DOI:
10.1039/D3NR00425B
(Review Article)
Nanoscale, 2023,
15, 10882-10903
DNA hydrogels and nanogels for diagnostics, therapeutics, and theragnostics of various cancers
Received
29th January 2023
, Accepted 15th May 2023
First published on 15th May 2023
Abstract
As an efficient class of hydrogel-based therapeutic drug delivery systems, deoxyribonucleic acid (DNA) hydrogels (particularly DNA nanogels) have attracted massive attention in the last five years. The main contributor to this is the programmability of these 3-dimensional (3D) scaffolds that creates fundamental effects, especially in treating cancer diseases. Like other active biological ingredients (ABIs), DNA hydrogels can be functionalized with other active agents that play a role in targeting drug delivery and modifying the half-life of the therapeutic cargoes in the body's internal environment. Considering the brilliant advantages of DNA hydrogels, in this survey, we intend to submit an informative collection of feasible methods for the design and preparation of DNA hydrogels and nanogels, and the responsivity of the immune system to these therapeutic cargoes. Moreover, the interactions of DNA hydrogels with cancer biomarkers are discussed in this account. Theragnostic DNA nanogels as an advanced species for both detection and therapeutic purposes are also briefly reviewed.
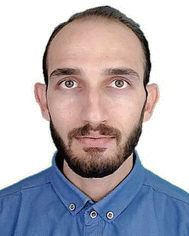 Iman Zare | Iman Zare received his BSc degree in Cellular and Molecular Biology from Semnan University in 2016. He focuses on bioengineering, synthetic biology, and nanomedicine to discover solutions to shift the paradigm of current treatments. He strives to harness biological schemes of nanosystems, emerging techniques, and innovative systems in the theragnostics of chronic diseases that lead to clinical trials. To achieve these aims, he founded Sina Medical Biochemistry Technologies Co. in 2021. He is also a member of the Global Burden of Disease (GBD), as a researcher of the global program to assess major diseases, injuries, and risk factors from 2021. His scientific interests include bioengineering, synthetic biology, nanomedicine, organ-on-chip, biostatistics, and bioinformatics. |
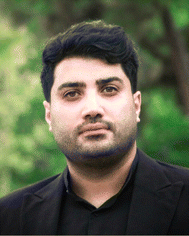 Reza Taheri-Ledari | Dr Reza Taheri-Ledari was born in Tehran in 1988. He graduated from the University of Tehran (UT) with B.Sc. (in 2012) and M.Sc. (in 2015) degrees in Pure and Organic Chemistry, respectively. He received his Ph.D. (in 2021) in Organic Chemistry from the Iran University of Science and Technology (IUST). Reza's research interest focuses on drug development, drug delivery, and high-tech pharmaceutical compounds like antibody–drug conjugates. So far, Reza has succeeded in publishing several ISI publications involving foreign researchers from China, USA, Spain and Canada. He was the Top Researcher of IUST in 2019 & 2020 and also one of the World's Top 2% Scientists in 2021 & 2022 (Web of Science). |
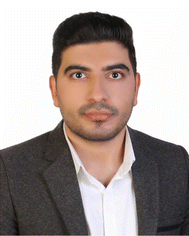 Farhad Esmailzadeh | Farhad Esmailzadeh was born in Mahshahr, Iran, in 1997. He graduated from Yasuj University with a B.Sc. degree in Applied Chemistry in 2019. He completed his M.Sc. in 2021 from the Iran University of Science and Technology (IUST) in Nanochemistry as the first-ranked student. In 2021, he started his Ph.D. at the Sharif University of Technology. His research interests include designing and preparing multifunctional biomaterials and nanocomposites for therapeutic applications and water treatment. |
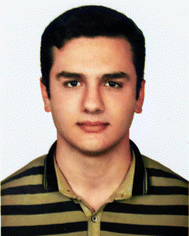 Mohammad Mehdi Salehi | Mohammad Mehdi Salehi grew up in Ahvaz/Iran and received his BS in Applied Chemistry from the Shahid Chamran University of Ahvaz (SCU) in 2020. Then, he continued his study in nanochemistry as an MSc student in the Chemistry Department of Iran University of Science & Technology (IUST) and graduated in 2022 under the supervision of Prof. Ali Maleki. Currently, he is working on different projects at IUST, as a Senior Researcher focusing on water purification, nanocatalysts, green synthesis, materials sciences, and bionanocomposites. |
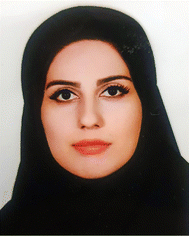 Adibeh Mohammadi | Adibeh Mohammadi was born in Masjed Soleyman in 1993. She graduated from the Shahid Bahonar University of Kerman (SBUK) with a BSc degree in Pure Chemistry in 2017. She completed her M.Sc. in 2020 from the Iran University of Science and Technology (IUST) in Organic chemistry. She became a PhD student in Organic Chemistry under the supervision of Prof. Ali Maleki at the Iran University of Science and Technology (IUST) in 2020. She is interested in nano-biotechnology research, catalysts, drug delivery, and cancer therapy. |
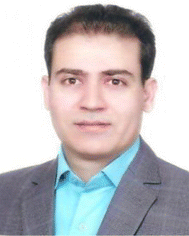 Ali Maleki | Prof. Dr Ali Maleki was born in Mianeh, East Azerbaijan in 1980. He received his Ph.D. in Chemistry in 2009. He started his career as an Assistant Professor at the Iran University of Science and Technology (IUST) in 2010, where he is currently Full Professor. His research interests focus on the design and development of novel catalysts, nanomaterials, and green chemistry. He has hundreds of ISI-JCR publications. Some of his honors include the Distinguished Researcher of IUST in 2010–2020, the IUPAC Prize for green chemistry in 2016, and the Top 1% International Scientists in ESI (Web of Science) in 2018, 2019, and 2020. |
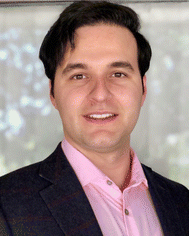 Ebrahim Mostafavi | Dr Ebrahim Mostafavi has so far received training at Stanford University School of Medicine (PostDoc), Northeastern University (Ph.D.), Harvard Medical School (Researcher), and University of Tehran (M.Sc. and B.Sc.). His research interests revolve around the engineering and development of (nano)biomaterials, nanocarriers, and 3D in vitro models (hydrogels, 3D bioprinted constructs, nanofibrous scaffolds, organoids, vascular grafts, and microfluidic systems) to create biologically complex systems for a range of applications such as tissue engineering and regenerative medicine, translational medicine, cancer therapy (with focus on women's cancer), biosensing, and infectious diseases. Dr Mostafavi serves as Associate Editor-in-Chief of several prestigious and high-impact journals of Elsevier, Springer, Cell Press, Dove Medical Press, T&F, Frontiers, etc. He is also an Editorial Board Member of 30+ impactful and prestigious biomedical and materials science journals. His scholarly work comprises >150 publications with an H-index of 35 (i10-index = 96), including papers published in The Lancet family (i.e., Oncology, Infectious Diseases, Public Health, Global Health, etc.). So far, he has edited several books such as “Pharmaceutical Nanobiotechnology for Targeted Therapy” and “Emerging Nanomaterials and Nano-Based Drug Delivery Approaches to Combat Antimicrobial Resistance”. He has also contributed to leading more than 45 introductory book chapters in a very multidisciplinary field of bio/medical engineering, biotechnology, nanotechnology, materials science, and regenerative/translational medicine. |
1. Introduction
The utilization of active biological ingredients (ABIs) in drug delivery has recently prospered due to several advantages, such as high compatibility with the body's internal environment and convenient degradation without harm.1,2 The main contributor to this is high levels of constructive interactions between the ABI drug carrier and the target organ or tissue.3,4 Among various ABIs, attention to deoxyribonucleic acid (DNA) hydrogels for cancer gene therapies has increased in the last five years.5,6 DNA is responsible for cell proliferation and structurally includes a double-strand spiral shape with a native structure based on the hydrogen bond (H-bond) interactions between the organic bases, namely adenine, thymine, guanine, and cytosine.7,8 Nowadays, the compilation of Watson–Crick pairing methods and nucleotide synthesis strategies has opened new windows for researchers in the field of materials science.9,10 Generally, hydrogels are considered as a highly appropriate substrate in various areas such as energy,11–13 catalysis,14–19 environmental sciences,20–26 and medical applications.27–31 Specifically, in the area of drug delivery to cancer tissues, hydrogels are widely used because they are capable of absorbing water molecules and swelling in the target organ, resulting in drug release in a controlled manner.32,33 DNA-based hydrogels, as the most exciting species that include 3D cross-linked lattices, have recently gained attention.34 In addition to chemical cross-link bonding, physical-based self-assembly interactions can assist the formation of DNA hydrogels.35 Generally, two types of DNA hydrogels can be defined; pure gels, including a single DNA species, and hybrid gels in which two or more DNA species are involved in the hydrogel network, or a DNA species is combined with other types of polymeric materials.36
As the main focus of this review, DNA nanogels are a specific class of hydrogels that include nanoscale dimensions. Since the DNA nature is programmable and chemically adjustable, it would be possible to design various DNA nanogels specifically a particular aim.37,38 As another unique point of DNA nanogels, their capability for functionalization with the other ABIs can be expressed. The 3D structures of DNA nanogels afford substantial rigidity, which is an important parameter in conjugating the DNA scaffolds with the ABIs.39 Like other hydrogel materials, DNA nanogels can be designed so that they respond to exogenous stimuli, such as light and a specific wavelength, to trigger the release process of the encapsulated agent with high control.40,41 Among all of the mentioned advantages of DNA nanogels, the programmability of DNA hydrogels is the most brilliant feature that is not found in other hydrogel materials. This unique property provides a great opportunity to precisely control the shape and size of DNA drug carriers besides a selective function in targeted drug delivery.42,43 Along with the advantages referred to DNA nanogels, potential challenges in real therapies should be noticed as well. As the main concern, low structural stability in a physiological environment especially against enzymatic degradation can be stated.44 Moreover, it is quite probable that the native structure of DNA strands is damaged (or lost) during the drug-loading process. To address this concern, in situ pathways for the incorporation of the target drugs into the DNA nanogel carriers are preferred.
