgro/Mediatech (Fisher Scientific, Pittsburgh PA). Endotoxin-free plasmid pCMV-Luc2 was isolated using the Qiagen Plasmid Giga Kit (Qiagen, Germany) according to the manufacturer's instructions. BCA protein quantification assay kits were obtained from Thermo Fischer Scientific (Waltham, MA), and luciferase expression quantification kits were obtained from Promega (Madison, WI).Synthesis of peptide monomers
A total of four methacrylamido-functionalized polycationic peptides containing a methacrylated (Ma) 6-aminohexanoic acid (Ahx) linker, MaAhxKKKKKKKKKK (MaAhxK10), MaAhxRRRRRRRRRR (MaAhxR10), MaAhxKKKKK (MaAhxK5), and MaAhxGKGKGKGKGK (MaAhx(GK)5), were synthesized using standard Fmoc/tBu SPPS techniques on an automated PS3 peptide synthesizer (Protein Technologies, Phoenix, AZ). Synthesized peptides were deprotected and cleaved from the solid phase resin supports using a solution of trifluoroacetic acid (TFA)–triisopropylsilane (TIPS)–1,3-dimethoxybenzene (DMB) (92.5
:
2.5
:
5, v/v/v) for 3 h (4 h in the case of MaAhxR10) under constant gentle mixing. The cleaved product was collected in three rounds of precipitation into cold ether, pelleting and dissolving in a minimum amount of methanol. After the third ether precipitation, the peptides were dried under vacuum, dissolved and frozen in a minimum volume of double distilled water (ddH2O), and lyophilized for long term storage. Each peptide was analyzed by RP-HPLC and shown to have greater than 95% purity. MALDI-TOF was used to confirm each synthesis reaction and all measured masses matched targeted masses. Measured mass for MaAhxK10 was 1480.11, expected 1479.7; measured mass for MaAhxR10 was 1760.4, expected 1759.4; measured mass for MaAhxK5 was 839.6, expected 838.8; and measured mass for MaAhx(GK)5 was 1124.6, expected 1124.1.Guanidinylation of HPMA-co-oligolysine copolymers. From each lysine-containing copolymer, 100 mg of copolymer was converted to a homoarginine-based copolymer by reaction with O-methylisourea in 10-fold molar excess to lysine as described previously.18 In brief, copolymer and O-methylisourea were each dissolved in 10 mL of 1
:
1 (v/v) saturated Na2CO3 and ddH2O and fully dissolved before combining in a round bottom flask sealed with a rubber septum. Reactions were carried out at room temperature under constant stirring under a stirbar for 72 h, followed by dialysis against ddH2O (MWCO = 10
000 Da) for an additional 72 h, replacing the dialysate every 18–24 hours and then lyophilized. Complete conversion of side chain primary amines to guanidine groups was confirmed by amino acid analysis of polymers. Characterization of copolymers
Size exclusion chromatography. Copolymers were dissolved in 0.15 M sodium acetate buffer, pH 4.4, to a final concentration of 10 mg mL−1. Molecular weight analysis was carried out using size exclusion chromatography (SEC) as described by Hennink et al.14 with analysis using a miniDAWN TREOS light scattering detector and an Optilab rEX refractive index detector (Wyatt). Molecular weight averages (Mn and Mw) as well as dn/dc were calculated using the ASTRA software (Wyatt). From this data, average molecular weight and the polydispersity index for each copolymer was calculated. Amino acid analysis. Amino acid analysis was used to determine the relative amount of amino acid to HPMA in each polymer, as well as to confirm complete conversion of lysine to homoarginine in the guanidinylated copolymers as described previously.15 In brief, copolymers were hydrolyzed in 6 N heated HCl for 24 h and derivatized with o-phthalaldehyde/β-mercaptopropionic acid. These derivatives were run on a Poroshell 120 EC-C18 (Agilent Technologies, Santa Clara, CA) HPLC column with a precolumn derivatization to label hydrolyzed amino acids (lysine, arginine, glycine, and 1-amino-2-propanol). Calibration curves were generated using serial dilutions of reagent grade amino acids and 1-amino-2-propanol. Characterization of polyplexes
Polyplex formation. The pCMV-Luc2 plasmid was diluted in double distilled H2O (ddH2O) to a concentration of 0.1 mg ml−1 and mixed with an equal volume of copolymer solution by adding polymer solution to DNA solution at the desired cation to anion (N/P) ratio. After mixing, polyplexes were incubated for 5 minutes at room temperature.
