Primary amine recognition in water by a calix[6]aza-cryptand incorporated in dodecylphosphocholine micelles†
Received
27th November 2014
, Accepted 6th January 2015
First published on 6th January 2015
Abstract
Water is a unique solvent and the design of selective artificial hosts that can efficiently work in an aqueous medium is a challenging task. It is known that the calix[6]tren zinc complex can recognize neutral guests in organic solvents. This complex was incorporated into dodecylphosphocholine micelles (DPC) and studied by NMR. The incorporated complex is able to extract selectively primary amines from the aqueous environment driven by an important hydrophobic effect which also affects the selectivity of the complex for these amines. This work shows how the incorporation of organo-soluble receptors in micelles can be an elegant and very efficient strategy to obtain water compatible nanosized supramolecular recognition devices which can be prepared via a straightforward self-assembly process.
Introduction
The design of artificial receptors that can selectively bind, with a high affinity, charged or neutral species is one of the major objectives of supramolecular chemistry.1 Indeed, such receptors have potential applications in the sensing of chemical species in the fields of biological and environmental analysis.2 The development of such receptors is nevertheless challenging because water is a very competitive solvent.3 Exploiting the hydrophobic effect which can drive apolar guests into apolar cavities is an interesting strategy. In this context, many examples of neutral guest complexation have been reported with water-soluble cavitands having a hydrophobic inner space such as cyclodextrins4 and cucurbiturils.5 These macrocycles however remain difficult to modify selectively6 which limits the design of sophisticated receptors. In the case of the other families of cavitands such as calixarenes, fastidious syntheses are often required in order to make them water-soluble.
Micelles are extensively used in a wide variety of industrial and recovery processes to incorporate lipophilic compounds7 and are thus a possible solution to overcome the solubility problem of organic receptors in water. This strategy is interesting, as no synthesis needs to be undertaken in order to introduce hydrophilic groups on the receptor. A few examples of receptors and sensors incorporated in micelles have been reported in the literature8 and in some cases their binding properties have been investigated and shown to be enhanced by the micellar environment. Rebek and co-workers have, for instance, observed that a conformational reorganization of a resorcinarene cavitand incorporated into dodecylphosphocholine (DPC) micelles increases significantly its binding capacity towards different guests.9 It has also been reported that uranyl-salophen receptors incorporated into cetyltrimethylammonium bromide (CTABr) micelles have an affinity for fluoride which is two order of magnitude larger than the affinity of the related water-soluble receptor.10,11
Calix[6]arenes are a well-known class of concave macrocyclic compounds presenting a cavity well adapted for the inclusion of organic guests and which can be selectively modified.12,13 Depending on their functionalization, they have revealed to be efficient receptors for a variety of neutral or charged species. In this context, different generations of biomimetic receptors based on calix[6]arene ligands bearing nitrogenous coordinating arms on the narrow rim have been developed.14 In particular, the calix[6]tren receptor 1,15 belonging to the third generation has been shown to display unique and versatile binding properties, notably toward metal ions and neutral guests (Fig. 1).16 Indeed, the tren cap offers a strong binding site for a metal ion (e.g. Zn2+, Cun+). Once coordinated, the metal ion presents a single coordination site accessible for an exogenous neutral guest, which is fully controlled by the hydrophobic funnel. Hence, complex 1·Zn2+ (Fig. 1) can bind with a high selectivity various neutral organic molecules (e.g. amines, alcohols, amides, etc.) inside the calix[6]arene core in different organic solvents.17
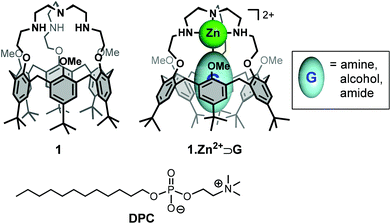 |
| Fig. 1 Structures of ligand 1, complex 1·Zn2+⊃G and DPC surfactant. | |
With the aim of developing selective molecular receptors that exhibit high affinity for specific guests in water, we were interested in evaluating the binding properties of complex 1·Zn2+ in an aqueous medium. In order to transfer this lipophilic metal complex into water, we thus envisioned its incorporation into micelles. Herein, we report the incorporation of calix[6]tren 1 and of its corresponding metal complex 1·Zn2+ into DPC micelles (Fig. 1). Characterization of the calix[6]arene/micelle systems and evaluation of the binding properties of the incorporated receptors were achieved by NMR spectroscopy.
Results and discussion
Incorporation of complex 1·Zn2+ and ligand 1 into DPC micelles.
