DOI:
10.1039/D3QI00743J
(Research Article)
Inorg. Chem. Front., 2023,
10, 4109-4116
C2O42−-templated cage-shaped Ln28 (Ln = Gd, Eu) nanoclusters with magnetocaloric effect and luminescence†
Received
24th April 2023
, Accepted 7th June 2023
First published on 8th June 2023
Abstract
The assembly of high-nuclearity lanthanide nanoclusters with favourable magnetocaloric effects suffers from a significant challenge. Herein, two outstanding examples of cage-shaped nanoclusters, namely, [Ln28(IN)25(C2O4)6(HCOO)(μ3-OH)36(μ2-OH)2(H2O)36]·8Br·xH2O (Ln = Gd and Eu; x = 21, abbreviated as Gd28; x = 17, abbreviated as Eu28, HIN = isonicotinic acid; H2C2O4 = oxalic acid) have been triumphantly designed and constructed under hydrothermal conditions. The crystallographic study revealed that the aesthetically pleasing triangle-shaped Gd12 subunit and Gd16 subunit built separately from four Gd3 units and four Gd4 units, respectively, are shaped into an unprecedented cage-shaped configuration. Prominently, six small and simple C2O42− anions generated via the in situ reaction of HIN are faultlessly anchored in the cage-shaped framework. Furthermore, the existence of abundant GdIII ions impart Gd28 with a captivating magnetic entropy change (−ΔSmaxm = 37.5 J kg−1 K−1) at 2.0 K for ΔH = 7.0 T.
Introduction
Owing to severe pollution of the environment and deficiencies from energy shortages, research on lanthanide nanoclusters with beneficial magnetocaloric effects (MCEs) has been heralded in materials chemistry and magnetochemistry.1–7 The MCE, which explains the phenomenon of heat absorption or heat release of a magnetic material in a changing magnetic field, was first discovered in 1881 by Warburg.8,9 In particular, magnetic cooling materials at ultra-low temperatures play an indispensable role in the development of quantum computers.7,10–12 As a unique class of energy-efficient and environmentally friendly refrigerants at ultra-low temperatures, lanthanide nanoclusters, especially high-nuclearity GdIII oxide/hydroxide clusters, are prospective materials featuring decent MCEs.13–15 At the same time, aesthetically captivating configurations with various conformations and shapes have been designed, such as chair, boat, cage, disk, wheel, nanocapsule, etc.16–21
However, due to practical obstacles in their sizeable ionic radius and high coordination numbers, as well as the mutual repulsion between lanthanide ions, the assembly of high-nuclearity lanthanide nanoclusters is a great challenge, especially when the number of the lanthanide ions is greater than 20.15,22,23 It is well known that in situ reactions can benefit the formation of anionic templates through decomposition, rearrangement and other unpredictable side reactions of solvents or ligands.24 Once an in situ reaction occurs, the decomposition of ligands can effectively realize the slow release of anions. The decomposition process is pretty slow, thus avoiding the formation of precipitation caused by the direct introduction of raw materials, which makes the sophisticated coordination mode possible.25,26 For example, the synthesis of classical cage-like Ln60 is through the in situ reaction of 2,2′-bipyridine-4,4′-dicarboxylic acid, where the small and simple carbonate anion is resoundingly encapsulated in the nanocluster. The synthesis of stable and compact inorganic–organic hybrid Gd4 with twisted cubic [Gd4O4] units is bridged by in situ generated oxalate and sulfate.27,28 So far, it has been observed that ligands containing carboxylic acid, an N-donor or sulfur tend to react in situ.29,30 Indeed, pyridinecarboxylate acids, such as isonicotinic acid, may be converted into some small ligands via an in situ reaction to form into exquisite frameworks with large MCEs. As a result, the first mixed-ligand (oxalate and isonicotinic acid) coordination polymer [Zn2(IN)2(oxa)] was afforded under hydrothermal conditions from the in situ reaction of HIN to oxalate. Subsequently, a 3D compound of [La2(C2O4)2] was reported under hydrothermal conditions from the in situ reaction of HIN.31,32 Although HIN can be chemically rearranged to oxalate under appropriate conditions, it has never been observed in high-nuclearity lanthanide nanoclusters.
Based on the high-nuclearity lanthanide nanoclusters of Ln26 (Ln = Ho and Er) and Ho48 by employing isonicotinic acid as the ligand, we elaborately designed and isolated two unprecedented cage-shaped nanoclusters under hydrothermal conditions with the formulas of [Gd28(IN)25(C2O4)6(HCOO)(μ3-OH)36(μ2-OH)2(H2O)36]·8Br·21H2O and [Eu28(IN)25(C2O4)6(HCOO)(μ3-OH)36(μ2-OH)2(H2O)36]·8Br·17H2O.20,33 Structural analysis showed that the aesthetically elegant cage-shaped Gd28 can be divided into the charming triangle-shaped Gd12 subunit and the triangle-shaped Gd16 subunit that are constructed from four Gd3 units and four Gd4 units, respectively. Remarkably, the small and simple C2O42− anion generated via the in situ reaction of HIN is splendidly anchored in the cage-shaped framework, which is the first case in the family of high-nuclearity lanthanide nanoclusters. Besides, magnetocaloric analysis showed that Gd28 exhibited a considerable value of −ΔSmaxm = 37.5 J kg−1 K−1 at 2.0 K for ΔH = 7.0 T.