Polyplex size and zeta potential determination by dynamic light scattering. Polyplexes (1 μg DNA, 40 μL solution) were mixed with either 60 μL of double distilled H2O or phosphate buffered saline (PBS, 150 mM) and then polyplex size determined by dynamic light scattering (DLS, Brookhaven Instruments Corp ZetaPLUS). Particle sizing measurements were performed at a wavelength of 659.0 nm with a detection angle of 90° at RT. Measurements were taken over five 30 second intervals, each polyplex type was also formulated and measured in triplicate. Zeta potential experiments were conducted using a Zetasizer Nano ZS (Malvern Instruments Inc., Southborough, MA). Polyplexes (1 μg of DNA, 20 μL of solution, N/P of 5 and 3) were mixed with 180 μL of double distilled H2O and 800 μL of 10 mM NaCl solution.
Determination of polyplex condensation by gel electrophoresis. The binding strength of the copolymers was tested using a gel retardation assay on complexed polyplexes formed with N/P ratios ranging from 0 to 2. Polyplexes were electrophoresed through a 1% (w/v) agarose gel containing ethidium bromide under constant 80 mA current in TAE buffer solution comprised of 40 mM Tris-HCl, 1% (v/v) acetic acid, and 1 mM EDTA.
Cell culture
In vitro luciferase plasmid transfection. Human epithelial adenocarcinoma cells (HeLa, passage 17), ATCC #CCL-2) were seeded in 10% FBS and 1% AbAm antibiotic supplemented MEM culture medium onto a 24-well plate at a density of 2.5 × 104 cells per well. Cells were placed in a 37 °C, 5% CO2, humidified incubator for 24 h to ensure attachment of cells to the plate surface. Polyplexes were formed as previously described at N/P ratios of 3 and 5 using 1 μg of pCMV-Luc2 plasmid DNA in 20 μL of total volume.17 Total protein content for each well was also measured using a BCA protein assay kit (Thermo Scientific, Rockford, IL) according to the manufacturer's instructions. Protein content was used to normalize luciferase expression as well as quantify cell population viability. In vitro EGFP plasmid transfection. HeLa cells were seeded overnight in 24-well plates at a density of 2.5 × 104 cells per well (0.5 mL per well) at 37 °C, 5% CO2. Polyplexes were formulated as described previously and then diluted to 200 μL with OptiMEM medium (Invitrogen) and added to cells after washing twice with PBS. The cells were incubated at 37 °C, 5% CO2 for 4 h, washed and then incubated with complete cell culture media at 37 °C, 5% CO2 for another 44 h. For analysis, cells were washed with PBS, trypsinized and pelleted at 1000 × g for 5 min at 4 °C. The pellet was resuspended in 0.2 mL propidium iodide (PI) solution (1 μg mL−1 in 0.5% BSA in PBS), kept on ice and analyzed using flow cytometry, MACSQuant Analyzer (Miltenyi Biotec Inc., Auburn, CA). Intact cells were identified using the forward and side scatter data. The resulting cell population were gated into GFP+/PI+, GFP+/PI−, GFP−/PI+ and GFP−/PI− based on the green fluorescence and PI intensity from the control samples (cells transfected without the polymers but DNA only) and reported as the mean percentage of cell population that is GFP+/PI− including standard deviation (SD). All experiments were conducted in quadruplicate.
Statistical analysis. All relevant statistical analyses were conducted by comparing two samples using the student's t-test, using a two-tailed analysis and assuming unequal variance. Samples were considered statistically significant if p < 0.05.