The receptor 1·Zn2+ was successfully incorporated into DPC micelles by simple mixing of the receptor and the surfactant (20 mM) in D2O at neutral pH (∼7.6) and concentrations of up to 0.5 mM of 1·Zn2+ could be obtained.18 The 1H NMR spectrum of the incorporated receptor 1·Zn2+ in DPC-d38 micelles shows a NMR pattern which is very similar to the one recorded in CDCl3 (Fig. 2a vs. 2b). No significant changes were observed in the 1H NMR spectrum between 283 K and 330 K. As shown previously, the complexity of the NMR profile arises from the heterochirality of the three nitrogen stereocenters which leads to a nonsymmetrical C1 calixtren-Zn complex.17 Similarly to 1·Zn2+ in CDCl3, the downfield shift of the OMe signals of the incorporated complex (δOMe > 3.9 ppm in CDCl3 and in D2O)19 suggests that these groups have been expelled from the cavity by a coordinated solvent water molecule.20 Two very distinct sets of signals can be observed for the tBu as well as for the ArH protons, indicating that the calixarene adopts a major flattened cone conformation. The incorporation of 1·Zn2+ inside the DPC micelles was unambiguously confirmed by DOSY NMR experiments as both receptor and micelle exhibit the same diffusion coefficient, corresponding to a hydrodynamic radius of ∼2 nm.21 Moreover, knowing that the micellar aggregation number is around 50,22 it is reasonable to estimate that there is 1 molecule of complex 1·Zn2+ per micelle.
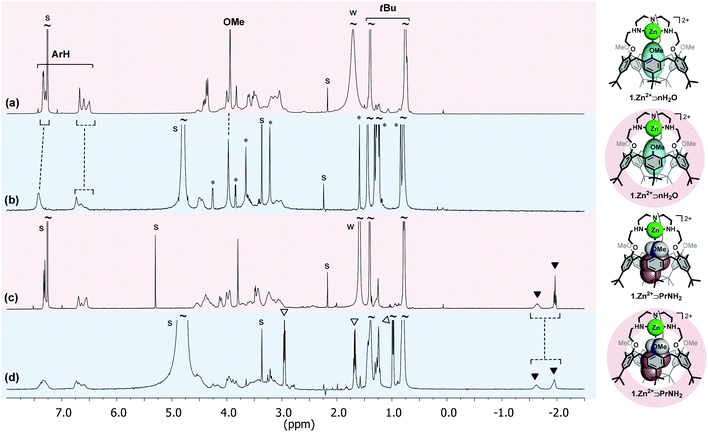 |
| Fig. 2
1H NMR spectra (298 K) of (a) 1·Zn2+ in CDCl3 (600 MHz); (b) 1·Zn2+ in DPC-d38 (20 mM in D2O at pH ∼7.6, 600 MHz); (c) 1·Zn2+ in CDCl3 after the addition of ∼1 equiv. of PrNH2 (400 MHz); (d) 1·Zn2+ in DPC-d38 (20 mM in D2O at pH ∼8.6) after the addition of 5 equiv. of PrNH2 (600 MHz). ▼: PrNH2 in; ▽: PrNH2 out; *: residual DPC; s: solvent; w: water. | |
In order to gain information about the position of 1·Zn2+ in the micelle, paramagnetic relaxation enhancement (PRE) experiments were undertaken.23 It is indeed possible to obtain reliable information on the localization of receptors in micelles by comparing the PRE data obtained for the receptor protons with those for the surfactant protons.10 When plotting the relaxation rate enhancement as a function of paramagnetic species concentration, a linear relationship was obtained and the slope, known as the relaxivity, was extracted. The normalized relaxivities for the aromatic and tBu protons of 1·Zn2+ have values similar to those of the tail of the surfactant but very different from those of the hydrophilic part.21 It was thus possible to conclude that the calixarene positions itself in the hydrophobic core. Interestingly, the spectrum of 1·Zn2+ in DPC micelles was not significantly modified by the addition of DCl or NaOD in the pH range from 6 to 9, highlighting the stability of the system in this pH window.24
The calix[6]tren ligand 1 was also successfully incorporated into DPC micelles, by simple mixing in D2O of the receptor and the surfactant, as attested by the NMR spectrum.21 It was however necessary to lower the pH to ∼3, showing that the ligand must be protonated at the level of its basic tren cap (1·nH+) in order to be incorporated.25 This result suggests that the favourable electrostatic interaction of the positively charged 1·nH+ and 1·Zn2+ with the phosphate group of the zwitterionic surfactant is a driving force for their incorporation (Fig. 3). It is noteworthy to mention that the OMe groups of the incorporated receptor 1·nH+ are pointing inside the cavity (δOMe = 2.41 ppm), clearly highlighting the absence of a guest molecule inside the cavity. The efficient incorporation of 1·nH+ and 1·Zn2+ into DPC micelles encouraged us to undertake host–guest complexation studies with these systems.