Experimental section
Materials and physical measurements
Chemical raw solvents and reagents from commercial sources were available for use without further purification. A PerkinElmer 2400 analyzer (USA) was used for the elemental analyses of C, H, and N. A Bruker D8 diffractometer (Germany) was used to collect powder X-ray diffraction (PXRD) data using Cu Kα radiation (λ = 1.5418 Å) in the 2θ range of 3–50° at a scanning rate of 3° min−1 at room temperature. IR spectra of the two compounds in KBr pellets were recorded using a Nicolet Impact 410 spectrometer (Thermo Fisher, USA) in the region of 4000–400 cm−1. In order to conduct a thermogravimetric analysis (TGA), a Q50 Thermogravimetric Analyzer (TA Instruments, USA) was used in flowing nitrogen air to record thermogravimetric curves of the two compounds from ambient temperature to 1000 °C at a rate of 10 °C min−1. The energy dispersive spectrometry (EDS) data were acquired with a Hitachi S-4800 (Japan) emission scanning electron microscope with a 20 kV accelerating voltage. Magnetization measurements were performed using an MPMS-XL7 SQUID magnetometer (Quantum Design, USA) at 1.8–300 K and in fields of 0–7 T. The solid-state luminescence properties of Eu28 were measured using an FLS1000 spectrophotometer (Edinburgh Instruments, UK) at room temperature.
X-ray crystallography
Under an optical microscope, single crystals of Gd28 with good crystal quality were selected and coated quickly with epoxy glue in air, and placed on a thin glass fiber for data collection; the crystal data of Eu28 were not good enough to be reported in detail. Single crystal X-ray diffraction (SCXRD) data of Gd28 were collected by employing a Bruker Apex II CCD (Germany) with a sealed-tube X-ray source in the ω–2θ scanning method at room temperature. The SHELX software package was used to resolve the crystal structures with direct methods and refine the crystal structures via full-matrix least-squares approaches. Besides, all the non-hydrogen atoms were refined by using anisotropic thermal parameters according to experience. The H atoms of water were not located. The SQUEEZE command was used to remove the H2O molecules. Table 1 provides the relevant crystal data. Table S5† shows the selected bond lengths and angles.
Table 1 Crystal data and structure refinements for Gd28
Compound |
Gd28
|
R
1 = ∑||Fo| − |Fc||/∑|Fo|.
wR2 = ∑[w(Fo2 − Fc2)2]/∑[w(Fo2)2]1/2.
|
Formula |
C163H253Gd28N25O171Br8 |
Formula weight |
10 341 |
T (K) |
296(2) |
Crystal system |
Triclinic |
Space group |
P![[1 with combining macron]](https://www.rsc.org/images/entities/char_0031_0304.gif) |
a (Å) |
21.465(5) |
b (Å) |
24.112(5) |
c (Å) |
30.492(6) |
α (°) |
87.190(3) |
β (°) |
79.559(3) |
γ (°) |
88.080(3) |
V (Å3) |
15 496(6) |
Z
|
2 |
D
c (mg m−3) |
2.216 |
μ (mm−1) |
7.027 |
F (000) |
9692 |
θ range (°) |
0.680–27.322 |
Limiting indices |
−26 ≤ h ≤ 27, −30 ≤ k ≤ 29, −38 ≤ l ≤ 36 |
Reflections collected |
123 261 |
R(int) |
0.0611 |
Data/restraints/parameters |
61 613/852/3297 |
GOF |
1.013 |
R
1
, wR2b [I > 2σ(I)] |
R
1 = 0.0496, wR2 = 0.1353 |
R
1, wR2 (all data) |
R
1 = 0.1085, wR2 = 0.1586 |
Synthesis of Gd28
The reactants of HIN (0.25 g, 2.00 mmol), Gd2O3 (0.20 g, 0.55 mmol), Al2O3 (0.04 g, 0.39 mmol) and KBr (0.01 g, 0.08 mmol) were placed in a 25 mL Teflon-lined autoclave, to which were added HCOOH (0.04 g, 0.87 mmol) and 8 mL of distilled water. Immediately after, the mixture was magnetically stirred at room temperature for 12 hours, and then the pH was adjusted from 4.8 to 2.0 with HNO3 (50%) under stirring conditions. Subsequently, the mixture was maintained at 170 °C for 7 days. After cooling to about room temperature, light-yellow quadrilateral crystals were harvested (as shown in Fig. S1†), which were washed with distilled water (37.0% yield based on Gd). Anal. calcd (%) for C163H253Gd28N25O171Br8 (formula weight = 10
341): C, 18.93; H, 2.45; N, 3.39. Found: C, 18.90; H, 2.41; N, 3.40.
Synthesis of Eu28
The synthesis method is the same as the method used for Gd28 except for Gd2O3 being replaced with Eu2O3. The light-yellow quadrilateral crystals were obtained (as shown in Fig. S1†) (35% yield based on Eu). Anal. calcd (%) for C163H245Eu28N25O167Br8 (formula weight = 10
269): C, 19.07; H, 2.40; N, 3.41. Found: C, 19.09; H, 2.37; N, 3.40.