In this review, we intend to describe the exact principles of the design and preparation of the DNA hydrogels (focusing on nanogels), which are applicable for drug delivery purposes. Then, the mechanisms of the immune response to therapeutic DNA hydrogels are discussed. Recognition of the cancerous biomarkers by the DNA hydrogels through biological interactions is presented as well. Afterward, the application of therapeutic DNA hydrogels in cancer therapy is classified based on therapeutic strategies.45 As a newly developed prestige for DNA hydrogels, theragnostic DNA hydrogels are presented at the end of this account. This class of DNA hydrogels is capable of playing a dual role in both therapy and diagnosis. Concisely, this focused review highlights the advantages of DNA hydrogel-based platforms in the area of advanced drug delivery continuing cancer combination therapy.
2. Design of DNA hydrogels and nanogels
DNA hydrogels are a new class of biomaterials that consist of 3D networks containing DNA polymer chains. It is possible to design a DNA chain composed of four deoxyribonucleotide monomers to perform specific functions and have desirable characteristics. DNA chains can have mechanical strength, pH-responsive properties, enzymes, and biomolecules due to their design.46 The principle topology of DNA building blocks is classified into three categories such as circular, linear, and branched types.7 The usual structure of DNA consists of two DNA molecules placed in a helix by hydrogen bonds and interactions between aromatic moieties. Recently, new DNA structures such as i-motifs,47 triple-helixes,48,49 and G-quadruplexes50 have developed the self-assembly behavior and geometry configuration of DNA polymeric chains. The DNA i-motif structure consists of four DNA chains joined by hydrogen bonds between cytosine–cytosine+ (C
:
C+) base pairs. Thus, there are two distinct intercalation topology categories named 3′E and 5′E (Fig. 1).51 According to the i-motif DNA structure, alkaline and neutral conditions are unsuitable for its formation but it can be formed simply under acidic conditions. This property can be helpful in forming pH-responsive hybrid DNA hydrogels (Fig. 2).52 In triple-helix DNA structures, an oligonucleotide chain joins the double helix DNA as the third segment. Hoogsteen interactions play an essential role in the formation of this structure.48 Triple-helix DNAs follow two main types of conformations such as parallel ternary structure configurations (G·G–C, T·A–T, C+·G–C) and antiparallel ternary structures configurations (G·G–C, C+·G–C, A·A–T, T·A–T) (Fig. 3). Various parameters can be effective in the stability of triple-helix DNAs, such as alterations in the triplex domains, the features and numbers of the triplex bridges, and the pH value of the reaction medium. In the third DNA structure, using an alternative base pairing, guanine-rich sequences form four-stranded G-quadruplex structures of DNA. Monovalent cations such as K+ and Na+ may be used to stabilize the mentioned structures.53,54 These designed DNA sequences can be integrated into building blocks to form smart hydrogels with favorable physicochemical features. The design of efficient DNA hydrogels can follow three general strategies, including DNA hybrid hydrogels, dendritic DNA assembled hydrogels, and the entanglement of ultra-long linear DNA chains.46
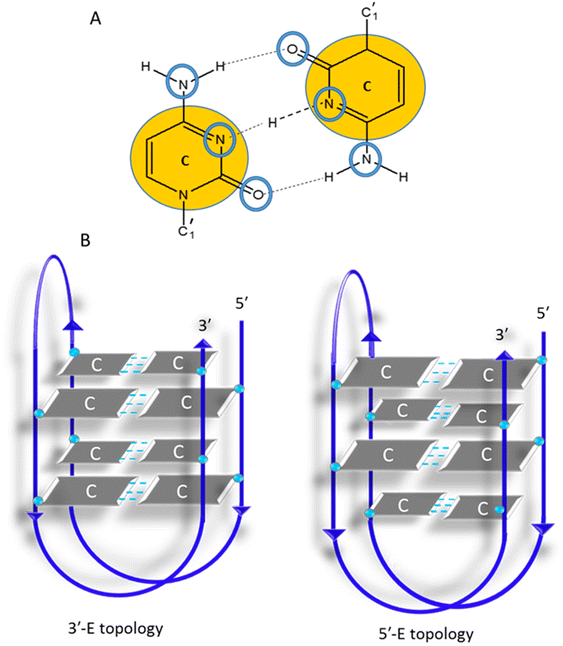 |
| Fig. 1 The C : C+ base pair structure and stacking between intercalating C : C+ base pairs (A), and two intercalation topologies of an intermolecular i-motif DNA structure (B). | |
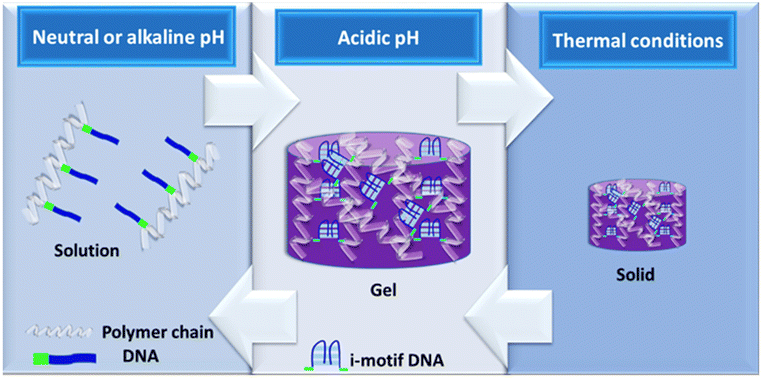 |
| Fig. 2 Overview of pH and thermal-responsiveness i-motif-crosslinked DNA/polymer hydrogel performance. | |
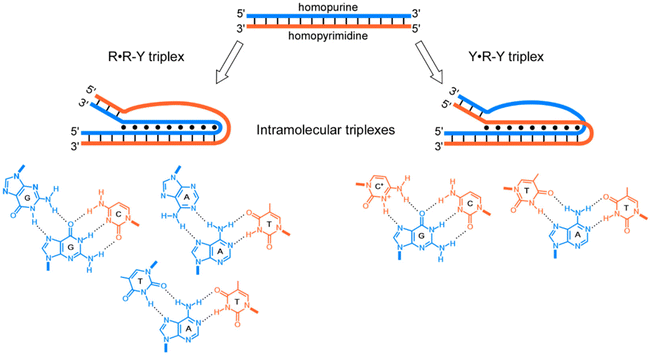 |
| Fig. 3 The five primary forms of intramolecular triple-helix structures.65 Adapted from ref. 65 with permission from Public Library of Science, Copyright 2015. | |
Multicomponent hydrogels have received considerable attention in recent years due to their ability to combine the physical and chemical properties of each component.55 Accordingly, a DNA molecule can be used as a cross-linking agent for a DNA hydrogel. This is based on the first studies conducted by Matsuda and Nagahara in 1996, which showed that the multifunctional hydrogels produced had no adverse effects on the human body.56
Moreover, DNA hydrogels respond to multiple stimuli and can be created by hybridizing DNA sequences with other materials.57 DNA/polymer hybrid hydrogels are an extensive category of hydrogels that can be prepared by integrating stimuli-responsive DNA structures such as i-motif DNA in polymer chains. This type of DNA hydrogel significantly responds to environmental pH and temperature changes.58 In addition, Liu and co-workers constructed peptide–DNA multiple stimuli-responsive hybrid hydrogels that are responsive to proteases and nucleases and are completely degraded under physiological conditions.59 Dendrite assembly hydrogels, as the second group of DNA hydrogels, consists of branched DNA molecules with sticky end-groups that come close to each other and form a 3D network.60,61 Branched DNAs have advantages such as controllable symmetry, size regulation, and multivalence, which complement other advantages of conventional hydrogels, such as biological function, programming, manipulation capabilities, and molecular detection capabilities.7 Based on the results of the studies, it has been demonstrated that in addition to changing the initial concentration of branched DNAs, the swelling profiles of hydrogels can also be adjusted. A higher initial concentration of DNA can contribute to higher degrees of hydrogel swelling. In addition, the swollen gel shows a higher tensile modulus, probably due to greater cross-linking. Another method of creating DNA hydrogels is to extend the DNA chain, which can be done through repeated copying of a circular DNA template.62 This manufacturing method introduced a new way to prepare pure DNA hydrogels using the RCA technique. Also, i-motif DNA can be used as a repeating sequence in DNA chains produced by the RCA technique to achieve a stimulus-responsive hydrogel.63,64
3. Modulation of the tumor immune microenvironment
As the body's primary defense system, the immune system plays an important role. To protect the body from various infections, it produces antibodies against pathogens and allergens when exposed to them. It is believed that vaccines reduce the risk of infections by stimulating the immune system to produce antibodies against the corresponding antigens.66,67 A wide range of immune-related diseases can be treated with immune-modulatory biologics including antigenic proteins, peptides, nucleic acids, adjuvants, drugs, and extracellular matrix components.68 In immunomodulation, it depends on the ultimate goal of (i) decreasing immune cell initiation under conditions characterized by hyperactive immunity, such as transplant rejection, autoimmune diseases, and chronic infections or (ii) inducing immune cell activation to reverse hypoactive immune reactions.69 Treating autoimmune diseases and inflammation requires immunomodulation, which suppresses immune cell initiation. In contrast, some diseases such as allergies are caused by overactive auto-immune responses against foreign substances like pollens. In some cases, healthy tissue can be mistaken for a dangerous substance, triggering an overactive immune response that causes an auto-immune disorder, including rheumatoid arthritis, type 1 diabetes, lupus, and sclerosis.70,71