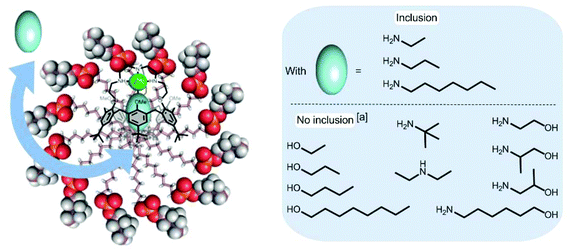 |
| Fig. 3 Summary, using a schematic representation, of the host–guest properties of the receptor 1·Zn2+ incorporated in DPC micelles. [a]: see ESI† for experimental conditions.21 | |
Guest complexation by 1·Zn2+ in DPC micelles
Propylamine (PrNH2) is known to display a high affinity for 1·Zn2+ in CDCl3.17 A titration with PrNH2 of the receptor in DPC micelles was monitored by 1H NMR spectroscopy. As the DPC solution was not buffered, the addition of the amine increased the pH somewhat during the titration (from 7.6 to 8.6). The following observations can be made from the spectrum recorded with the addition of 5 equiv. of PrNH2 (pH = 8.6) (Fig. 2d):
(i) High-field signals for the protons of the alkyl chain of PrNH2 (i.e. −1.62 and −1.95 ppm) attest of its inclusion inside the cavity and thus of the formation of the complex 1·Zn2+⊃PrNH2. These chemical shifts are similar to those obtained with the same system in CDCl3 suggesting a similar positioning of the guest in the cavity (Fig. 2c vs. 2d). The exchange process is slow on the NMR chemical shift timescale.
(ii) There are only small changes in the chemical shifts of the aromatic and tBu calixarene protons, which shows that the conformation of the receptor essentially does not change upon inclusion of a small amine such as propylamine. The receptor maintains a flattened cone conformation clearly evidenced by the large difference between the resonances of the tBu or ArH protons pointing inside and pointing outside the cavity (ΔδtBu ∼ 0.65 ppm and ΔδArH ∼ 0.67 ppm).
These NMR data show that the host–guest properties of 1·Zn2+ toward amines are maintained in the micellar environment (Fig. 3). An apparent binding constant for propylamine (Kapp) of ∼5 × 103 M−1 for a 1
:
1 binding was determined by signal integration at pH ∼ 8.26 At pH ∼10, this constant was estimated to be >104 M−1. The complex 1·Zn2+⊃PrNH2 was observed on a larger pH window (pH from ∼3 to ∼11) in the micelle than complex 1·Zn2+ devoid of organic guest coordinated to the Zn atom (vide supra). From these data, a pseudo pKa of ∼4.3 for complexed propylamine could be estimated.21 This value can be compared to the pKa value of free propylamine of 10.6. This remarkable result shows the extraordinary stabilization of the amine due to its complexation to the metal cation inside the calixarene cavity. This pKa shift is comparable to the one estimated for heptylamine with a related water-soluble Zn-calixarene receptor bearing imidazole moieties.27 It is interesting to point out that this related receptor does not complex PrNH2 in water, which highlights the role played by the micellar environment on the complexation properties of the calix[6]tren-based system.
Complex 1·Zn2+ in DPC micelles also binds ethylamine (EtNH2) and heptylamine (HeptylNH2) as evidenced by the presence of high field signals for their alkyl chain protons in the 1H NMR spectra.21 The affinity constants for these amines relative to PrNH2 were evaluated by competition experiments (Table 1)28 and the following sequence of affinities was observed at pH ∼ 11.1 (±0.1): EtNH2 > PrNH2 > HeptylNH2. They are all of the same order of magnitude, with, however, a significant advantage to the smallest amine, in spite of its lower lipophilicity. This may be explained by a better fit of the guest considering the volume of the calix[6]arene cone as defined by the tBu substituents at the large rim. Indeed, with other related p-tBu-substituted calix[6]arene-based metal complexes studied in chloroform, it has been shown that 3- and 4-atom length guests that are fully encapsulated in the cavity (as shown here by the high-field shifted 1H-NMR signals), display the highest affinities. In contrast, the alkyl chain of the larger amino guest has to protrude outside the cavity and, in order to avoid a steric clash with the tBu groups, the calix[6]arene skeleton is forced to adopt an energetically less favourable straight conformation.29 Finally, it is interesting to note that the relative affinity for HeptylNH2 (vs. PrNH2) in the micellar solution is spectacularly much higher than the one measured in CDCl3 (i.e. 0.5 vs. 0.00025). Such a difference may well reflect the benefit of the hydrophobic effect for lipophilic amine binding in water.
Table 1 Affinities, relative to PrNH2 (pKa = 10.58), of various guests G for host 1·Zn2+
G (pKa)b |
Relative affinitiesa |
DPC (20 mM in D2O) |
CDCl3 |
Defined as [Gin]/[PrNH2in] × [PrNH2Free]/[GFree] and measured at 298 K. The subscript “in” stands for “included”. Errors estimated ±20%.
For the pKa values, see ref. 30.