Results and discussion
Synthetic description
The nearly light-yellow quadrilateral crystals of Gd28 were synthesized using the hydrothermal reaction of Gd2O3, Al2O3, HIN and KBr in the presence of HCOOH at 170 °C for seven days. Notably, the reagent isonicotinic acid with both an O-donor and an N-donor on opposite sides is exceptionally significant in the self-assembly of the Gd28 nanocluster. Furthermore, the target product could not be captured when we tried to add oxalic acid directly to the reaction. Due to the slow production of C2O42− anions in the in situ reaction of isonicotinic acid, the environment can self-adjust to realize the balance between the GdIII ions, C2O42− anions and HIN ligands, resulting in the assembly of the Gd28 nanocluster in the method of slow crystallization.15 According to the literature, formic acid (HCOOH), as the smallest carboxylate ligand, has been favourably used to prepare lanthanide clusters with great MCEs. Gd2O3 is a reactant which not only can slowly release GdIII ions but also regulates the pH of the reaction system. Although AlIII ions are not involved in the final construction, we cannot obtain the target product without them. We replaced Al2O3 with other metallic oxides, such as Fe2O3 or Cr2O3, but we did not get the target crystal. At the same time, we tried using Eu2O3, Tb2O3 or Dy2O3 instead of Gd2O3; only from the environment of Eu2O3 were light-yellow quadrilateral crystals of Eu28 isolated, but unfortunately, the crystal data were not good enough to be reported in detail. On the other hand, the synthetic process of high-nuclearity Ln-exclusive nanoclusters is problematic and heavily relies on certain reaction factors, such as reaction conditions (duration, temperature, pH, cooling rate) and reactants (solvents, ligands, and the kinds of anions). For Gd28, a temperature of 170 °C plays a remarkable role in the formation of the framework; no crystals can be obtained at 160 °C or 140 °C. Most importantly, an absolutely acidic environment (pH = 2) is quite prominent in the self-assembly of the structure, where no crystals can be obtained at pH = 3, pH = 4 or pH = 5. The high temperature and acidic conditions make it possible for the slow release or production of C2O42− ions from HIN.
Crystal structure
Counterions were confirmed via EDS analyses and charge balance studies, while the guest molecules were determined by elemental analysis, and TG and IR measurements. Data from SCXRD analysis revealed that Gd28 crystallized in a triclinic crystal system, with P
space group. As shown in Fig. 1a, the isolated nanocluster with formula [Gd28(IN)25(C2O4)6(HCOO)(μ3-OH)36(μ2-OH)2(H2O)36] can be viewed as being constructed from a 28-metal nanocage of [Gd28(C2O4)6(μ3-OH)36(μ2-OH)2]34+ protected and stabilized by 25 IN− groups, one HCOO− group and about 36 guest water molecules. Broadly, six small and simple C2O42− anions generated via the in situ reaction of HIN are splendidly anchored in the cage-shaped metal–organic framework to balance the high positive charges and stabilize its construction (Fig. 1b).
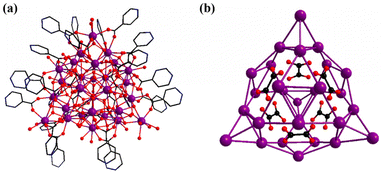 |
| Fig. 1 (a) Plot of the whole molecular structure and (b) the C2O42− anion template in the metal skeleton of Gd28. Purple, Ln; blue, N; red, O; and black, C. | |
For the sake of facilitating the description and understanding of the structural skeleton, the cage-like cationic core of Gd28 can be subdivided into two different types of primary units, i.e., a triangular Gd3 unit formulated as [Gd3(μ3-OH)2]7+ and a cubane-like Gd4 unit formulated as [Gd4(μ3-OH)8]4+. A Gd3-type unit, as shown in Fig. 2a, can be considered a triangular construction connected by two μ3-OH groups and four Gd3 units are linked by six C2O42− anions and formed into a [Gd12(μ3-OH)8(C2O4)6]16+ (Gd12) subunit. For clarity, each of the Gd3 units, with a shell-shaped construction, is combined with six C2O42− anions, and formed into a delicate triangle-like structure (Fig. 2d). A Gd4-typeunit is a cubane-like configuration formed by the banding of four μ3-OH groups, which is extremely common in other lanthanide clusters (Fig. 2c). Four Gd4 units are combined with the same six C2O42− anions forming a [Gd16(μ3-OH)16(C2O4)6]20+ (Gd16) subunit. For clarity, each tetrahedron is connected by six C2O42− anions forming a fancy triangle-shaped framework (Fig. 2e). As shown in Fig. 2f, the frog-shaped Gd12 subunit and the frog-shaped Gd16 subunit are bonded together into an individual cage-shaped framework templated by six C2O42− anions. The cage-like nanocluster is further stabilized by the 25 IN− groups and one HCOO− group. Additionally, the IN− ligands in Gd28 display three different types of coordination modes, μ2-η1:η0:η1, μ1-η0:η0:η1 and μ1-η1:η0:η1 (Fig. 3a), and the six C2O42− anions exhibit only one coordination mode, μ6-η2:η2:η2:η2 (Fig. 3b).
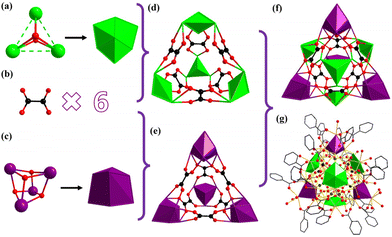 |
| Fig. 2 Ball-and-stick views of (a) the triangular [Gd3(μ3-OH)2]7+ unit; (b) the C2O42− anion; (c) the cubane-like [Gd4(μ3-OH)4]8+ unit; (d) the [Gd12(μ3-OH)8(C2O4)6]16+ subunit; (e) the [Gd16(μ3-OH)16(C2O4)6]20+ subunit; (f) the [Gd28(C2O4)6(μ3-OH)36(μ2-OH)2]34+ nanocluster; and (g) the subunits representing Gd28. | |
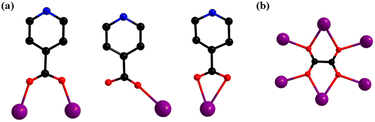 |
| Fig. 3 Coordination modes of (a) IN− ligands and (b) C2O42− in Gd28. | |
The metal framework of Gd28 is shown in Fig. 1b; four tetrahedra (16 GdIII) and four triangles (12 GdIII) were joined together to complete the nano-caged framework. All GdIII ions are located in eight-coordinated or nine-coordinated environments with different geometries (Fig. S5†). Gd3, Gd5, Gd6, Gd8, Gd11, Gd12, Gd14, Gd16, Gd25, Gd26, Gd27 and Gd28 represent eight coordination modes in the [GdO8] polyhedra; Gd1, Gd2, Gd4, Gd7, Gd9, Gd10, Gd13, Gd15, Gd17, Gd18, Gd19, Gd20, Gd21, Gd22, Gd23 and Gd24 display nine coordination modes in the [GdO9] polyhedra. As shown in Fig. S5,† 28 GdIII ions have different coordination environments. For example, Gd1 is coordinated with one HIN, one C2O42− anion, four μ3-OH groups and two coordinated water molecules. According to the continuous shape measurement (CShM) analysis, the 28 GdIII ions have seven different configurations. For instance, Gd28 exhibits a twisted octa-coordinated square antiprism, while Gd10 shows a distorted nine-coordinated spherical capped square antiprism (Tables S1 and 2†). The bond lengths between two neighboring ions (Gd–O = 2.3–2.9 Å) and the bond angles between the neighboring ions (O–Gd–O = 58.8–155.3 Å) are equivalent to the previously reported Gd-based nanocluster (Table S5†).