Cancer and pathogenic microbial infections can be treated with cytosine–phosphate–guanine (CpG) sequences as immune adjuvants. Hydrogels made from DNA triggered antigen-specific immune responses. Hydrogels made from DNA are considered to be a superior biomaterial, especially when it comes to their biological effects.72 The hydrogen atom in DNA possesses both the mechanical properties of polymer macromolecules and their unique properties.73,74 Moreover, DNA hydrogels maintain biological functions originating from DNA sequence programming, including identification of molecules, outstanding biocompatibility, and biodegradability as well as the features of communal hydrogels – an excellent water content, connectivity, consistency, an excellent degree of flexibility, and excellent mechanical aspects.75 DNA hydrogels have several advantages over other hydrogel materials, including self-assembly, programmability, and repairability. There are a variety of functional DNA motif binding sites that can be used to accomplish complex functions, such as receptivity to signals.71,76 Therefore, DNA hydrogels are suitable for the preparation of responsive materials. There are many uses for DNA hydrogels applied to biomedicine, including encapsulating biologically active substances that are released under controlled conditions, making stents for tissues and organs that are artificial, and many others.77,78 Cell culture in 3D, tissue engineering in 3D, and analysis of single cells and multiple biosensors are some of the latest developments. Under acidic conditions, pH-sensitive DNA bridges in the hydrogel are broken, forming an i-motif structure that increases fluidity and accelerates drug release.79,80 For example, Sun et al.81 achieved an immunomodulatory effect using DNA nanogels to target lymph nodes with enhanced immune responses (Fig. 4A). Based on this research, such DNA structures allowed for effective lymph node accumulation, longer CpG retention in lymph nodes, and enhanced DC absorption capacity with increased immune activation both in vitro and in vivo. Significantly, immunization with an immunostimulatory DNA nanogel adjuvanted antigen induces a vigorous immune response, increasing the effectiveness of anticancer treatments. The easily constructed DNA nanogel offers a beautiful and valuable platform for coordinating the immune responses in lymph nodes. Significantly, the DNA nanogel immunomodulator shown here exemplifies a novel approach to adjuvant production that encourages the advancement of vaccines. Also, such an immunostimulatory DNA nanostructure has the potential to significantly increase the responsiveness to other immune treatments including immunogenic cell death (ICD) triggered therapy and immunological checkpoint blockers (ICBs).
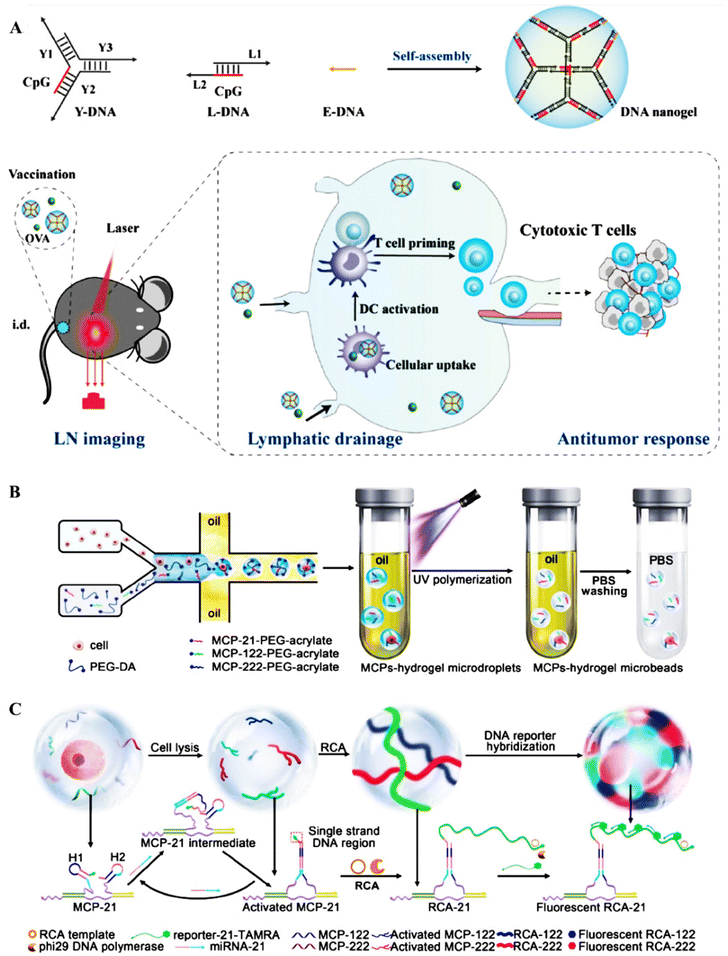 |
| Fig. 4 Schematic illustration of (A) self-assembled immunostimulatory DNA nanogels to target lymphoid organs and enhance the vaccine efficacy for amplified cancer immunotherapy81 Adapted from ref. 81 with permission from American Chemical Society, Copyright 2020. (B) single-cell encapsulation in microdroplets and the preparation of MCPs–hydrogel microbeads and (C) cell lysis and miRNA specific signal amplification in the hydrogel microbeads for single-cell multi-miRNA quantification.98 Adapted from ref. 98 with permission from the Royal Society of Chemistry, Copyright 2022. | |
4. Detection of cancer biomarkers
The National Cancer Institute (NCI) defines biomarkers as biological molecules in the blood, other body fluids, or tissues. Hence, biomarkers can indicate a normal or abnormal process, a condition, or a disease (NCI), for instance, cancer. An affected patient can usually be distinguished from a person without the disease using biomarkers.82,83 Various factors can lead to changes including germline or somatic mutations, transcriptional changes, and post-translational modifications. There are several types of biomarkers including proteins (e.g., enzymes or bioreceptors), nucleic acids (e.g., microRNA or other non-coding RNA), antibodies, and peptides, among other classifications. It is also possible for biomarkers to be a collection of alterations, like gene expression, proteomic, or metabolomic signatures.84,85 The presence of biomarkers can be identified via circulation (blood, serum, or plasma) or excretion (soil, urine, sputum, or nipple discharge); therefore, they can be tested non-invasively and continuously, or they can be detected using tissue samples, requiring biopsy or specific imaging for assessment. Moreover, genetic biomarkers can be inherited and identified as sequence differences in germ line DNA isolated from whole blood, sputum, or buccal cells or can be non-inherited and detected as variations in DNA derived from tumor tissue.86
In biosensing and biomedical applications, DNA hydrogels are well suited for biosensing and biomedical applications due to their stimuli-responsive properties, minimum environmental toxicity, and excellent biocompatibility. Another type of DNA nanogel material that has gained popularity is DNA microgels, which are colloidally stable hydrogels with a range of sizes from nanometers to micrometers. A DNA microgel exhibits much more rapid responses to stimuli than bulk hydrogels at the microscopic level, and greater permeability and retention are obtained as a result of the enhanced permeability of DNA microgels in tumors; the nanometer scale of DNA microgels makes them more readily capable of passively targeting internal cells and tissues.87–89 In addition, DNA nanogels can target cells actively through the recognition of biomarkers on goal cells when functionalized with DNA that can identify and bind targets, including aptamers. Biosensors, bioimaging, and controlled drug delivery can be achieved using DNA nanogels. Researchers have developed a variety of colorimetric visual sensors and readout devices for various biotargets, including metal ions and glucose, utilizing responsiveness differences in DNA hydrogels encapsulated with enzymes, DNAzymes, or catalytic nanomaterials.90–92 The 3D scaffold has abundant conjugation sites, so covalently crosslinked hydrogel networks can also be used as cellular immobilization matrices to design biosensing systems for DNA. Biomolecules can be selectively identified and extracted from biofluids using DNA aptamer-immobilized hydrogels, which may enable a reversible and efficient process of accumulation and release of biomolecules. Among the many advantages of immobilizing DNA within hydrogels are low DNA usage, specific molecular recognition capabilities for various chemical and biological targets, high loading capacity, low optical background, keeping DNA or enzymes in action and storing them in transparent hydrogel matrixes is simple and easy. High construction costs and long reaction times of these kinds of sensors, however, make them not suitable for all applications.93,94 Using functional nanomaterials, including nanozymes, it is possible to create susceptible DNA hydrogel biosensing systems, silver nanoclusters, and photonic crystals. Hydrogel scaffolds serve as stable supports and barriers for immobilizing nanomaterials as well as for immobilizing nanomaterials. By reducing macromolecule interference, these complex hydrogels can last much longer. By capturing cells during gelation, hydrogels are capable of isolating and enriching specific targets. Even though the cells are entrapped, they are still viable and can be released without damage. Functional DNA motifs have excellent biocompatibility, even permitting in situ sensing with gelation within cells.95,96 By using DNA hybridization to target hydrogel assembly, electrochemical sensors could be manufactured that employ DNA hydrogels to inhibit electron transfer with their hindrance effect.97 Wang et al.98 conducted research on this topic in 2022 by encapsulating single-cells in hydrogel microbeads to quantify multi-miRNA within single cells to differentiate different subpopulations of liver cancer cells, as illustrated in Fig. 4B and C, to create single cell encapsulated microdroplets, a flow-focusing microfluidic device with two aqueous phase inlets is used. The target miRNA responsive hairpin probe pairs H1/H2 and DNA nanowires are hybridized to form miRNA capture probes (MCPs). To distinguish between the normal liver cells HHL-5 and the liver cancer cell subpopulations HepG2, HCCLM3, and MHCC97L, miRNA-21, 122, and 222 are chosen as the target miRNAs. Although non-targeted nucleic acids and proteins are eliminated by PBS washing of the hydrogel microbeads, the released target miRNAs are identified by their matching MCPs and kept in the hydrogel microbeads. When the target miRNAs are recognized, the MCPs undergo structural changes that expose single-stranded DNA regions and trigger rolling circle amplification (RCA) reactions in the hydrogel microbeads. The RCA responses are repeatedly triggered by the target miRNA interactions with MCPs.