Not detected with up to 50 equiv. of HO(CH2)2NH2.
|
EtNH2 (10.67) |
1.3 (pH ∼ 11.1) |
— |
HeptylNH2 (10.65) |
0.5 (pH ∼ 11.2) |
∼0.00025 |
HO(CH2)2NH2 (9.50) |
n.d.c (pH ∼ 9.4) |
0.8 |
Very interestingly, the binding of tBuNH2 as well as the secondary amine (Et)2NH was not observed (from pH ∼ 8 to pH ∼ 11) despite the fact that these amines are less hydrophilic than EtNH2 or PrNH2. This result shows that steric hindrance at the level of amino group is a major factor of selectivity and thus highlights the cavity-based selectivity ensured by the incorporated receptor. The complexation of other families of guests such as alcohols (ethanol, propanol, butanol, octanol) and aminoalcohols (ethanolamine, (±)-1-amino-2-propanol, (±)-2-amino-1-propanol, 6-amino-1-hexanol) was also studied. Again, no sign of inclusion was evidenced for any of these guests (Fig. 3). The fact that octanol, which should like heptylamine benefit from the hydrophobic effect, is not complexed by the calixarene suggests that the amino group plays a key role for complexation. It has indeed been shown that the affinity of 1·Zn2+ for ethanol in CDCl3 is four orders of magnitude lower than for propylamine (KEtOH/PrNH2 ∼2.5 × 10−4).17 In order to understand why the aminoalcohols, and in particular the small ethanolamine, are not recognized by 1·Zn2+ incorporated into DPC micelles, the affinity for ethanolamine was measured in CDCl3. The relative affinity compared to PrNH2 was determined to be close to 1 (KPrNH2/Ethanolamine ∼0.8, see Table 1). Such a discrepancy between the results obtained in water/micelle conditions and in an organic solvent is also assignable to the hydrophobic effect that obviously plays a major role in the host–guest recognition processes and in the observed selectivities. In this context, it is worth pointing out that DOSY experiments undertaken with DPC solutions showed that PrNH2 does not incorporate into the DPC micelles but that HeptylNH2 co-micellizes. The diffusion coefficient of PrNH2 in the presence of DPC micelles is indeed identical to the diffusion coefficient of the amine in D2O and this is not the case for HeptylNH2 which clearly partitions itself between the aqueous and micellar environments. This difference can be rationalized in terms of the hydrophobicity of the amines which are more hydrophobic with increasing chain length.
Guest complexation by 1·nH+ into DPC micelles
We have previously shown that calix[6]arene-based receptors bearing either a polyammonium,31 polyamido32 or polyureido cap33 can bind with a high affinity the neutral guest 2-imidazolidinone (Imi) in their cavity. As shown on the XRD structure of one of these host–guest systems,34 the high affinity for Imi stems from a complementary DAAD-ADDA quadruple H-bonding array between the urea guest and the calixarene host. Imi is also recognized by the protonated calixtren derivative 1·nH+ in CDCl3 and, to date, behaves as the best “key” for this receptor.35 The binding of this neutral guest was thus evaluated in the micellar environment by NMR spectroscopy. It was observed that Imi (∼230 equiv.) is not recognized by 1·Zn2+ incorporated in DPC micelles (from pH ∼8 to ∼11). However, 1·nH+ incorporated in DPC micelles at low pH complexes Imi under a slow exchange regime on the NMR chemical shift timescale. A singlet at 0.22 ppm is observed for the two methylene groups of the included Imi and an impressive downfield shift of the signal corresponding to the OMe groups (ΔδOMe = 1.35 ppm) attests to the ejection of these groups from the cavity upon guest inclusion. A large excess of Imi (40 equiv.), which is very soluble in water, was however required to observe the binding. Unfortunately, the addition of Imi also leads to a pH increase and ultimately to a partial deprotonation of receptor 1. As a result, quantitative formation of the complex 1·nH+⊃Imi was difficult to achieve. From signal integration, the binding constant is estimated to be lower than 10 M−1 at pH = 5.3.
Reversible interconversion of host–guest adducts 1·Zn2+⊃PrNH2 and 1·nH+⊃Imi
In CDCl3, the quantitative acid–base induced interconversion of complexes 1·Zn2+⊃EtOH and 1·nH+⊃EtOH was previously reported.17 In order to see if such a supramolecular switching process can be transposed into water and triggered by a pH change, the inter-conversion of the 1·Zn2+⊃PrNH2 and 1·nH+⊃Imi host–guest adducts incorporated into DPC micelles was tested (Fig. 4). First, PrNH2 (4.5 equiv.) and Imi (4 equiv.) were added to a solution of 1·Zn2+ in DPC micelles and the resulting NMR spectrum (pH = 10.5) clearly showed the exclusive formation of 1·Zn2+⊃PrNH2 (Fig. 4a). Fig. 4b shows the spectrum of the same solution at pH 3.1 after the addition of DCl and a large excess of Imi (∼106 equiv.) It evidences the disappearance of complex 1·Zn2+⊃PrNH2 and the formation of 1·nH+⊃Imi together with some free protonated receptor 1·nH+. The subsequent addition of NaOD (to reach pH = 8.8) restored very easily the initial NMR profile corresponding to 1·Zn2+⊃PrNH2 (Fig. 4c). This experiment highlights that the host–guest properties of receptor 1 can be nicely tuned and controlled by the pH.