Remarkably, cage-shaped high-nuclearity Gd-based nanoscale clusters, where the number of lanthanide ions is more than 20, are still underdeveloped. To date, only cage-shaped Gd20, Gd26, Gd27, Gd32, Gd38, Gd50, Gd60 and Gd104 nanoclusters have been reported.18,34–37 The Gd28 nanocluster reported in this work is extraordinarily different from other reported cage-shaped nanoclusters. For example, the spherical Gd26 nanocluster consists of five cubane-like Gd4 units and two triangular Gd3 units templated by nine CO32− anions. Nicotinic acid is the organic linker and the protective ligand in the formation of Gd26.35 Other cage-shaped Gd27 nanoclusters have been isolated by employing sodium propionate as the ligand. The metal skeleton of Gd27 is achieved by five tetrahedral Gd4 units and one crown-shaped Gd7 unit templated by eight CO32− anions.18 Explicitly, the Gd28 nanocluster exhibits an unprecedented configuration in the family of high-nuclearity cage-shaped nanoclusters.
It is worth noting that the structure of the Eu28 nanocluster is roughly the same as that of Gd28, according to the main characteristic diffraction peaks of the PXRD (Fig. 4). Meanwhile, the free H2O molecules of the two nanoclusters were determined by TG analysis, as shown in Fig. S9 and 10.† In the temperature range of 25–265 °C, the weight loss of 3.67% (calcd 3.66%) was attributed to the loss of 21 free water molecules for Gd28, while the weight loss of 2.96% (calcd 2.98%) was attributed to the loss of 17 free water molecules for Eu28.
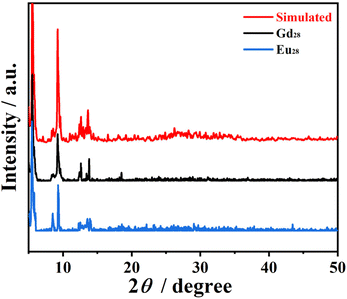 |
| Fig. 4 PXRD patterns of Gd28 and Eu28. | |
Luminescence of Eu28
One of the most meaningful characteristics of lanthanide ions is their picturesque photoluminescence properties derived from f–f intraconfigurational transitions.13 Additionally, EuIII is most commonly used in sensing applications due to its strong visible light emission in the red region.38 Accordingly, the luminescence properties of Eu28 with 28 EuIII ions were measured at room temperature, as shown in Fig. 5. Under UV lamp irradiation, we observed the solid-state luminescence of Eu28. Besides, under maximum excitation at 394 nm, the electronic transitions of EuIII from 5D0 → 7FJ (J = 0, 1, 2, 3 and 4) were observed. The relatively weak emission bands at 581, 653 and 700 nm are attributed to the electronic transitions of 5D0 → 7F0, 5D0 → 7F3 and 5D0 → 7F4, respectively. In comparison, the two sharp lines at 593 and 616 nm are assigned to the dominant electronic transitions of 5D0 → 7F1 and 5D0 → 7F2, respectively. Notably, the absence of a strong and broad peak from the IN− ligand indicates the high energy transfer efficiency to the EuIII center emission, known as the “antenna effect”.39 Furthermore, under the maximum excitation at 394 nm, the luminescence lifetime and the solid-state photoluminescence quantum yield (PLQY) of Eu28 were 309.8 μs and 4.18%, respectively (Fig. 5b). To further learn about the solid-state luminescence of Eu28, we drew a simple Jablonsky level diagram to explain the luminescence properties (Fig. 5c).40–43 These findings are of significant value for understanding the optical properties of the lanthanide clusters and their applications in optoelectronic devices, among others.44
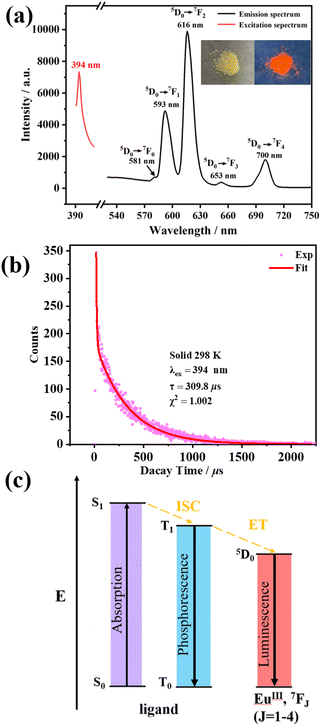 |
| Fig. 5 (a) Excitation and emission spectra; (b) luminescence lifetime; and (c) Jablonsky level diagrams of Eu28. | |
Magnetic properties of Gd28