Several studies have been conducted in the area of cancer therapy and the methods involved are presented in Table 1.
Table 1 A review of recent studies on the application of DNA hydrogels for cancer therapy
DNA hydrogel |
Biomarker |
Type of cancer |
Model |
Lower LOD |
Detection principle |
Ref. |
Cross-linking hybridization chain reaction (c-HCR).
Circulating tumor cells.
|
DNAzyme hydrogel |
Using a smartphone-assisted pure DNAzyme hydrogel platform (colorimetry) |
Breast cancer, gastric cancer, lung cancer, & brain cancer |
E542K (a hotspot mutation in the PIK3CA gene) |
0.042 pM |
The concentration value could be transduced into an optical signal through the catalytic capability of the DNAzyme hydrogel, which can be easily recorded and quantified with the smartphone platform |
99
|
AFP-responsive DNA hydrogel |
SERS biosensor |
Hepatocellular carcinoma (HCC) |
AFP |
50 pg mL−1 |
In the presence of AFP, the AFP-aptamer can specifically recognize AFP and form a target–aptamer complex, which leads to the dissociation of the reticular structure of hydrogels and the release of IgG embedded in the hydrogels. Then, the released IgG combined with the antibody attached to the nanoprobes and capture probes |
100
|
Palindromic DNA hydrogel |
Using a DNA hydrogel (fluorescence) |
Breast cancer |
MiRNA-21 (MCF-7 & HeLa cells) |
5 pM |
The basic hairpin probes form dimers by hybridization between palindromes, and then different DNA hairpin-based dimers can autonomously interact with each other upon the stimuli of target miRNAs, finally forming cross-linked 2 h-DNA hydrogel networks (c-HCRa technique) |
88
|
CDs-chitosan nanocomposite DNA hydrogel |
Selective and sensitive DNA hydrogel (fluorescence) |
Breast cancer |
MiRNA-21 (MCF-7 cells) |
0.03 fM |
The specific interaction between the DNA-probe and target miRNA-21 resulted in incomplete quenching of the fluorescence of the CDs-chitosan nanocomposite hydrogels (about 67%) |
93
|
DNA hydrogel AgNPs-CuS NPs DNAzyme |
Analyte-triggered signal conversion, cascaded amplification via DNA hydrogel and DNAzyme (electrophoresis) |
Breast cancer |
CTCsb by liquid biopsy (MCF-7 cell) |
0.1 fg mL−1 |
The quadruple signal amplification strategy is based on biomaterials and nanomaterials with nanopore single-molecular sensing to significantly reduce the LOD and improve interference resistance |
95
|
CdSe/ZnS QDs–DNA/acrylamide hydrogel microcapsules |
QD loaded miRNA-responsive microcapsules |
Prostate cancer |
MiRNA-141 |
44.9 pM |
The hollow structure of DNA hydrogel microcapsules was loaded with fluorescent QDs as a readout signal. The bridged DNA in the microcapsule shell was designed in a caged sandwich-type structure and implanted with miRNA recognition elements |
96
|
5. DNA hydrogels and nanogels in cancer treatment
It is common practice to use small molecules to treat disease, particularly cancer therapy. Hydrogels made from DNA can be designed to physically entrap and chemically bind small molecules to enhance drug efficacy and eliminate side effects.38,94 Hydrogels perform differently in the delivery process due to their size, conformation, and component features. It will be discussed here how hydrogels are used for cancer treatment.
5.1. Chemotherapy
Cancer cells proliferate in your body, and chemotherapy uses powerful chemicals to kill them.101,102 Many types of cancer can be treated with chemotherapy drugs alone or in combination. According to research, chemotherapy is one of the most effective cancer treatments. The patients will inevitably experience mild or severe side effects as a result of this treatment method.103
The latest DNA nanotechnology can be used to construct DNA nanostructures with well-controlled size, shape, and surface chemistry based on the base pairing principle.104 Several advantages distinguish DNA nanostructure-based drug delivery systems from traditional drug delivery systems.105,106 For instance, Yan et al.107 in 2022 designed a nanohydrogel for drug delivery and microRNA self-regulation (Fig. 5). In Fig. 5A, a hydrophobic mesoporous silica nanoparticle (MSN) interacts with a DNA nanohydrogel shell to recognize miRNA. The DNA nanohydrogel may be employed as a gate of the pores of the MSN for targeted microRNA toehold self-regulating switches, thereby controlling the release of pharmaceuticals and concurrently silencing the targeted gene. The porous structure of MSN can achieve a high hydrophobic drug loading rate (Fig. 5C).
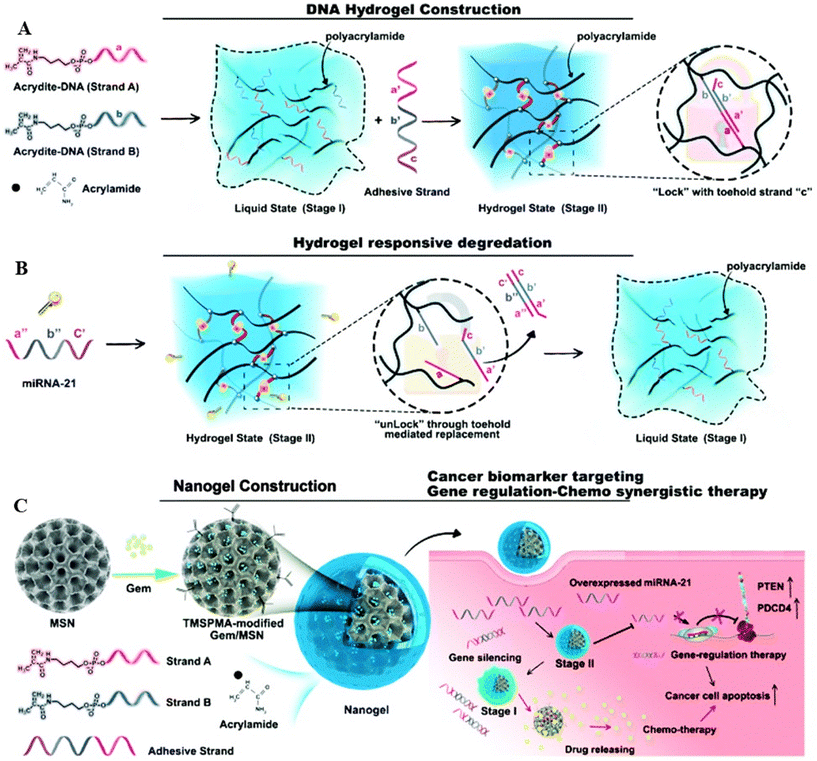 |
| Fig. 5 (A) Construction and (B) degradation mechanisms of the designed DNA hydrogel. (C) Combining the DNA hydrogel with MSNs for targeted gene regulation therapy.107 Adapted from ref. 107 with permission from the Royal Society of Chemistry, Copyright 2022. | |
DNA nanostructures can now be designed and built thanks to the advancement of nanotechnology. Drug carriers should generally meet several criteria to be considered ideal candidates. It is possible to load chemotherapeutic drugs onto the carrier with high efficiency and capacity. Furthermore, the carriers must remain stable during blood circulation so that the drugs can accumulate at the tumor site as long as possible. Also, the carriers must be well-suited for cellular uptake by having appropriate surface properties.108 Based on the driving force for the formation of nanostructures, drug–DNA nanostructures can be divided into several subcategories: chemical conjugation drives the formation of drug–SNA (spherical nucleic acid) nanostructures; hydrophobic interactions drive the formation of drug–DNA micelle nanostructures; base pairing is responsible for the formation of drug–aptamers, drug–DNA nanogels, drug–DNA cages, and drug–DNA origami nanostructures.109,110 As the driving force and the components being used in the construction (DNA sequences) of drug–DNA nanostructures differ in complexity, they can be highly simple or extremely complicated.111,112 An example of this is illustrated in Fig. 6A–C, which demonstrates a technique for producing nanogels that are structurally stable, architecturally well-defined, and functionally expandable so that therapeutics and imaging agents can be carried, as well as anticancer drugs can be released into living cells. Also, the atomic structure of DNA allows it to form duplex complementarity and three-/four-way junctions.113