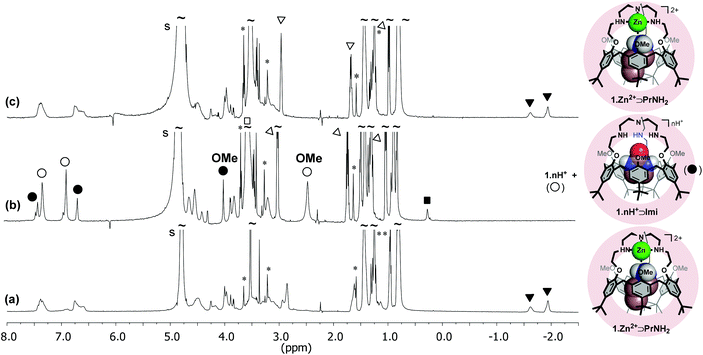 |
| Fig. 4
1H NMR spectra (600 MHz, 298 K, DPC-d38 20 mM in D2O) of (a) complex 1·Zn2+ in the presence of ∼4.5 equiv. of PrNH2 and ∼4 equiv. of Imi (pH = 10.5); (b) after the subsequent addition of DCl and ∼106 equiv. of Imi (pH = 3.1); (c) after the subsequent addition of NaOD (pH = 8.8). ▼: PrNH2 in; ▽: PrNH2 out; ■: Imi in; □: Imi out; ●: 1·nH+⊃Imi; ○: 1·nH+; s: solvent. | |
Conclusion
In summary, we have shown that a calix[6]azacryptand (1) can be incorporated into DPC micelles either as a Zn2+ complex in the [6–9] pH window or as a polyammonium at low pH. The 1·Zn2+ complex, incorporated into DPC micelles, is able to host selectively small or long linear primary amines with high affinity constants. This corresponds to one of the rare examples of systems capable of recognising primary amines in water media.36 Interestingly, the host–guest complex 1·Zn2+⊃PrNH2 was detected in the micelles in an even larger pH window (3–11) than was the 1·Zn2+ host itself, which shows that the presence of the amine strengthens the complex even at very low pH where the free amine is fully protonated in water. Moreover, we observed a quite remarkable pseudo pKa shift of propylamine recognised by 1·Zn2+ in DPC micelles (from 10.6 to 4.3). This impressive stabilisation of the basic form of propylamine is the result of its coordination to Zn2+ but also the environment provided by the calixarene cavity and the micelle which protects the system from the water. The 1·Zn2+ complex preferentially binds small amines and this size selectivity stands in strong contrast with the previously reported water-soluble calixarene-based Zn2+ complex for which hydrophobic amine binding could be detected, but not with small hydrophilic ones.27 With free receptor 1, no amine binding was detected even at low pH. However, an entrapped urea guest (i.e. Imi) could be observed, though only at a high concentration. Interestingly, a pH driven guest switch was evidenced with 1·Zn2+ in the presence of both an amine and Imi: at high pH, the Zn2+ complex binds the amine, at low pH the amino arms are protonated, which leads to the decoordination of the metal ion and the embedment of Imi. All in all, these results validate the very simple strategy that consists of incorporating an organo-soluble receptor in micelles to obtain water compatible nanosized supramolecular recognition devices. Efforts are now being directed towards the design of calixarene-based sensing systems incorporated in micelles.
Experimental section
Chemicals
DPC and DPC-d38 were purchased from Avanti Lipids and used with no further purification. D2O and CDCl3 were purchased from VWR BDH PROLABO. Compounds 1 and 1·Zn2+ were synthesized according to the literature.15,16
General procedures
1H NMR spectra were recorded on a 600 MHz, 400 MHz or a 300 MHz spectrometer. For the 1D 1H spectra, parameters (acquisition time, recycling times, and signal accumulation) were chosen so as to ensure that quantitative data could be obtained from signal integration. Traces of residual solvents were used as internal chemical shift references. Chemical shifts are quoted on the δ scale. The NMR spectra were recorded at 298 K unless otherwise stated.
NMR solutions preparation
All solutions for NMR studies were prepared following a similar protocol: 1·Zn2+ (∼4 mg) and DPC (∼35 mg, deuterated or not depending on the experiments) were added to 5 mL of D2O in order to have, respectively, concentrations of ∼0.5 mM and ∼20 mM. Solutions were stirred until a clear solution was obtained.
1H NMR characterization of various complexes 1·Zn2+⊃nH2O
1H NMR (DPC 20 mM in D2O, 298 K, 600 MHz): δ (ppm) 0.79 (bs, 27H, tBu), 1.44 (bs, 27H, tBu), 3.97 (bs, 9H, OMe), 2.87–4.61 (m, 24H, ArCH2eq/ArCH2ax/CH2N/CH2O), 6.49–6.81 (m, 6H, ArHOMe), 7.40 (bs, 6H, ArHcap). 1·Zn2+⊃PrNH2: 1H NMR (DPC 20 mM in D2O, 298 K, 600 MHz): * δ (ppm) −1.95 (bs, 3H, CH3PrNH2,in), −1.63 (bs, 2H, CH2PrNH2,in), 0.74–0.87 (m, 27H, tBu), 1.36–1.57 (m, 27H, tBu), 2.99–4.68 (m, 33H, ArCH2eq/ArCH2ax/CH2N/CH2O/OMe), 6.49–6.99 (m, 6H, ArHOMe), 7.22–7.51 (m, 6H, ArHcap). * The chemical shift of the CH2N of PrNH2 (in) was not observed due to overlapped signals.