Results and discussion
Synthesis and evaluation of HPMA-co-peptide copolymers based on decamers of lysine, arginine and homoarginine for plasmid delivery
Polymer synthesis and characterization. Guanidine-based polymers have been prepared using guanidine-containing monomers or by guanidinylation of primary amine-containing polymers.6,18–21 Therefore, guanidine-based HPMA-co-peptide copolymers were synthesized by polymerization of oligoarginine peptide monomers or by guanidinylation of oligolysine-based copolymers (Scheme 1). Guanidinylation of lysine-based copolymers is more cost effective than polymerization of oligoarginine monomers. The synthesis of oligoarginine peptides by sequential coupling of Fmoc-amino acid, containing a bulky side-chain protecting group Pbf, has proven to be difficult. Even with double coupling step and longer time for TFA side-chain deprotection, peptide synthesis resulted in low (<30%) overall peptide yields after purification. Thus, we looked into a more efficient way of preparing copolymers with guanidine-groups.18,22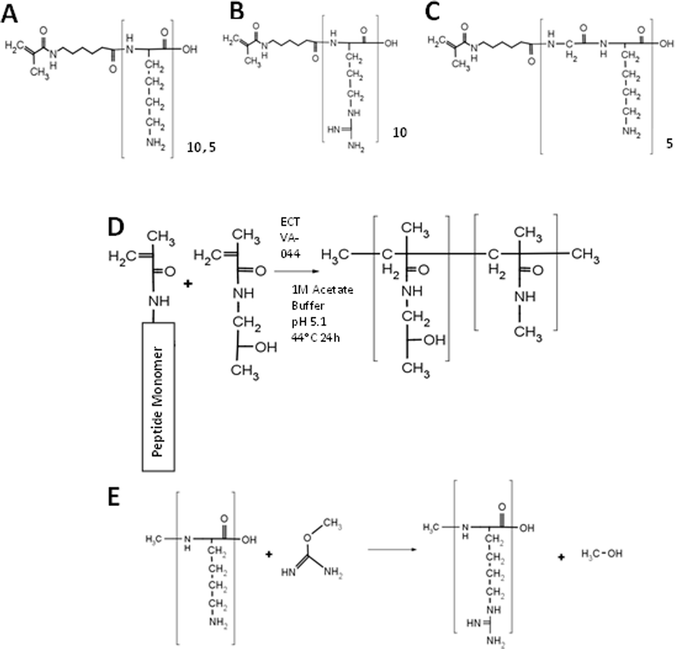 |
| Scheme 1 Polymer synthesis. (A–C) Structure of monomers: MaAhxK10 (A), MaAhxR10 (B), and MaAhx(GK5) (C). (D) Synthesis of peptide brush copolymers by RAFT copolymerization. (E) Guanidinylation reaction between a primary amine and O-methyl isourea to form homoarginine. | |
Decamer peptide copolymers HPMA-co-MaAhxK10 and HPMA-co-MaAhxR10 were synthesized by RAFT copolymerization of HPMA with MaAhxK10 or MaAhxR10 monomers and HPMA-co-MaAhxhR10 polymers were synthesized by reaction of O-methylisourea with HPMA-co-MaAhxK10 copolymers. We showed previously that statistical peptide-based copolymers with good control over polymer composition are synthesized using this approach.14 Amino acid analysis confirmed that guanidinylation efficiency was >99%. The initial set of polymers was synthesized with ∼20 mol% peptide monomer content and with a target degree of polymerization (DP) of ∼180–190. Copolymer properties (molecular weight, polydispersity, and composition) are reported in Table 1. Measured molecular weights were within 1% of target molecular weights and polydispersity index (PDI) of polymers was below 1.2. Amino acid analysis confirmed that polymers contained ∼20% peptide.
Characterization of polyplexes. The polymers were tested as gene delivery materials to probe the effect of basic amino acid selection on transfection efficiency. Polyplexes (polymer–plasmid complexes) were formed by mixing the two components in aqueous solution at various amine to phosphate (N/P) ratios and DNA complexation monitored by gel electrophoresis (Fig. S1†). Complete complexation was observed at N/P of 1.5 for all copolymers. Based on these results, polyplexes formed at N/P = 3 and 5 in water and in physiological salt concentrations were characterized for average size and surface charge by dynamic light scattering (Fig. 1). All three decamer peptide copolymer formulations formed small, positively-charged complexes with DNA in water, regardless of N/P ratio (zeta potentials ∼+15–20 mV and diameters <200 nm). In 150 mM PBS, particles remained relatively stable with particle diameter <250 nm, with an exception of HPMA-co-MaAhxR10 particles formed at N/P = 3. While polyplexes formed from polycations such as PLL or PEI (polyethylenimine) flocculate in physiologic salt concentrations, polyplexes formed from HPMA-co-peptide copolymers were previously shown to exhibit salt stability at these peptide incorporation ratios due to the hydrophilic HPMA comonomer.15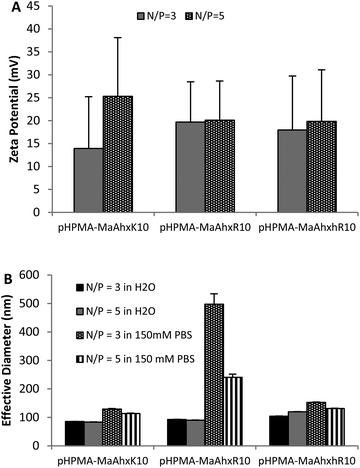 |