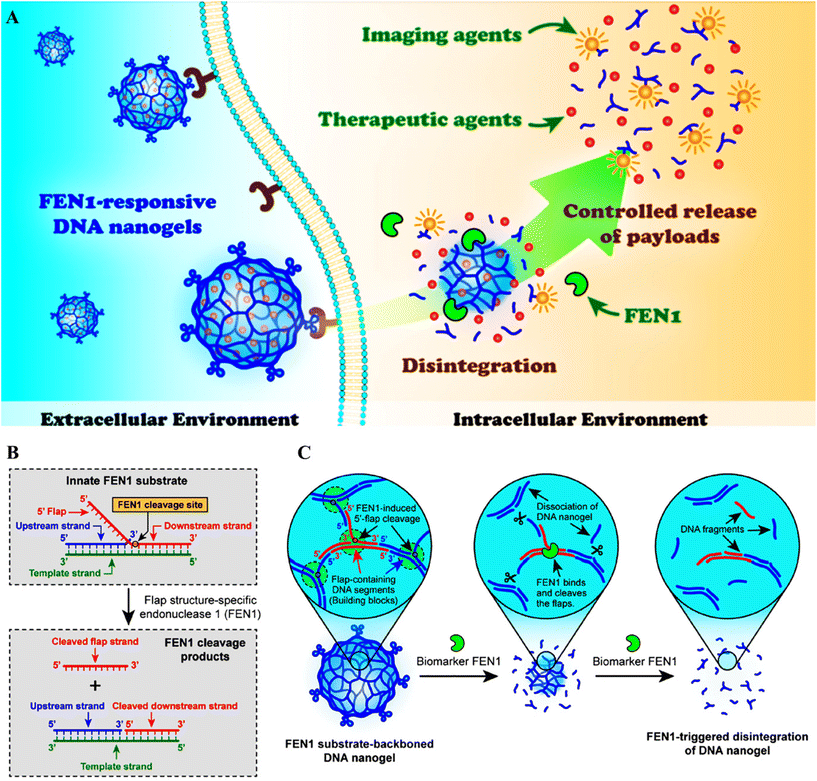 |
| Fig. 6 (A) Schematic illustration of cancer biomarker-triggered disintegrable DNA nanogels for intelligent drug delivery; (B) schematic illustration of the catalytic action of the substrate-backbone (FEN1) on its innate DNA substrates; (C) anticipated disintegration of our FEN1 substrate-backboned DNA nanogels by FEN1's catalytic activties.113 Adapted from ref. 113 with permission from American Chemical Society, Copyright 2020. | |
5.2. Gene therapy
Cells can be genetically modified by delivering DNA or RNA (e.g., small interfering RNA, siRNA), which can up-regulate or down-regulate genes. Viral delivery (viral gene therapy) or nonviral delivery (nonviral gene therapy) are methods of delivering genetic materials into cells by encapsulating or condensing plasmid DNA or siRNA into nanoparticles.38,114
Cancer, hemophilia, and viral infections can be treated using gene therapy, which provides a promising approach to both inherited and acquired diseases. Viral and nonviral vectors are currently the two main types of gene therapy vectors. Even though viral vectors are extensively used for effective gene transfer, their safety issues, such as immunogenicity and mutagenic toxicity, tend to make them highly risky.115 In response, nonviral vectors have become increasingly attractive and safer alternatives to viral vectors, leading to increased development of nonviral vectors. Nonviral vectors indeed have a much lower transfection efficiency than viral vectors, making their clinical application much more limited than that of viral vectors. As a result, it is paramount that safe nonviral vectors capable of efficient delivery and elevated therapeutics are developed. There have been several nonviral vectors developed over the past decade, such as liposomes, dendrimers, micelles, inorganic nanoparticles, DNA nanostructures, and polymeric nanoparticles (named nanohydrogels), and their gene delivery potential has been investigated.116,117 A wide variety of biotechnological and biomedical applications have been explored by DNA or RNA-functionalized hydrogels, including biosensors, controlled drug delivery, RNA interference, and tissue engineering, extending the application fields of hydrogels. The complex and labor-intensive modification steps associated with DNA–polymer hybrids have restricted this progress. Nevertheless, DNA hydrogels have limited biomedical applications due to their bulky size and ineffective release mechanisms.118
There has been recent evidence that antisense oligonucleotides and microRNA (miRNA) can modulate gene expression. Recently, the most popular strategy in gene therapy has been to supplement endogenous gene down-regulation and to silence highly expressed pathogenic genes.119 Nucleases break dsDNA to induce DNA insertions and deletions at specific genomic loci according to its mechanism. Cas (CRISPR-associated) toolbox was previously one of the most significant breakthroughs for gene editing since the development of CRISPR (clustered regularly interspaced short palindromic repeats). Cas proteins and guide RNAs, both biomolecules, comprise this toolbox. Cas protein and gRNA form a complex after binding together.120–122
5.3. Phototherapy
Due to its minimal invasiveness, high efficacy, and low side effects, phototherapy, especially photothermal therapy and photodynamic therapy, is a promising therapeutic technique to treat skin cancer.123 Due to these limitations, the combined application of photothermal therapy (PTT) and photodynamic therapy (PDT) has frequently been documented; however, PTT and PDT often require photoagents and excitation light, making them difficult to use clinically.124,125
In recent years, PTT has attracted increasing attention as a controllable and emerging antitumor technique. The tumor site is hyperthermic using photothermal convertible agents under near-infrared (NIR) irradiation, which as a consequence results in tumor ablation. The photothermal conversion efficiency of photothermal convertible materials for PTT treatment has reached a significant level that creates a satisfactory thermal ablation temperature (more than 50 °C) at the tumor site.126,127
By oxidative damage caused by reactive oxygen species (ROS), such as singlet oxygen (1O2), PDT causes cancer cells to die, and ROS are generated under laser irradiation by photosensitizers. PDT is a potential therapeutic route in cancer therapy because of its advantages, including a rapid cure, minimal or non-invasiveness, and circumvention of drug resistance.128 Since photosensitizers are hydrophobic organic molecules, they are always poorly soluble in blood, resulting in low drug delivery efficiency and limited yields of 1O2, which reduce the effectiveness of PDT. Additionally, hypoxic tumor microenvironments reduce O2 supply, which weakens the therapeutic effect of PDT. Recent research has highlighted the value of DNA materials with programmable sequences, good biocompatibility, and the ability to design new structures.129–131
Additionally, DNA hydrogels can improve the penetration of nanoparticles into cells and reduce the risk of cancer by combining them with phototherapy.132 For instance, to treat breast cancer through phototherapy, Wang et al.133 introduced DNA-based hydrogels with Au nanoparticles or Au nanorods in 2019. In this article, gold nanostructures with the LSPR effect were used for cancer therapy with doxorubicin. In addition, this study has provided insight into DNA-based hydrogels containing AuNPs or AuNRs as functional, thermoresponsive plasmonic materials that exhibit shape memory, self-healing, controlled release, and mechanical properties (Fig. 7A–C). The dehybridization of the DNA duplexes and creation of the hydrogels in low-stiffness states were accomplished by photoirradiation of the AuNP- or AuNR-loaded hydrogels, which resulted in the wavelength-selective, plasmonic heating of the hydrogels. The hydrogels regained the rigid forms that are stabilized by two cross-linking motifs, such as bis-acrylamide/duplexes or boronate ester–glucosamine/duplexes, after turning off the light source.