Determination of the apparent affinity of PrNH2 toward complex 1·Zn2+ through 1H NMR titration in DPC (20 mM in D2O)
Different aliquots of a solution of amine (PrNH2) were progressively added to 500 μL of a DPC solution (20 mM in D2O) containing the complex 1·Zn2+. Non-deuterated DPC was used and the integration of the CH2β and CH2γ signals were used as internal reference to determine the concentration of 1·Zn2+. The integrations of the free guests and of the included guests were used to calculate the apparent affinity defined as [PrNH2in]/(1 − [PrNH2in] [PrNH2Free][1·Zn2+]) (errors estimated ±20%).
Determination of the relative affinities of heptyl- and ethylamine compared to propylamine through 1H NMR competitive binding studies in DPC (20 mM in D2O)
At room temperature, ∼7 equivalents of heptylamine (HeptylNH2) or ∼3 equivalents of ethylamine (EthylNH2) were added to 500 μL of a DPC solution (20 mM in D2O) containing the complex 1·Zn2+. Propylamine (PrNH2) was then added (∼5 equivalent). The 1H NMR spectrum showed the guest resonances of both complexes 1·Zn2+⊃PrNH2 and 1·Zn2+⊃HeptylNH2 or 1·Zn2+⊃EthylNH2 and signals corresponding to the free amines. The integrations of the signals corresponding to the free guests and included guests were used to calculate relative affinity defined as [Gin]/[PrNH2in] × [PrNH2Free]/[GFree] (errors estimated ±20%).
Determination of the relative affinity of ethylamine compared to propylamine in CDCl3via1H NMR competitive binding studies
At room temperature, ∼30 equivalents of propylamine (PrNH2) and ∼15 equivalents of ethylamine (EthylNH2) were added in 500 μL of a CDCl3 solution of complex 1·Zn2+. A 1H NMR spectrum showed the guest resonances of both complexes 1·Zn2+⊃PrNH2 and 1·Zn2+⊃EthylNH2 and signals corresponding to the free amines. The integrations of the signals corresponding to free guests and the included guests were used to calculate relative affinity defined as [EtNH2in]/[PrNH2in] × [PrNH2Free]/[EtNH2Free] (errors estimated ±20%).
Determination of the relative affinity of heptylamine compared to DMF in CDCl3via1H NMR competitive binding studies
At room temperature, ∼40 equivalents of dimethylformamide (DMF) and ∼100 equivalents of heptylamine (HeptylNH2) were added in 500 μL of a CDCl3 solution of complex 1·Zn2+. A 1H NMR spectrum showed the guest resonances of both complexes 1·Zn2+⊃DMF and 1·Zn2+⊃HeptylNH2 and the signals corresponding to free DMF and HeptylNH2. The integrations of the signals corresponding to the free and included guests were used to calculate relative affinity defined as [DMFin]/[HeptylNH2in] × [HeptylNH2Free]/[DMFFree] (errors estimated ±20%). In order to obtain the value corresponding to KPrNH2/HeptylNH2, we used the value for KPrNH2/DMF from ref. 17 (1610).
Reversible interconversion of host–guest adducts 1·Zn2+⊃PrNH2 and 1·nH+⊃Imi
PrNH2 (4.5 equiv.) and Imi (4 equiv.) were added to a ∼0.5 mM solution of 1·Zn2+ in DPC-d38 micelles (pH ∼10.5). In a second stage, DCl and a large excess of Imi (∼106 equiv.) were added to the system (pH ∼3.1). In the final stage, NaOD was added to reach pH = 8.8.
DOSY experimental parameters
15 values for the magnitude of the gradient pulses (ranging from 2 G cm−1 to 50 G cm−1), diffusion delay 50 ms, time-length of the gradient 5 ms, acquisition time 2 s, relaxation delay 7 s, 32 transients.
PRE experimental parameters
For the PRE experiments, aliquots of 5 μl of a 5 mM solution of K3[Cr(CN)6] in D2O were added to the 600 μl solution under study. T1 measurements were undertaken using the classical inversion recovery (180 – τ – 90 – acquisition) sequence, with 15 points and the delay varying between 0 and 7 s. For each signal monitored, the increase in the longitudinal relaxation rate induced by the paramagnetic species (Δ1/T1; the difference between the longitudinal relaxation rate measured in the presence and in the absence of the paramagnetic species) was plotted as a function of the concentration of paramagnetic species. A linear regression was undertaken with the experimental data points from which the relaxivity value was derived (slope of the line).