| Fig. 1 Zeta potential (A) and particle sizing (B) of decamer polymer polyplexes measured by dynamic light scattering (DLS). Polyplexes were formed in water and allowed to form for 5 minutes. After addition of either water or 150 mM PBS, mixtures were measured by DLS. Data is presented as mean ± SD, n = 3. | |
In vitro transfection studies. Plasmid delivery efficiency to HeLa cells using the three polymers was assessed by delivering the luciferase reporter plasmid. Branched polyethylenimine (bPEI, 25k) complexed with plasmid at N/P = 5 was used a standard for comparison. Analysis of luciferase plasmid delivery using polyplexes at N/P = 3 and 5 revealed that N/P = 5 yielded higher transfection efficiency than N/P = 3 for all tested polymers. These N/P ratios were selected because previous tests of N/P ratios (N/P = 3, 5, 7, and 10) with oligolysine decamer peptide copolymers determined that N/P ratios of 3 and 5 resulted in optimal transfection efficiency, avoiding the increased cytotoxicity observed at higher polymer concentrations (Fig. S2†). While all three HPMA-co-peptide copolymers transfected HeLa cells efficiently (∼3 orders of magnitude higher luciferase activity compared to naked DNA), plasmid delivery using HPMA-co-MaAhxR10 copolymers provided the highest reporter gene expression, approximately 2.3-fold greater than PEI (Fig. 2A).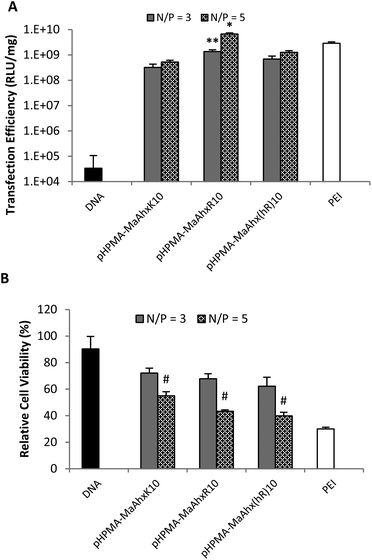 |
| Fig. 2 Luciferase plasmid transfection efficiency (A) and cytotoxicity (B) using polyplexes from HPMA-co-MaAhxK10, HPMA-co-MaAhxR10, and HPMA-co-MaAhxhR10 at N/P ratios of 3 and 5. Naked DNA and bPEI (25 kD) controls are included for comparison. Data presented as mean ± SD, n = 3. * greater transfection than PEI (p < 0.05). ** greater transfection than pHPMA-co-MaAhxK10 and pHPMA-co-MaAhx(hR)10 (p < 0.05). # higher cell viability than cells treated with PEI at the same N/P ratio (p < 0.05). | |
Toxicity of HPMA-co-MaAhxK10, HPMA-co-MaAhxR10, and HPMA-co-MaAhxhR10. Cytotoxicity of the decamer peptide copolymers to cultured mammalian cells was determined by measuring total protein concentration in cell lysates 48 hours after transfection and comparing to untreated cells (Fig. 2B). Both guanidinylated polymers were more cytotoxic than lysine-based polymers but were less toxic than PEI polyplexes prepared at the same charge ratio. This effect was previously reported by Tziveleka and coworkers for their guanidinylated poly(propylene imine) dendrimers.23 Toxicity was greater at higher charge ratios (54 ± 3%, 43 ± 1% and 39 ± 3% cell survival, respectively, for HPMA-co-MaAhxK10, HPMA-co-MaAhxR10 and HPMA-co-MaAhxhR10 polyplexes at N/P = 5 compared to 72 ± 3%, 67 ± 4% and 62 ± 6% cell survival at N/P = 3). This increased toxicity at higher N/P is expected and can be attributed to higher concentrations of free, uncomplexed polycation.24 Overall, the HPMA-co-MaAhx(hR)10 copolymers are more effective and better tolerated than PEI at gene transfer to HeLa cells. Synthesis and evaluation of HPMA-co-peptide copolymers based on reduced positive charge density