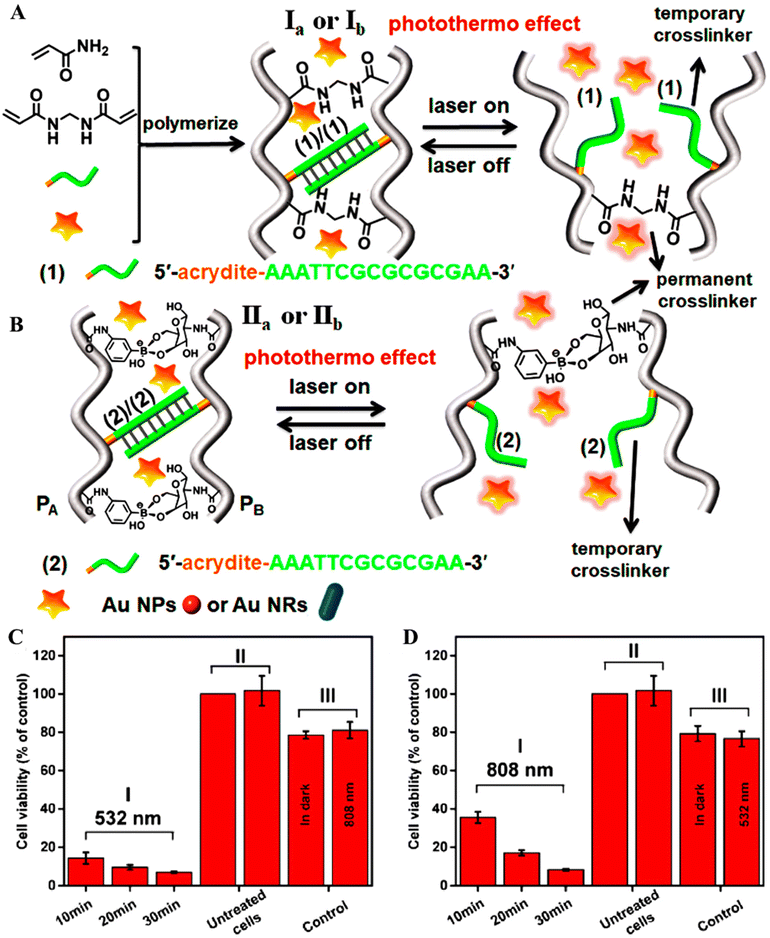 |
| Fig. 7 Preparation and light-induced thermoresponsive stiffness control of AuNP-loaded (Ia and IIa) or AuNR-loaded (Ib and IIb) hydrogels crosslinked cooperatively by bis-acrylamide and nucleic acid duplexes (1)/(1) (A) or boronate ester–glucosamine and nucleic acid duplexes (2)/(2) (B), respectively. The cytotoxicity of the Dox/AuNP- and Dox/AuNR-loaded hydrogels toward MDA-MB-231 breast cancer cells. The bars represent the cell viability after the treatment of the cells with Dox-loaded hydrogels for 3 days. (C) Entry I: cells treated with the Dox/AuNP-loaded hydrogel Ia irradiated with a 532 nm light source. Entry II: untreated cells in growth media; untreated cells in growth media and HEPES buffer. Entry III: control experiments, where the cells were treated with Dox/AuNP-loaded hydrogel Ia in the dark or upon irradiation with an 808 nm light source for 30 min. (D) Entry I: cells treated with the Dox/AuNR-loaded hydrogel Ib irradiated with the 808 nm light source. Entry II: untreated cells in growth media; untreated cells in growth media and HEPES buffer. Entry III: control experiments where the cells were treated with Dox/AuNRs-loaded hydrogel Ib in the dark or upon irradiation with a 532 nm light source for 30 min.133 Adapted from ref. 133 with permission from American Chemical Society, Copyright 2019. | |
5.4. Cancer immunotherapy
Based on the immunotherapy method, the cancer cells are destroyed by the body's immune system. Various types of immunotherapies exist, including immunotherapy that blocks immune checkpoints, immunotherapy that delivers tumor vaccines, and photothermal immunotherapy that delivers radiation to the tumor. Different fields have explored hydrogels with 3D network structures. Many studies have reported the use of hydrogels as cancer treatment and tissue engineering materials because of their unique porosity, swelling properties, mechanical properties, and biocompatibility.134–136
Furthermore, some intelligent hydrogels have attracted significant attention due to their ability to release therapeutic agents when activated by external stimuli (such as light and magnetic fields) or endogenous stimuli (such as redox, pH, ionic strength, or enzymes). Polymeric hydrogels have been recently used for cancer immunotherapy to deliver targeted drugs precisely timed and spatially located.137,138 The development of polymer-based hydrogels for the local delivery of immunotherapeutic agents for cancer immunotherapy has received considerable attention in recent years. Numerous immunotherapeutic agents, chemotherapeutic drugs, photosensitizers, and combinations of the three can be incorporated into polymer-based hydrogels to facilitate sole immunotherapy, combinational chemo-immunotherapy, and photo-immunotherapy.139,140
5.5. Combination therapy
In the progression of these cancers in a cell line, many mutations occur successively, which is the hallmark of nanoparticle-based combination therapy. Furthermore, tumors can be challenging. A variety of chemotherapeutic agents are intrinsically resistant and have acquired resistance due to mutations favorable for tumor survival as chemotherapy progresses. A single drug may not be able to reduce tumors by inhibiting a pathway. When a single drug is combined with a pathway, it may not be sufficient to achieve tumor regression.141–143 Using a combination chemotherapy strategy, two (or more) agents working against the same cancer path, gene, or cell cycle checkpoint are combined with increasing its chance of being eliminated. Oncologists can reduce cytotoxic effects by combining chemotherapeutic medications, which increase efficacy while reducing cytotoxic effects. The combination treatment results in better responses and better survival than single-agent treatment in practice.144–146 In a case study of combination therapy using DNA hydrogels, Tang et al.147 have reported the generation of aptamer-modified DNA tetrahedron (TET)-based nanogels for combination (chemo/gene) therapy of multidrug-resistant cancers. According to this study, functional DNA-based nanogels were designed to facilitate targeted internalization of embedded antisense oligonucleotides (ASOs) and stimulation-responsive release of embedded ASOs with the incorporation of an active cell-targeting group (aptamer in one vertex of TET) and a controlled-release element (disulfide bridges in ASO terminals). As a result, combined chemo/gene therapy produced an impressive antitumor effect in Dox-resistant MCF-7R breast cancer cells (Fig. 8A–C). Moreover, Song et al.145 reported an intelligent DNA nanohydrogel capable of targeting cancer cells, controlling their size, adjusting the pH, and releasing glucose oxidase (GOx). The drug carriers used in this study were prepared by encapsulating GOx within DNA nanohydrogels and modifying the DNA linker with ferrocene (Fc). Ultimately, DNA nanohydrogels showed significant apoptosis and tumor suppression capabilities both in vitro and in vivo (Fig. 8D–F).
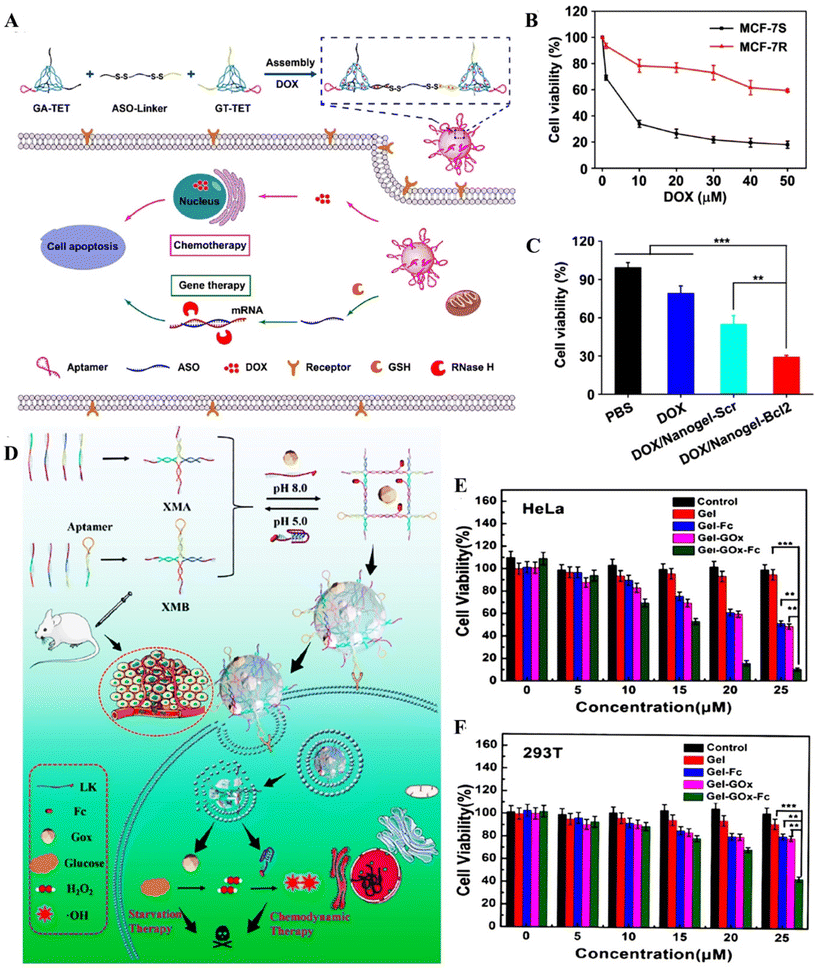 |
| Fig. 8 (A) An aptamer-modified DNA tetrahedron-based nanogel for efficient combined chemo/gene therapy of multidrug-resistant tumors; (B) cell viability analysis of Dox-resistant and Dox-sensitive breast tumor cells (MCF-7R and MCF-7S); (C) cell viability of MCF-7R with different treatments for 48 h.147 Adapted from ref. 147 with permission from American Chemical Society, Copyright 2021. (D) Schematic illustration of a self-assembled DNA nanohydrogel and application of the antitumor combination therapy, and the cytotoxicity of DNA nanohydrogels was estimated using the CCK-8 assay, (E) HeLa cells and (F) 293T cells incubated with different concentrations of DNA nanohydrogels.145 Adapted from ref. 145 with permission from American Chemical Society, Copyright 2021. | |
A number of studies have been conducted in the area of cancer therapy and the methods involved are presented in Table 2.
Table 2 A review of recent studies on the application of DNA hydrogels for cancer therapy
Treatment method |
DNA–hydrogel |
Target |
Delivery route |
Cell model (in vitro) |
Cancer model (in vivo) |
Treatment effect |
Ref. |
Black phosphorus quantum dots (BPQDs).