Acknowledgements
E. B., A. I. and F. K. have a PhD grant from the Fonds pour la formation à la Recherche dans l'Industrie et dans l'Agriculture (FRIA-FRS, Belgium). This research was supported by the Fonds de la recherche scientifique (FNRS; FRFC 2.4.617.10.F project), the Agence Nationale de la Recherche (ANR10-BLAN-714 Cavity-zyme(Cu) project), and undertaken within the framework of the COST Action CM1005 “Supramolecular Chemistry in Water”.
Notes and references
-
(a)
J.-M. Lehn, in Supramolecular Chemistry, Wiley-VCH, Weinheim, 1995 Search PubMed;
(b)
J. W. Steed, D. R. Turner and K. J. Wallace, in Core Concepts in Supramolecular Chemistry and Nanochemistry, Wiley-VCH, Chippenam, 2007 Search PubMed;
(c) J. Hartley, T. D. James and C. J. Ward, J. Chem. Soc., Perkin Trans. 1, 2000, 3155–3184 RSC.
-
B. Valeur and J. C. Brochon, New Trends in Fluorescence Spectroscopy: Applications to Chemical and Life Sciences, Springer, Berlin, 2001 Search PubMed.
- G. V. Oshovsky, D. N. Reinhoudt and W. Verboom, Angew. Chem., Int. Ed., 2007, 46, 2366–2393 CrossRef CAS PubMed.
- J. Szejtli, Chem. Rev., 1998, 98, 1743–1753 CrossRef CAS PubMed.
- J. Lagona, P. Mukhopadhyay, S. Chakrabarti and L. Isaacs, Angew. Chem., Int. Ed., 2005, 44, 4844–4870 CrossRef CAS PubMed.
- P. Sinaÿ and M. Sollogoub, Chem. Commun., 2006, 1112–1114 Search PubMed.
-
(a) W. Chu and C. Y. Kwan, Chemosphere, 2003, 53, 9–15 CrossRef CAS;
(b) C. e O. Rangel-Yagui, A. Pessoa and L. C. Tavares, J. Pharm. Pharm. Sci., 2005, 8, 147–163 CAS;
(c) M. Ding, J. Li, H. Tan and Q. Fu, Soft Matter, 2012, 8, 5414–5428 RSC.
-
(a) N. Basilio, M. Martín-Pastor and L. García-Río, Langmuir, 2012, 28, 6561–6568 CrossRef CAS PubMed;
(b) S. Uchiyama, K. Iwai and A. P. de Silva, Angew. Chem., Int. Ed., 2008, 47, 4667–4669 CrossRef CAS PubMed;
(c) F. Su, R. Alam, Q. Mei, Y. Tian, C. Youngbull and R. H. Johnson, PLoS One, 2012, 7, 1–7 Search PubMed;
(d) Y. J. Kim, M. T. Lek and M. P. Schramm, Chem. Commun., 2011, 47, 9636–9638 RSC;
(e) T. Riis-Johannessen and K. Severin, Chem. – Eur. J., 2010, 16, 8291–8295 CrossRef CAS PubMed.
- S. Javor and J. Rebek, J. Am. Chem. Soc., 2011, 133, 17473–17478 CrossRef CAS PubMed.
-
(a) F. Keymeulen, P. De Bernardin, A. Dalla Cort and K. Bartik, J. Phys. Chem. B, 2013, 117, 11654–11659 CrossRef CAS PubMed;
(b) M. Cametti, A. Dalla Cort and K. Bartik, ChemPhysChem, 2008, 9, 2168–2171 CrossRef CAS PubMed.
- A. Dalla Cort, G. Forte and L. Schiaffino, J. Org. Chem., 2011, 76, 7569–7572 CrossRef CAS PubMed.
- D. Coquière, S. Le Gac, U. Darbost, O. Sénèque, I. Jabin and O. Reinaud, Org. Biomol. Chem., 2009, 7, 2485–2500 Search PubMed.
-
C. D. Gutsche, in Calixarenes: An introduction, Monographs in Supramolecular Chemistry, ed. J. F. Stoddart, The Royal Society of Chemistry, Cambridge, 2nd edn, 2008 Search PubMed.
-
(a) J.-N. Rebilly and O. Reinaud, Supramol. Chem., 2014, 26, 454–479 CrossRef CAS;
(b) J.-N. Rebilly, B. Colasson, O. Bistri, D. Over and O. Reinaud, J. Chem. Soc. Rev., 2015, 44, 467–489 RSC.
- I. Jabin and O. Reinaud, J. Org. Chem., 2003, 68, 3416–3419 CrossRef CAS PubMed.
-
(a) G. Izzet, J. Zeitouny, H. Akdas-Killig, Y. Frapart, S. Ménage, B. Douziech, I. Jabin, Y. Le Mest and O. Reinaud, J. Am. Chem. Soc., 2008, 130, 9514–9523 CrossRef CAS PubMed;
(b) G. Izzet, B. Douziech, T. Prangé, A. Tomas, I. Jabin, Y. Le Mest and O. Reinaud, Proc. Natl. Acad. Sci. U. S. A., 2005, 102, 6831–6836 CrossRef CAS PubMed;
(c) U. Darbost, X. Zeng, M.-N. Rager, M. Giorgi, I. Jabin and O. Reinaud, Eur. J. Inorg. Chem., 2004, 4371–4374 CrossRef CAS.