Increased charge densities in polycations has been correlated with greater cytotoxicity.25 Therefore, we next attempted to decrease the cytotoxicity of the HPMA-co-MaAhxhR10 polymers by (i) decreasing the number of homoarginine residues per peptide monomer (from decamers to pentamers), (ii) decreasing the density of charge in peptide monomers by including glycine spacers between charges and (iii) changing the peptide monomer content in the copolymers. An additional eight HPMA-co-peptide polymers were synthesized using MaAhxK5 and MaAhx(GK)5 monomers and their guanidinylated, homoarginine derivatives at 20 and 40 mol% monomer incorporation (Table 1).Polymer synthesis and characterization. Pentamer peptide copolymers HPMA-co-MaAhxK5 and HPMA-co-MaAhx(GK)5 were synthesized by RAFT copolymerization of HPMA with MaAhxK5 or MaAhx(GK)5 monomers. The copolymers with 40% mole peptide contained the same mole of charge per mole of polymer as the decamer peptide copolymers, while the 20% mole peptide compositions contained half the charge units per polymer. Glycine was selected as a spacer unit to decrease charge density in peptides. HPMA-co-MaAhxhR5 and HPMA-co-MaAhx(GhR)5 copolymers were synthesized by reaction of O-methylisourea with HPMA-co-MaAhxK5 and HPMA-co-MaAhx(GK)5 copolymers, respectively. Amino acid analysis confirmed that guanidinylation efficiency was >99%. Copolymers were synthesized with either 20 of 40 mol% peptide monomer with target degree of polymerization (DP) of ∼180–190. Statistics on each copolymer molecular weight and amino acid composition are reported in Table 1. Measured molecular weights for the 20 mol% copolymers were within 5% of target molecular weights with a PDI below 1.2. Amino acid analysis confirmed that polymers contained ∼20% peptide. Analysis of the 40 mol% peptide copolymers confirmed that the copolymers contained ∼40% peptide with PDI below 1.1, but showed a greater degree of difference between target and measured molecular weight. HPMA-co-MaAhx(GK)5, HPMA-co-MaAhxhR5, and HPMA-co-MaAhx(GhR)5 had measured molecular weights that were 70%, 81%, and 65% of the target molecular weight, respectively. Characterization of polyplexes. The polymers were tested as gene delivery materials to probe the effect of charge density on transfection efficiency. As with the decamer peptide copolymers, polyplexes formed in water at both N/P of 3 and 5 remained compact, with a hydrodynamic diameter less than 100 nm and zeta potential ∼+20–30 mV. Polyplexes were generally remained stable in 150 mM salt solutions, although some size increase was observed with the HPMA-co-MaAhx(GK)5 copolymer (Fig. 3).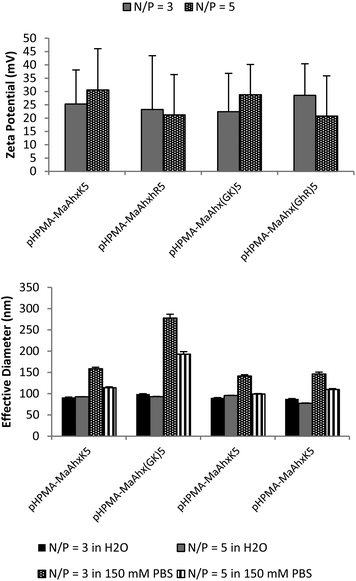 |
| Fig. 3 Zeta potential (A) and particle sizing (B) of 40 mol% pentamer polymer polyplexes measured by dynamic light scattering (DLS). Polyplexes were formed in water and allowed to form for 5 minutes. After addition of either water of 150 mM PBS, mixtures were measured by DLS. Data is presented as mean ± SD, n = 3. | |
In vitro transfection and cytotoxicity. Plasmid delivery efficiency using the eight pentamer peptide copolymers was assessed by luciferase plasmid delivery (Fig. 4A). Transfected HeLa cells showed with luciferase transgene activity ranging from 2–4 orders of magnitude higher than cells transfected with plasmid alone. Plasmid delivery was highest using HPMA-co-MaAhx(GhR)5 (20% peptide incorporation) and HPMA-co-MaAhxhR5 (40% peptide incorporation) polymers, although no copolymer transfected higher than PEI. In general, all guanidinylated copolymers transfected higher than their lysine counterparts by 1–3 orders of magnitude as measured by luciferase activity.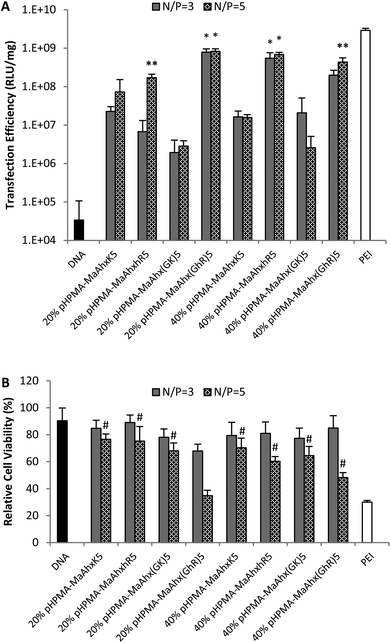 |