At an applied direct current (dc) magnetic field of 1.0 kOe from 1.8 K to 300 K, temperature-dependent magnetic susceptibilities of Gd28 were characterized. The observed χMT value (225.0 cm3 K mol−1) at 300 K is slightly larger than the expected value (220.5 cm3 K mol−1) based on 28 independent GdIII ions (S = 7/2, g = 2) with the Lande formula.6 As shown in Fig. 6a, as the temperature drops from 300 K to 1.8 K, the χMT value gradually decreases to a minimum of 94.4 cm3 K mol−1 at 1.8 K.45 The overall trend of χMT vs. T data indicated that Gd28 exhibited antiferromagnetic interaction between these metal cations. At the same time, fitting the χM−1vs. T data with the Curie–Weiss law from 1.8 K to 300 K resulted in the Weiss constant θ = −5.7 K and Curie constant C = 226.5 cm3 K mol−1. All the above discussions demonstrate the existence of weak antiferromagnetic interactions among metal ions (Fig. 6b).46–49
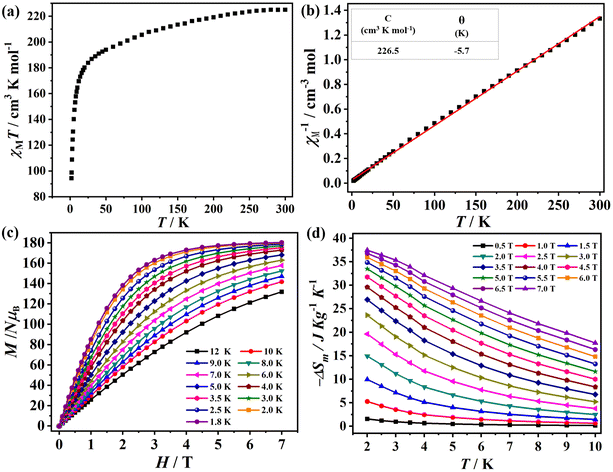 |
| Fig. 6 (a) Plot of experimental magnetic susceptibility (χMT) versus T; (b) χM−1versus T plot; (c) plot of M–H under different temperatures; and (d) plot of experimental magnetic entropy change (−ΔSm) versus T for Gd28. | |
A large number of GdIII ions and fairly weak magnetic interactions between GdIII ions make Gd28 nanoclusters a valid class of materials for magnetic cooling applications. Hence, it encouraged us to conduct research of the magnetocaloric effect of Gd28. Here, the magnetization (M) and magnetic field (H) at low temperature (1.8 K–10 K) was measured (Fig. 6c). With the increase of magnetic field (H), the magnetization (M) also increased steadily to the maximum of 180.3Nβ under 1.8 K and 7 T; the discrepancy between the two magnetization values (calculated value is 196.0Nβ based on 28 uncorrelated metal ions) resulted from the weak antiferromagnetic interaction in the nanocluster. Subsequently, we investigated the magnetocaloric effects of Gd28 by employing the Maxwell equation of
(Fig. 6d).50 With an increased H and a reduced T, the experimental entropy changes gradually increased, and the −ΔSmaxm is 37.5 J kg−1 K−1 for ΔH = 7 T at 2 K. By using the equation of −ΔSm = nR
ln(2S + 1), the 28 uncorrelated GdIII ions were calculated and resulted in a theoretical value of 46.8 J kg−1 K−1; the discrepancy between the two ΔSm values might be due to the existence of antiferromagnetic exchanges among the metal centers.51 As far as we know, the Gd28 nanocluster exhibits a decent magnetocaloric effect value, comparable to those of the reported high-nuclearity nanoscale clusters Gd27 (−ΔSm = 41.8 J kg−1 K−1), Gd38 (−ΔSm = 37.9 J kg−1 K−1) and Gd36 (−ΔSm = 39.7 J kg−1 K−1), validating its excellent potential application in magnetic cooling (Table S3†).18,36,52
Conclusions
In summary, we triumphantly obtained two novel high-nuclearity lanthanide nanoclusters Gd28 and Eu28 in the presence of HIN under hydrothermal conditions. Subsequently, through structural characterization studies, we observed that the charming triangle-shaped Gd12 subunit and Gd16 subunit assembled by four Gd3 units and four Gd4 units, respectively, were arranged into an unprecedented cage-shaped structure featuring six C2O42− anions. Notably, this is the first case of an in situ hydrolysis of isonicotinic acid to C2O42− ions in constructing high-nuclearity lanthanide nanoclusters. Magnetization analysis revealed that Gd28 exhibits a decent magnetocaloric effect of 37.5 J kg−1 K−1 at 2.0 K for ΔH = 7.0 T. This paper has given a new idea for introducing C2O42− from the in situ reaction of HIN to assemble high-nuclearity lanthanide clusters with large magnetic entropy changes. Next, we will continue to isolate molecular magnetocaloric materials based on Gd nanoclusters through the in situ hydrolysis of HIN and probe more excellent properties and potential applications for high-nuclearity lanthanide clusters.
Conflicts of interest
The authors declare no competing financial interest.
Acknowledgements
This work was supported by the Natural Science Foundation of China (Grant 92161109).
References
- W. P. Chen, P. Q. Liao, Y. Yu, Z. Zheng, X. M. Chen and Y. Z. Zheng, A Mixed-Ligand Approach for a Gigantic and Hollow Heterometallic Cage {Ni64RE96} for Gas Separation and Magnetic Cooling Applications, Angew. Chem., Int. Ed., 2016, 55, 9375–9379 CrossRef CAS PubMed.