- U. Darbost, M.-N. Rager, S. Petit, I. Jabin and O. Reinaud, J. Am. Chem. Soc., 2005, 127, 8517–8525 CrossRef CAS PubMed.
- This value is far above the critical micellar concentration (cmc = 1.1 mM). See: R. Stafford, T. Fanni and E. Dennis, Biochemistry, 1989, 28, 5113–5120 CrossRef CAS.
- The assignment of the OMe signal was confirmed by a NMR HSQC experiment.
- Similarly to related complexes, the presence of a second water guest, H-bonded to the coordinated one, is likely. See: O. Sénèque, M.-N. Rager, M. Giorgi and O. Reinaud, J. Am. Chem. Soc., 2001, 123, 8442–8443 CrossRef.
- See the ESI.†.
- T. Lazaridis, B. Mallik and Y. Chen, J. Phys. Chem. B, 2005, 109, 15098–15106 CrossRef CAS PubMed.
- R. B. Lauffer, Chem. Rev., 1987, 87, 901–927 CrossRef CAS.
- Note that all pH values have been measured in D2O solutions.
- Note that studies undertaken with the tren ligand in water have shown that, at pH ∼ 3, the ligand is protonated at least three times. See: J. W. Canary, J. Xu, J. M. Castagnetto, D. Rentzeperis and L. A. Marky, J. Am. Chem. Soc., 1995, 117, 11545–11547 CrossRef CAS.
- As the DPC solution was not buffered, it is therefore difficult to discuss this binding constant quantitatively.
- O. Bistri, B. Colasson and O. Reinaud, Chem. Sci., 2012, 3, 811–818 RSC.
- All measurements were conducted in pure water, i.e. in absence of buffer in order to avoid any interaction with the complex and micelles that could alter the results.
-
(a) O. Sénèque, M.-N. Rager, M. Giorgi and O. Reinaud, J. Am. Chem. Soc., 2000, 122, 6183–6189 CrossRef;
(b) E. Brunetti, J.-F. Picron, K. Flidrova, G. Bruylants, K. Bartik and I. Jabin, J. Org. Chem., 2014, 79, 6179–6188 CrossRef CAS PubMed.
-
H. C. Brown, D. H. McDaniel and O. Häfliger, in Determination of Organic Structures by Physical Methods, ed. E. A. Braude and F. C. Nachod, Academic Press, New York, 1955 Search PubMed.
- E. Garrier, S. Le Gac and I. Jabin, Tetrahedron: Asymmetry, 2005, 16, 3767–3771 CrossRef CAS PubMed.
- A. Lascaux, S. Le Gac, J. Wouters, M. Luhmer and I. Jabin, Org. Biomol. Chem., 2010, 8, 4607–4616 CAS.
-
(a) M. Ménand and I. Jabin, Chem. – Eur. J., 2010, 16, 2159–2169 CrossRef PubMed;
(b) D. Cornut, J. Marrot, J. Wouters and I. Jabin, Org. Biomol. Chem., 2011, 9, 6373–6384 RSC.
- S. Le Gac, J. Marrot, O. Reinaud and I. Jabin, Angew. Chem., Int. Ed., 2006, 45, 3123–3126 CrossRef CAS PubMed.
-
S. Le Gac, I. Jabin and O. Reinaud, in Bioinspiration and Biomimicry in Chemistry, ed. G. Swiegers, Wiley-VCH, 2012, ch. 12 Search PubMed.
-
(a) J. Kumpf and U. H. F. Bunz, Chem. – Eur. J., 2012, 18, 8921–8924 CrossRef CAS PubMed;
(b) G. Gattuso, A. Notti, S. Pappalardo, M. F. Parisi and I. Pisagatti, Supramol. Chem., 2014, 26, 597–600 CrossRef CAS;
(c) T. Mizutani, K. Wada and S. Kitagawa, J. Org. Chem., 2000, 65, 6097–6106 CrossRef CAS PubMed;
(d) E. K. Feuster and T. E. Glass, J. Am. Chem. Soc., 2003, 125, 16174–16175 CrossRef CAS PubMed.
Footnote |
† Electronic supplementary information (ESI) available: 2D DOSY experiment of 1·Zn2+ incorporated in DPC. NMR PRE experiment with 1·Zn2+ in DPC. 1H NMR spectrum of 1·nH+ in DPC. Determination of the pseudo pKa for PrNH2 with 1·Zn2+ in DPC. 1H NMR spectra of 1·Zn2+ in the absence and presence of EtNH2 in DPC-d38. 1H NMR spectra of 1·Zn2+ in the absence and presence of HeptylNH2 in DPC. Experimental conditions for titrations of 1·Zn2+ with amines, alcohols and aminoalcohols in DPC. See DOI: 10.1039/c4ob02495h |
|
This journal is © The Royal Society of Chemistry 2015 |
Click here to see how this site uses Cookies. View our privacy policy here.