| Fig. 4 Luciferase transfection efficiency (A) and relative cytotoxicity (B) of the eight pentamer peptide copolymers. Data is presented as mean ± SD, n = 3. * higher transfection efficiency than all other copolymer with the same peptide incorporation (p < 0.05). # greater cell viability than cells treated with bPEI at the same N/P ratio (p < 0.05). | |
Reducing the charge per peptide from 10 to 5 significantly decreased polymer cytotoxicity (Fig. 4B). For example, when comparing polymers containing the same charge centers per polymer (i.e. HPMA-co-MaAhxhR5 (40% peptide) vs. HPMA-co-MaAhxhR10 (20% peptide)) polymers synthesized using pentamers of charge were better tolerated than polymers synthesized using decamers of charge (60% cell survival vs. 43% cell survival at N/P = 5 in the aforementioned example). Furthermore, increasing peptide content correlated with increases in cell toxicity; for example, cells treated with HPMA-co-MaAhxhR5 (20% peptide) at N/P = 5 showed ∼75% cell survival compared with ∼60% cell survival for cells treated with HPMA-co-MaAhxhR5 (40% peptide). As with the decamer polymers, polyplexes were more toxic when formulated at higher charge ratios. Surprisingly, polymers synthesized with peptides containing glycine-spaced charge groups were more toxic than non-spaced peptides, although these polymers were still less toxic than the decamer analogues. One possible explanation is that the spacing increases the distance of charge from the polymer backbone, which may result in more non-specific protein interactions. Increased spacing between charge center and polymer backbone has been previously shown to correlate with higher polymer cytotoxicity for other polycation systems.26,27 Overall, the cell viability of HPMA-co-peptide formulations was better tolerated than the PEI control.
EGFP plasmid delivery to culture cells. While luciferase assay provides a quick quantification of total transgene expression, the percent of cells transfected is also an important assessment in determining gene transfer efficiency. Therefore, select polymers were tested for delivery of the enhanced green fluorescence protein (EGFP) plasmid to HeLa cells. Specifically, the HPMA-co-MaAhxhR10 (20% peptide), HPMA-co-MaAhxhR5 (40% peptide), and HPMA-co-MaAhx(GhR)5 (40% peptide) polymers which all have the same charge/polymer ratio were selected because they showed high transfection by luciferase assay. In addition, PEI and HPMA-co-MaAhxhK10 polymers were used as controls. Because N/P = 5 was shown to have higher luciferase plasmid delivery compared to N/P = 3, EGFP plasmid transfections were performed using N/P = 5, with analysis of gene expression by flow cytometry (Fig. 5A). Cytotoxicity was determined by propidium iodide exclusion in live cells (Fig. 5B).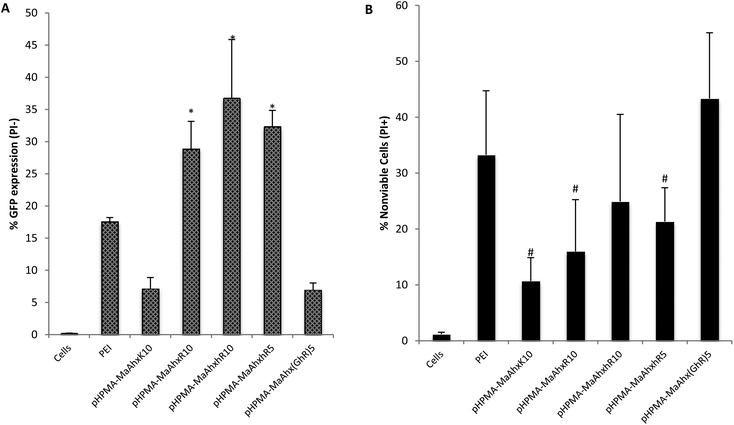 |
| Fig. 5 GFP transfection (A) and % of nonviable cells (B) of HeLa cells as measured by flow cytometry. Cells were transfected using the above copolymers at N/P = 5. Percent GFP+ is given as Mean ± SD for n = 4. * greater percentage of cells GFP+ compared to bPEI treatment (p < 0.05). # lower toxicity compared to PEI treated cells (p < 0.05). | |