- N. F. Li, Q. F. Lin, Y. M. Han, Z. Y. Du and Y. Xu, The chain-shaped coordination polymers based on the bowl-like Ln18Ni24(23.5) clusters exhibiting favorable low-field magnetocaloric effect, Chin. Chem. Lett., 2021, 32, 3803–3806 CrossRef CAS.
- N. F. Li, Q. F. Lin, X. M. Luo, J. P. Cao and Y. Xu, Cl−-Templated Assembly of Novel Peanut-like Ln40Ni44 Heterometallic Clusters Exhibiting a Large Magnetocaloric Effect, Inorg. Chem., 2019, 58, 10883–10889 CrossRef CAS PubMed.
- E. Moreno Pineda, F. Tuna, R. G. Pritchard, A. C. Regan, R. E. Winpenny, E. J. McInnes and Y. Z. Zheng, Molecular amino-phosphonate cobalt-lanthanide clusters, Chem. Commun., 2013, 49, 3522–3524 RSC.
- J. B. Peng, Q. C. Zhang, X. J. Kong, Y. Z. Zheng, Y. P. Ren, L. S. Long, R. B. Huang, L. S. Zheng and Z. Zheng, High-nuclearity 3d-4f clusters as enhanced magnetic coolers and molecular magnets, J. Am. Chem. Soc., 2012, 134, 3314–3317 CrossRef CAS PubMed.
- Z. X. Lu, Z. Zhuo, W. Wang, Y. G. Huang and M. C. Hong, {Gd44Ni22}: a gigantic 3d–4f wheel-like nanoscale cluster with a large magnetocaloric effect, Inorg. Chem. Front., 2023, 10, 979–983 RSC.
- J. L. Wang, M. X. Yang, N. F. Li, X. M. Liu, J. N. Li, Q. D. Ping and Y. Xu, Two Ni-Substituted Trilacunary Keggin-Type Polyoxometalates: Syntheses, Crystal Structures, NLO Studies, and Magnetic Properties, Inorg. Chem., 2021, 60, 13748–13755 CrossRef CAS PubMed.
- E. Warburg, Magnetische Untersuchungen, Ann. Phys., 1881, 249, 141–164 CrossRef.
- N. F. Li, Q. Wang, J. N. Li, Y. T. Yu and Y. Xu, Two SiO44−-Templated Ln23Ni20 Clusters with Magnetic Cooling and Stability, Inorg. Chem., 2022, 61, 7180–7187 CrossRef CAS PubMed.
- Y. Zheng, Q. C. Zhang, L. S. Long, R. B. Huang, A. Muller, J. Schnack, L. S. Zheng and Z. Zheng, Molybdate templated assembly of Ln12Mo4-type clusters (Ln = Sm, Eu, Gd) containing a truncated tetrahedron core, Chem. Commun., 2013, 49, 36–38 RSC.
- Y. Z. Zheng, E. M. Pineda, M. Helliwell and R. E. Winpenny, MnII-GdIII phosphonate cages with a large magnetocaloric effect, Chem. – Eur. J., 2012, 18, 4161–4165 CrossRef CAS PubMed.
- J. J. Hu, Y. Peng, S. J. Liu and H. R. Wen, Recent advances in lanthanide coordination polymers and clusters with magnetocaloric effect or single-molecule magnet behavior, Dalton Trans., 2021, 50, 15473–15487 RSC.
- Q. Wang, Y. T. Yu, J. L. Wang, J. N. Li, N. F. Li, X. Fan and Y. Xu, Two Windmill-Shaped Ln18 Nanoclusters Exhibiting High Magnetocaloric Effect and Luminescence, Inorg. Chem., 2023, 62, 3162–3169 CrossRef CAS PubMed.
- J.-L. Liu, Y.-C. Chen, F.-S. Guo and M.-L. Tong, Recent advances in the design of magnetic molecules for use as cryogenic magnetic coolants, Coord. Chem. Rev., 2014, 281, 26–49 CrossRef CAS.
- X. Y. Zheng, X. J. Kong, Z. Zheng, L. S. Long and L. S. Zheng, High-Nuclearity Lanthanide-Containing Clusters as Potential Molecular Magnetic Coolers, Acc. Chem. Res., 2018, 51, 517–525 CrossRef CAS PubMed.
- J. Hao, L. Geng, J. Zheng, J. Wei, L. Zhang, R. Feng, J. Zhao, Q. Li, J. Pang and X. H. Bu, Ligand Induced Double-Chair Conformation Ln12 Nanoclusters Showing Multifunctional Magnetic and Proton Conductive Properties, Inorg. Chem., 2022, 61, 3690–3696 CrossRef CAS PubMed.
- J. N. Li, N. F. Li, J. L. Wang, X. M. Liu, Q. D. Ping, T. T. Zang, H. Mei and Y. Xu, A new family of boat-shaped Ln8 clusters exhibiting the magnetocaloric effect and slow magnetic relaxation, Dalton Trans., 2021, 50, 13925–13931 RSC.
- X. Y. Zheng, J. B. Peng, X. J. Kong, L. S. Long and L. S. Zheng, Mixed-anion templated cage-like lanthanide clusters: Gd27 and Dy27, Inorg. Chem. Front., 2016, 3, 320–325 RSC.
- J. M. Peng, H. L. Wang, Z. H. Zhu, J. Bai, F. P. Liang and H. H. Zou, Series of the Largest Dish-Shaped Dysprosium Nanoclusters Formed by In Situ Reactions, Inorg. Chem., 2022, 61, 6094–6100 CrossRef CAS PubMed.
- L. Chen, J. Y. Guo, X. Xu, W. W. Ju, D. Zhang, D. R. Zhu and Y. Xu, A novel 2-D coordination polymer constructed from high-nuclearity waist drum-like pure Ho48 clusters, Chem. Commun., 2013, 49, 9728–9730 RSC.