Dendritic mesoporous organosilica nanoparticles (DMONs).
|
Chemotherapy |
DNA–DOX NGs |
Cancer therapy |
— |
A549, BEAS-2B, H1299 & LL2 cells |
— |
The environmentally-friendly DNA NGs with pH-triggered/GSH-triggered anticancer effects can potentially be used as efficient carriers (>90%) in the targeting of cancer cells |
101
|
MB–Dox hydrogel |
Ovarian carcinoma therapy |
Injection |
SKOV3-luc cells |
SKOV3-luciferase intraperitoneal murine model |
The MB–Dox hydrogel slowed down the rate of the murine intraperitoneal tumor growth 72.2% more than free Dox |
105
|
RNA-triple-helix hydrogel |
Treatment of triple negative breast cancers (TNBCs) |
Injection |
MDA–MB-231, MCF-7 & HeLa cells |
MDA–MB-231 tumor-bearing BALB/c nude mice |
RNA-triple-helix hydrogel provides a potential specific treatment to TNBCs with high selectivity (this hydrogel can clear tumors of about ∼60 mm3) |
109
|
AAL-nanogel |
Breast and Liver cancer therapy |
— |
MCF-7 & L02 cells |
— |
This nanohydrogel realized specific cancer cell killing capabilities due to different intracellular ATP levels in normal and cancer cell lines |
110
|
Ade-FFF hydrogel |
Breast cancer therapy |
Injection |
4T1 cells |
Tumor-bearing BALB/c mouse model |
Ade-FFF hydrogel can clear tumor of about ∼100 mm3 |
111
|
Peptide-modified erythrocyte membrane-coated nucleic hydrogel nanoparticle (Vir-Gel) |
Glioblastoma therapy |
Injection |
Raw264.7 & GL261 cells |
Glioma-bearing C57/BL6 mice |
Vir-Gel had active tumor-targeting capability and excellent tumor inhibition efficacy |
112
|
Collagen/silk fibroin/polydopamine + thrombin-loaded (BSDA + th hydrogel) |
Breast cancer therapy |
Injection |
4T1 cells |
Tumor-bearing BALB/c mice |
The in vitro and in vivo results have demonstrated the efficacy (∼99%) of this DNA-hydrogels in nutrition deprivation for preventing cancer recurrence and metastasis |
148
|
Phototherapy |
PD-L1/PPA-NG |
B16 melanoma model |
Injection |
B16–F10 cells |
Six- to 7-week-old C57BL/6 female mice |
The DNA gel can clear tumor of about ∼100 mm3 |
128
|
DNA-grafted polycaprolactone (DNA-g-PCL) |
The cells from Lacks's cancerous cervical tumor |
Injection |
L929 cells |
The HeLa tumor-bearing nude mice |
The DNA gel can clear tumor of about ∼100 mm3 |
129
|
DOX/PEI@BPQDsa–DNA gel |
Breast cancer tumor tissue |
Injection |
MCF-7 cells |
Mouse models |
The DNA gel can clear large clinical tumors of about ∼500 mm3 |
130
|
DNA–inorganic hybrid hydrogels (DNA–UCNP–Au) |
Malignant bladder cancer therapy |
Injection |
T24 cells |
Female BALB/c nude mice bearing T24 tumors |
A photothermal efficiency as high as 42.7% is realized in the hydrogel. The tumors (∼100 mm3) are eradicated and no recurrence is observed |
131
|
Combination therapy |
RNA transcriptions/FAM–S6APT–CHOL (RNA nano-hydrogels, RNA NHs) |
Lung cancer therapy |
Injection |
A549 cells |
A549 tumor-bearing mouse |
Co-delivery of three types of miRNAs realizes gene silencing for both pump and non-pump resistance of chemotherapy at the same time. (The RNA nano-gel can clear tumor of about ∼100 mm3) |
143
|
DMONsb/DOX@DNA |
Breast tumor therapy |
— |
MCF-7/ADR cells |
— |
A significant anti-tumor therapeutic effect was achieved by overcoming MDR in tumor cells |
149
|
131I-hydrogel/DOX/GNP aggregates |
Breast tumor therapy |
Injection |
MCF-7 |
MCF-7-luciferase tumor-bearing mice |
The hybrid hydrogel not only inhibits self-repair of the damaged DNA but also effectively relieves tumor hypoxic conditions |
150
|
MXene–DNA hydrogel |
Cervical cancer treatment |
Injection |
HeLa cells |
BALB/c female nude mice |
The MXene–DNA hydrogel system exhibited highly efficient localized cancer treatment with fewer side effects to the organisms, which was attributed to the synergistic effect from the photothermal tumor damage, highly efficient localized release of chemotherapeutic agents, and the enhanced chemotherapy effect at mild hyperthermia conditions upon NIR irradiation |
151
|
Gene therapy |
G5–BGG/pDNA |
Glioblastoma treatment |
Injection |
U87MG cells |
Orthotopic U87MG tumor-bearing mice |
G5–BGG/shRNA871-loaded hydrogels combined with temozolomide downregulated CD47 protein expression, increased macrophage infiltration into residual tumors, and significantly prolonged the survival time of mice, indicating potential applications for glioblastoma treatment |
121
|
siPLK1–DNA nanohydrogel |
Liver cancer therapy |
Injection |
Human liver (L02) cells |
HeLa cell-bearing mice |
The telomerase-activated DNA nanogel enabled simultaneous in vitro telomerase imaging and in vivo antitumor gene therapy with minimal systemic toxicity |
122
|
Gem@MSN@DNA |
Oncogene of pancreatic cancer treatment |
— |
pancreatic cancer cell |
— |
The up-regulation of the expression of tumor suppressor genes PTEN and PDCD4 with Gem@MSN@DNA |
107
|
Immunotherapy |
CpGgel–siSTAT3 |
Synergistic cancer immunotherapy |
Injection |
RAW264.7 cells |
Male C57BL/6 mice |
After CpGgel–siSTAT3 uptake, the macrophage is activated through TLR9–MyD88 signaling. Meanwhile, siSTAT3 released from the nanogel will greatly inhibit the STAT3 expression, resulting in the stagnation of tumor progression |
135
|
Cas9/sgRNA hydrogel |
Lung cancer immunotherapy |
Injection |
B16F10 melanoma cells |
B16F10 tumor-bearing C57BL mice |
The hydrogel exhibits prolonged retention at tumor sites in vivo, while its gradual Cas9-mediated liberation of PD-1 aptamers inhibits the activity of immune cells in the tumor microenvironment |
140
|
FAM–RDgel |
Colorectal cancer immunotherapy |
— |
RAW264.7 & mouse dendritic DC2.4 cells |
— |
The RDgel constructed using DNA nanotechnology can be a useful adjuvant in cancer therapy with sustained RNA release and high immunostimulatory activity |
152
|
6. Theragnostic applications
The development of novel technologies for disease diagnosis and treatment has contributed to the rapid development of medicine and health worldwide. To improve the treatment effectiveness and survival rates, it is crucial to diagnose the disease at the earliest stage. Therefore, advanced diagnostic techniques and treatments are highly needed to ensure patient safety.153,154 A theragnostic nanosystem usually contains multifunctional components by including at least an imaging and a therapeutic compartment. When administered, the therapeutic component provides effective disease treatment. A visual component can give real-time feedback on drug delivery, release, accumulation, and pharmacokinetics, enabling the patient's medical treatment to be monitored and optimized rationally throughout the entire treatment.155,156 Recently, DNA hydrogels have shown significant promise as stimuli-responsive drug delivery platforms. The DNA hydrogel can adapt to various environments due to its programmability. Developing DNA hydrogels with tumor marker-responsive properties can improve the specificity and efficacy of treatment strategies.157 For example, Leo and co-workers showed cell-size structures of DNA–gel developed under culture conditions and their effects on DNA–gel. One of the peculiarities is that a dense (gel) and thin layer coexist in this structure, resulting from phase separation and loose regions are generated in the process. The results of the study also showed that different cell lines responded to the treatment differently, with varying degrees of pronounced effects. These observations may be helpful in future applications.158 Also Li and the other co-workers developed an activatable DNA nanohydrogel for the detection and treatment of cancer cells with targeted imaging. In this case, SDHs were easily prepared using three types of DNA self-assembly. There are many advantages of streptavidin-based DNA tetrads, including good biocompatibility and size-controllability, and easy to use.159 In another study, Xu and co-workers developed drug carriers capable of sensing tumor markers within the tumor microenvironment and responding to cancer therapy, and self-assembled adenosine triphosphate (ATP)-responsive AAL nanogels have been used to visualize cancer cells using specific fluorescence imaging and treat them with chemotherapies.110
7. Conclusion and prospects
DNA-based hydrogels, as an efficient class of soft drug delivery systems, have gained significant attention because of several advantages. In particular, DNA nanogels that are 3D scaffolds at the nanoscale have attracted more attention. As the most crucial feature, DNA hydrogels are programmable within the treatment process of cancerous genes. Herein, a collection of information about the design and preparation methods of DNA hydrogels and other functions in detecting biomarkers is given. In addition, a logical classification for the applications of DNA hydrogels in the area of advanced drug and gene therapy has been made in this report. As the main challenge of DNA hydrogels, their mechanical stability in the real physiological environment is quite low; therefore much effort has been made to increase the strength and half-life of the DNA hydrogels in blood serum. In this regard, the capability of DNA hydrogels for functionalization with the other active ingredients is considered another advantage of this species. In this overview, the achievements made by the researchers in the field of DNA hydrogels and nanogels are quickly reviewed to give valid support for further endeavors. The hopes are tied to further studies on DNA hydrogels to resolve the challenges and lead this substantial natural species to reach the market of gene therapy for cancer diseases.