By EGFP analysis, the HPMA-co-MaAhxhR10 copolymer provided the highest transfection efficiency (36 ± 9% of live cells EGFP+), with the HPMA-co-MaAhxR10 copolymer transfecting comparably (28.9 ± 5% of live cells EGPF+). EGFP plasmid transfection efficiency for guanidine-based copolymers was up to 5-fold higher compared to lysine-based copolymers. Hashimoto and co-workers recently investigated polyarginine vs. polylysine as gene transfer vectors and demonstrated similar trends of transcription suppression after microinjection into cell nuclei and cytosol, although guanidinylated polymers were more efficient overall at gene transfer. They concluded that the guanidine-containing polymers are more efficient at endosomal escape compared to their primary-amine based analogues by analyzing the difference in transgene expression with and without chloroquine treatment.28 In this work, we further demonstrate that the three guanidinylated polymers showed higher transfection efficiency with less cytotoxicity (cell viability > 75%) compared to PEI, which is hypothesized to use proton sponge buffering for endosomal escape.29,30
Conclusion
In conclusion, we have synthesized a panel of guanidine-based brush copolymers by RAFT polymerization. All copolymers condensed plasmid DNA to salt-stable particles. Chemical guanidinylation was established as resulting in higher yield and greater purity copolymers than direct arginine incorporation while maintaining comparable transfection efficiencies and toxicity profiles. Guanidine-based copolymers exhibit higher transfection efficiency to cultured cells than analogous lysine-based polymers but with higher cytotoxicity. The cytotoxicity of arginine-based polymers could be mitigated by reducing the peptide length without much change in EGFP plasmid delivery efficiency. In contrast, cytotoxicity increased by reducing charge density in peptide brushes via glycine spacers. The copolymers developed and presented here provide a viable alternative to bPEI for in vitro plasmid transfection. Guanidinylation is therefore a straightforward modification that could be used to improve the in vitro and in vivo delivery efficiencies of other primary amine-based polycations used for nucleic acid delivery.Acknowledgements
This work was supported by NIH 1R01NS064404. PMC was supported by a Mary Gates Fellowship for this work. We are grateful to Profs Anthony Convertine and Patrick Stayton for generously providing the ECT chain transfer agent, to Prof. Kim Woodrow for use of the NanoSizer and to David Chu for helpful discussions.Notes and references
- E. Vives, P. Brodin and B. Lebleu, J. Biol. Chem., 1997, 272, 16010–16017 CrossRef CAS.
- S. Futaki, T. Suzuki, W. Ohashi, T. Yagami, S. Tanaka, K. Ueda and Y. Sugiura, J. Biol. Chem., 2001, 276, 5836–5840 CrossRef CAS.
- P. A. Wender, D. J. Mitchell, K. Pattabiraman, E. T. Pelkey, L. Steinman and J. B. Rothbard, Proc. Natl. Acad. Sci. U. S. A., 2000, 97, 13003–13008 CrossRef CAS.
- J. P. Richard, K. Melikov, E. Vives, C. Ramos, B. Verbeure, M. J. Gait, L. V. Chernomordik and B. Lebleu, J. Biol. Chem., 2003, 278, 585–590 CrossRef CAS.
- S. Futaki, W. Ohashi, T. Suzuki, M. Niwa, S. Tanaka, K. Ueda, H. Harashima and Y. Sugiura, Bioconjugate Chem., 2001, 12, 1005–1011 CrossRef CAS.
- T. I. Kim, M. Lee and S. W. Kim, Biomaterials, 2010, 31, 1798–1804 CrossRef CAS.
- T. I. Kim, M. Ou, M. Lee and S. W. Kim, Biomaterials, 2009, 30, 658–664 CrossRef CAS.
- C. Rudolph, C. Plank, J. Lausier, U. Schillinger, R. H. Muller and J. Rosenecker, J. Biol. Chem., 2003, 278, 11411–11418 CrossRef CAS.