- L. X. Chang, G. Xiong, L. Wang, P. Cheng and B. Zhao, A 24-Gd nanocapsule with a large magnetocaloric effect, Chem. Commun., 2013, 49, 1055–1057 RSC.
- Z.-R. Luo, H. L. Wang, Z. H. Zhu, T. Liu, X. F. Ma, H. F. Wang, H. H. Zou and F. P. Liang, Assembly of Dy60 and Dy30 cage-shaped nanoclusters, Commun. Chem., 2020, 3, 30–36 CrossRef CAS PubMed.
- W. Q. Lin, X. F. Liao, J. H. Jia, J. D. Leng, J. L. Liu, F. S. Guo and M. L. Tong, Lanthanide oxide clusters: from tetrahedral [Dy4(μ4-O)]10+ to supertetrahedral [Ln20(μ4-O)11]38+ (Ln = Tb, Dy, Ho, Er), Chem. – Eur. J., 2013, 19, 12254–12258 CrossRef CAS PubMed.
- S. J. Liu, S. D. Han, J. P. Zhao, J. Xu and X. H. Bu,
In situ synthesis of molecular magnetorefrigerant materials, Coord. Chem. Rev., 2019, 394, 39–52 CrossRef CAS.
- J. Xu, W. Su and M. Hong, 3D lanthanide–transition-metal–organic frameworks constructed from tetranuclear {Ln4} SBUs and Cu centres with fsc net, CrystEngComm, 2011, 13, 3998–4004 RSC.
- X. Y. Zheng, S. Q. Wang, W. Tang, G. L. Zhuang, X. J. Kong, Y. P. Ren, L. S. Long and L. S. Zheng, Two nanosized 3d-4f clusters featuring four Ln6 octahedra encapsulating a Zn4 tetrahedron, Chem. Commun., 2015, 51, 10687–10690 RSC.
- X. M. Luo, Z. B. Hu, Q. F. Lin, W. Cheng, J. P. Cao, C. H. Cui, H. Mei, Y. Song and Y. Xu, Exploring the Performance Improvement of Magnetocaloric Effect Based Gd-Exclusive Cluster Gd60, J. Am. Chem. Soc., 2018, 140, 11219–11222 CrossRef CAS PubMed.
- S.-D. Han, X. H. Miao, S. J. Liu and X. H. Bu, Magnetocaloric effect and slow magnetic relaxation in two dense (3,12)-connected lanthanide complexes, Inorg. Chem. Front., 2014, 1, 549–552 RSC.
- P. F. Shi, Y. Z. Zheng, X. Q. Zhao, G. Xiong, B. Zhao, F. F. Wan and P. Cheng, 3 D MOFs containing trigonal bipyramidal Ln5 clusters as nodes: large magnetocaloric effect and slow magnetic relaxation behavior, Chem. – Eur. J., 2012, 18, 15086–15091 CrossRef CAS PubMed.
- S. Zhang, E. Duan and P. Cheng, An exceptionally stable 3D GdIII-organic framework for use as a magnetocaloric refrigerant, J. Mater. Chem. A, 2015, 3, 7157–7162 RSC.
- L. Z. Zhang, W. Gu, B. Li, X. Liu and D. Z. Liao, {[Nd4(ox)4(NO3)2(OH)2(H2O)2]·5H2O}n: A Porous 3D Lanthanide-Based Coordination Polymer with a Special Luminescent Property, Inorg. Chem., 2007, 46, 622–624 CrossRef CAS PubMed.
- J. Y. Lu, J. Macias, J. Lu and J. E. Cmaidalka, An Unforeseen Chemical Rearrangement of Pyridine carboxylate to Oxalate under Hydrothermal Conditions Afforded the First Oxalato and Isonicotinato Mixed-Ligand Guest-Inclusion Coordination Polymer, Cryst. Growth Des., 2002, 2, 485–487 CrossRef CAS.
- L. Chen, L. Huang, C. Wang, J. Fu, D. Zhang, D. Zhu and Y. Xu, Hydrothermal synthesis, structure, and properties of two new nanosized Ln26 (Ln=Ho, Er) clusters, J. Coord. Chem., 2012, 65, 958–968 CrossRef CAS.
- L. Qin, G. J. Zhou, Y. Z. Yu, H. Nojiri, C. Schroder, R. E. P. Winpenny and Y. Z. Zheng, Topological Self-Assembly of Highly Symmetric Lanthanide Clusters: A Magnetic Study of Exchange-Coupling “Fingerprints” in Giant Gadolinium(III) Cages, J. Am. Chem. Soc., 2017, 139, 16405–16411 CrossRef CAS PubMed.
- R. Sen, D. K. Hazra, M. Mukherjee and S. Koner, Gd26 Cluster Consisting of Distorted Cubane Cores: Synthesis, Structure and Heterogeneous Catalytic Epoxidation of Olefins, Eur. J. Inorg. Chem., 2011, 2011, 2826–2831 CrossRef CAS.
- F. S. Guo, Y. C. Chen, L. L. Mao, W. Q. Lin, J. D. Leng, R. Tarasenko, M. Orendac, J. Prokleska, V. Sechovsky and M. L. Tong, Anion-templated assembly and magnetocaloric properties of a nanoscale {Gd38} cage versus a {Gd48} barrel, Chem. – Eur. J., 2013, 19, 14876–14885 CrossRef CAS PubMed.
- J. B. Peng, X. J. Kong, Q. C. Zhang, M. Orendac, J. Prokleska, Y. P. Ren, L. S. Long, Z. Zheng and L. S. Zheng, Beauty, symmetry, and magnetocaloric effect-four-shell keplerates with 104 lanthanide atoms, J. Am. Chem. Soc., 2014, 136, 17938–17941 CrossRef CAS PubMed.