- Y. W. Won, S. M. Yoon, K. M. Lee and Y. H. Kim, Mol. Ther., 2011, 19, 372–380 CrossRef CAS.
- G. Y. Wu and C. H. Wu, J. Biol. Chem., 1987, 262, 4429–4432 CAS.
- C. W. Pouton, P. Lucas, B. J. Thomas, A. N. Uduehi, D. A. Milroy and S. H. Moss, J. Controlled Release, 1998, 53, 289–299 CrossRef CAS.
- C. Plank, M. X. Tang, A. R. Wolfe and F. C. Szoka, Hum. Gene Ther., 1999, 10, 319–332 CrossRef CAS.
- J. S. Choi, K. Nam, J. Park, J. B. Kim and J. K. Lee, J. Controlled Release, 2004, 99, 445–456 CrossRef CAS.
- R. N. Johnson, R. S. Burke, A. J. Convertine, A. S. Hoffman, P. S. Stayton and S. H. Pun, Biomacromolecules, 2010, 11, 3007–3013 CrossRef CAS.
- R. N. Johnson, D. S. H. Chu, J. Shi, J. G. Schellinger, P. M. Carlson and S. H. Pun, J. Controlled Release, 2011, 155, 303–311 CrossRef CAS.
- J. Shi, R. N. Johnson, J. G. Schellinger, P. M. Carlson and S. H. Pun, Int. J. Pharm., 2012, 427, 113–122 CrossRef CAS.
- J. G. Schellinger, J. Pahang, R. N. Johnson, D. S. H. Chu, D. L. Sellers, D. O. Maris, A. J. Convertine, P. S. Stayton, P. J. Horner and S. H. Pun, Biomaterials, 2013, 34, 2318–2326 CrossRef CAS.
- F. L. Brancia, S. G. Oliver and S. J. Gaskell, Rapid Commun. Mass Spectrom., 2000, 14, 2070–2073 CrossRef CAS.
- A. M. Funhoff, C. F. van Nostrum, M. C. Lok, M. M. Fretz, D. J. A. Crommelin and W. E. Hennink, Bioconjugate Chem., 2004, 15, 1212–1220 CrossRef CAS.
- V. P. Taori, H. Lu and T. M. Reineke, Biomacromolecules, 2011, 12, 2055–2063 CrossRef CAS.
- N. J. Treat, D. Smith, C. W. Teng, J. D. Flores, B. A. Abel, A. W. York, F. Q. Huang and C. L. McCormick, ACS Macro Lett., 2012, 1, 100–104 CrossRef CAS.
- H. Han, S. Nho, A. Lee and J. Kim, Bull. Korean Chem. Soc., 2010, 31, 1527–1534 CrossRef CAS.
- L. A. Tziveleka, A. M. G. Psarra, D. Tsiourvas and C. M. Paleos, J. Controlled Release, 2007, 117, 137–146 CrossRef CAS.
- S. Boeckle, K. von Gersdorff, S. van der Piepen, C. Culmsee, E. Wagner and M. Ogris, J. Gene Med., 2004, 6, 1102–1111 CrossRef CAS.
- S. Hwang, N. Bellocq and M. Davis, Bioconjugate Chem., 2001, 12, 280–290 CrossRef CAS.
- M. E. Davis, S. H. Pun, N. C. Bellocq, T. M. Reineke, S. R. Popielarski, S. Mishra and J. D. Heidel, Curr. Med. Chem., 2004, 11, 179–197 CrossRef CAS.
- T. M. Reineke and M. E. Davis, Bioconjugate Chem., 2003, 14, 247–254 CrossRef CAS.
- T. Hashimoto, T. Kawazu, T. Nagasaki, A. Murakami and T. Yamaoka, Sci. Technol. Adv. Mater., 2012, 13 Search PubMed.
- O. Boussif, F. Lezoualcl'h, M. Zanta, M. Mergny, D. Scherman, B. Demeneix and J.-P. Behr, Proc. Natl. Acad. Sci. U. S. A., 1995, 92, 7297–7301 CrossRef CAS.
- N. D. Sonawane, F. C. Szoka and A. S. Verkman, J. Biol. Chem., 2003, 278, 44826–44831 CrossRef CAS.
Footnote |
† Electronic supplementary information (ESI) available: Included as separate formatted document. See DOI: 10.1039/c3bm60079c |
|
This journal is © The Royal Society of Chemistry 2013 |
Click here to see how this site uses Cookies. View our privacy policy here.