- T. Q. Lu, H. Xu, L. T. Cheng, X. T. Wang, C. Chen, L. Cao, G. L. Zhuang, J. Zheng and X. Y. Zheng, Family of Nanoclusters, Ln33 (Ln = Sm/Eu) and Gd32, Exhibiting Magnetocaloric Effects and Fluorescence Sensing for MnO4−, Inorg. Chem., 2022, 61, 8861–8869 CrossRef CAS PubMed.
- Y. L. Li, H. L. Wang, Z. C. Chen, Z. H. Zhu, Y. C. Liu, R. Y. Yang, F. P. Liang and H. H. Zou, Lanthanoid hydrogen-bonded organic frameworks: Enhancement of luminescence by the coordination-promoted antenna effect and applications in heavy-metal ion sensing and sterilization, Chem. Eng. J., 2023, 451, 138880 CrossRef CAS.
- H. F. Wang, X. F. Ma, Z. H. Zhu, H. H. Zou and F. P. Liang, Regulation of the Metal Center and Coordinating Anion of Mononuclear Ln(III) Complexes to Promote an Efficient Luminescence Response to Various Organic Solvents, Langmuir, 2020, 36, 1409–1417 CrossRef CAS PubMed.
- H. F. Wang, Z. H. Zhu, J. M. Peng, B. Yin, H. L. Wang, H. H. Zou and F. P. Liang, Multifunctional Binuclear Ln(III) Complexes Obtained via In Situ Tandem Reactions: Multiple Photoresponses to Volatile Organic Solvents and Anticounterfeiting and Magnetic Properties, Inorg. Chem., 2020, 59, 13774–13783 CrossRef CAS PubMed.
- J. Zhou, H. Li, H. Zhang, H. Li, W. Shi and P. Cheng, A Bimetallic Lanthanide Metal-Organic Material as a Self-Calibrating Color-Gradient Luminescent Sensor, Adv. Mater., 2015, 27, 7072–7077 CrossRef CAS PubMed.
- S. S. Sun, Z. Wang, X. W. Wu, J. H. Zhang, C. J. Li, S. Y. Yin, L. Chen, M. Pan and C. Y. Su, ESIPT-Modulated Emission of Lanthanide Complexes: Different Energy-Transfer Pathways and Multiple Responses, Chem. – Eur. J., 2018, 24, 10091–10098 CrossRef CAS PubMed.
- P.-Da Liu, Ao.-G. Liu, P.-M. Wang, Y. Chen and B. Li, Smart crystalline frameworks constructed with bisquinoxaline-based component for multi-stimulus luminescent sensing materials, Chin. J. Struct. Chem., 2023, 42, 100001 CrossRef.
- W. P. Chen, P. Q. Liao, P. B. Jin, L. Zhang, B. K. Ling, S. C. Wang, Y. T. Chan, X. M. Chen and Y. Z. Zheng, The Gigantic {Ni36Gd102} Hexagon: A Sulfate-Templated “Star-of-David” for Photocatalytic CO2 Reduction and Magnetic Cooling, J. Am. Chem. Soc., 2020, 142, 4663–4670 CrossRef CAS PubMed.
- F. Shao, J. J. Zhuang, M. G. Chen, N. Wang, H. Y. Shi, J. P. Tong, G. Luo, J. Tao and L. S. Zheng, Facile and environmentally friendly synthesis of six heterometallic dumbbell-shaped MLn (M = Co, Ni; Ln = Eu, Gd, Dy) clusters as cryogenic magnetic coolants and molecular magnets, Dalton Trans., 2018, 47, 16850–16854 RSC.
- M. H. Du, D. H. Wang, L. W. Wu, L. P. Jiang, J. P. Li, L. S. Long, L. S. Zheng and X. J. Kong, Hierarchical Assembly of Coordination Macromolecules with Atypical Geometries: Gd44Co28 Crown and Gd95Co60 Cage, Angew. Chem., Int. Ed., 2022, 61, e202200537 CAS.
- N. F. Li, Y. M. Han, J. N. Li, Q. Chen and Y. Xu, Efficiently increasing low-field magnetic entropy by incorporating SO32− into Gd22Ni21 clusters, Dalton Trans., 2022, 51, 2669–2673 RSC.
- P. Hu, S. Li, L. Cao, A. Liu, G. L. Zhuang, L. Ji and B. Li, Construction of a High Nuclear Gadolinium Cluster with Enhanced Magnetocaloric Effect through Structural Transition, ACS Omega, 2022, 7, 38782–38788 CrossRef CAS PubMed.
- N. F. Li, X. M. Luo, J. Wang, J. L. Wang, H. Mei, Y. Song and Y. Xu, Largest 3d-4f 196-nuclear Gd158Co38 clusters with excellent magnetic cooling, Sci. China: Chem., 2022, 65, 1577–1583 CrossRef CAS.
- R. Chen, C. L. Chen, M. H. Du, X. Wang, C. Wang, L. S. Long, X. J. Kong and L. S. Zheng, Soluble lanthanide-transition-metal clusters Ln36Co12 as effective molecular electrocatalysts for water oxidation, Chem. Commun., 2021, 57, 3611–3614 RSC.
- M. Y. Wu, F. L. Jiang, X. J. Kong, D. Q. Yuan, L. S. Long, S. A. Al-Thabaiti and M. C. Hong, Two polymeric 36-metal pure lanthanide nanosize clusters, Chem. Sci., 2013, 4, 3104–3109 RSC.
|
This journal is © the Partner Organisations 2023 |