Abbreviations
ABIs | Active biological ingredients |
ADR | Adriamycin |
AFP | α-Fetoprotein |
ATP | Adenosine triphosphate |
BPQDs | Black phosphorus quantum dots |
CDs | Carbon dots |
cfDNA | Cell-free DNA |
CTCs | Circulating tumor cells |
CpG | Cytosine–phosphate–guanine |
c-HCR | Cross-linking hybridization chain reaction |
CRISPR | Clustered regularly interspaced short palindromic repeats |
DMONs | Dendritic mesoporous organosilica nanoparticles |
DNA | Deoxyribonucleic acid |
Dox | Doxorubicin |
Gem | Gemcitabine |
AuNPs | Gold nanoparticles |
AuNRs | Gold nanorods |
LOD | Limit of detection |
DMON | Mesoporous organosilica nanoparticle |
MSNs | Mesoporous silica nanoparticles |
MB | Methylene blue |
miRNA | microRNA |
MDR | Multidrug resistance |
NIR | Near-infrared |
PDT | Photodynamic therapy |
PTT | Photothermal therapy |
PD-1 | Programmed death receptor |
QDs | Quantum dots |
ROS | Reactive oxygen species |
RDgel | RNA/DNA hydrogel |
RCA | Rolling circle amplification |
SDHs | Scaffolded DNA nanohydrogels |
siRNA | Small interfering RNA |
sgRNA | Single guide RNA |
1O2 | Singlet oxygen |
SERS | Surface-enhanced Raman scattering |
3D | Three-dimensional |
TLR | Toll-like receptor |
TNBCs | Triple-negative breast cancers |
Author contributions
I. Z. proposed the hypothesis. R. T. L. managed the work, wrote the Introduction section, reviewed the document, and drew the graphical abstract. F. E. wrote some sections of the manuscript and revised the whole document. M. M. S. wrote some sections of the manuscript. A. M. wrote a section of the manuscript. A. M. revised some sections of the manuscript. E. M. reviewed and revised the manuscript and submitted the work.
Conflicts of interest
The authors declare no conflict of interest.
References
- M. A. Shahbazi, T. Bauleth-Ramos and H. A. Santos, Adv. Ther., 2018, 1, 1800042 CrossRef
.
- X. Mao, D. Mao, T. Chen, M. Jalalah, M. S. Al-Assiri, F. A. Harraz, X. Zhu and G. Li, ACS Appl. Mater. Interfaces, 2020, 12, 36851–36859 CrossRef CAS PubMed
.
- M. Puccetti, M. Pariano, G. Renga, I. Santarelli, F. D'onofrio, M. M. Bellet, C. Stincardini, A. Bartoli, C. Costantini, L. Romani, M. Ricci and S. Giovagnoli, Cells, 2021, 10, 1601 CrossRef CAS PubMed
.
- T. C. Roberts, R. Langer and M. J. A. Wood, Nat. Rev. Drug Discovery, 2020, 19, 673–694 CrossRef CAS PubMed
.
- W. Yang, H. Veroniaina, X. Qi, P. Chen, F. Li and P. C. Ke, Adv. Ther., 2020, 3, 1900102 CrossRef PubMed
.
- J. Tang, C. Yao, Z. Gu, S. Jung, D. Luo and D. Yang, Angew. Chem., Int. Ed., 2020, 59, 2490–2495 CrossRef CAS PubMed
.
- Y. Dong, C. Yao, Y. Zhu, L. Yang, D. Luo and D. Yang, Chem. Rev., 2020, 120, 9420–9481 CrossRef CAS PubMed
.
- T. Yuan, Y. Shao, X. Zhou, Q. Liu, Z. Zhu, B. Zhou, Y. Dong, N. Stephanopoulos, S. Gui, H. Yan and D. Liu, Adv. Mater., 2021, 33, 2102428 CrossRef CAS PubMed
.
- D. W. Conroy, Y. Xu, H. Shi, N. G. Salguero, R. N. Purusottam, M. D. Shannon, H. M. Al-Hashimi and C. P. Jaroniec, Proc. Natl. Acad. Sci. U. S. A., 2022, 119, e2200681119 CrossRef CAS PubMed
.
- L. Slocombe, J. S. Al-Khalili and M. Sacchi, Phys. Chem. Chem. Phys., 2021, 23, 4141–4150 RSC
.
- R. Taheri-Ledari, F. Ganjali, S. Zarei-Shokat, M. Saeidirad, F. Ansari, M. Forouzandeh-Malati, F. Hassanzadeh-Afruzi, S. M. Hashemi and A. Maleki, Energy Fuels, 2022, 36, 10702–10720 CrossRef CAS
.
- R. Taheri-Ledari, S. Gharibi, A. Maleki, S. Akin and A. E. Shalan, Energy Technol., 2023, 11, 2100936 CrossRef CAS
.
- K. Valadi, S. Gharibi, R. Taheri-Ledari, S. Akin, A. Maleki and A. E. Shalan, Environ. Chem. Lett., 2021, 19, 2185–2207 CrossRef CAS
.
- R. Taheri-Ledari, S. M. Hashemi and A. Maleki, RSC Adv., 2019, 9, 40348–40356 RSC
.
- S. S. Soltani, R. Taheri-Ledari, S. M. F. Farnia, A. Maleki and A. Foroumadi, RSC Adv., 2020, 10, 23359–23371 RSC
.
- A. Maleki, R. Taheri-Ledari and R. Ghalavand, Comb. Chem. High Throughput Screening, 2020, 23, 119–125 CrossRef CAS PubMed
.
- F. Hassanzadeh-Afruzi, Z. Amiri-Khamakani, M. Saeidirad, M. M. Salehi, R. Taheri-Ledari and A. Maleki, RSC Adv., 2023, 13, 10367–10378 RSC
.
- F. Esmailzadeh, F. Hassanzadeh-Afruzi, H. Dogari and A. Maleki, Mater. Sci. Eng., B, 2023, 292, 116420 CrossRef CAS
.
- R. Taheri-Ledari, F. R. Asl, M. Saeidirad, A. Kashtiaray and A. Maleki, Sci. Rep., 2022, 12, 1–14 CrossRef PubMed
.
- W. Zhang, R. Taheri-Ledari, M. Saeidirad, F. S. Qazi, A. Kashtiaray, F. Ganjali, Y. Tian and A. Maleki, J. Environ. Chem. Eng., 2022, 10, 108836 CrossRef CAS
.
- F. Hassanzadeh-Afruzi, F. Esmailzadeh, S. Asgharnasl, F. Ganjali, R. Taheri-Ledari and A. Maleki, Sep. Purif. Technol., 2022, 291, 120956 CrossRef CAS
.
- R. Taheri-Ledari, M. R. Ahghari, F. Ansari, M. Forouzandeh-Malati, S. S. Mirmohammadi, S. Zarei-Shokat, S. Ramezanpour, W. Zhang, Y. Tian and A. Maleki, Nanoscale Adv., 2022, 4, 4418–4433 RSC
.
- V. Soltaninejad, M. R. Ahghari, R. Taheri-Ledari, A. Maleki and A. E. Shalan, J. Mol. Struct., 2022, 1256, 132456 CrossRef CAS
.
- R. Taheri-Ledari, F. Jalali, L. Heidari, F. Ganjali, F. R. Asl, S. Zarei-Shokat, M. Forouzandeh-Malati, A. Mohammadi and A. Maleki, RSC Adv., 2022, 12, 35383–35395 RSC
.
- F. Hassanzadeh-Afruzi, G. Ranjbar, M. M. Salehi, F. Esmailzadeh and A. Maleki, Sep. Purif. Technol., 2023, 306, 122700 CrossRef CAS
.
- F. Hassanzadeh-Afruzi, F. Esmailzadeh, G. Heidari, A. Maleki and E. Nazarzadeh Zare, ACS Omega, 2023, 8.7, 6337–6348, DOI:10.1021/ACSOMEGA.2C06555
.
- R. Taheri-Ledari, N. Tarinsun, F. Sadat Qazi, L. Heidari, M. Saeidirad, F. Ganjali, F. Ansari, F. Hassanzadeh-Afruzi and A. Maleki, Inorg. Chem., 2023, 62.6, 2530–2547, DOI:10.1021/ACS.INORGCHEM.2C02634
.
- R. Taheri-Ledari, A. Fazeli, A. Kashtiaray, S. Salek Soltani, A. Maleki and W. Zhang, Langmuir, 2022, 38, 132–146 CrossRef CAS PubMed
.
- R. Taheri-Ledari, E. Zolfaghari, S. Zarei-Shokat, A. Kashtiaray and A. Maleki, Commun. Biol., 2022, 5, 1–16 CrossRef PubMed
.
- R. Taheri-Ledari, W. Zhang, M. Radmanesh, N. Cathcart, A. Maleki and V. Kitaev, J. Nanobiotechnol., 2021, 19, 1–21 CrossRef PubMed
.
- R. Taheri-Ledari, W. Zhang, M. Radmanesh, S. S. Mirmohammadi, A. Maleki, N. Cathcart and V. Kitaev, Small, 2020, 16, 2002733 CrossRef CAS PubMed
.
- J. Su, S. Lu, S. Jiang, B. Li, B. Liu, Q. Sun, J. Li, F. Wang and Y. Wei, Adv. Mater., 2021, 33, 2100619 CrossRef CAS PubMed
.
- S. Kumar and A. Bajaj, Biomater. Sci., 2020, 8, 2055–2073 RSC
.
- V. Morya, S. Walia, B. B. Mandal, C. Ghoroi and D. Bhatia, ACS Biomater. Sci. Eng., 2020, 6, 6021–6035 CrossRef CAS PubMed
.
- F. Li, D. Lyu, S. Liu and W. Guo, Adv. Mater., 2020, 32, 1806538 CrossRef CAS PubMed
.
- Y. Hou, R. Han, Y. Sun, C. Luo and X. Wang, Anal. Chim. Acta, 2022, 1195, 339386 CrossRef CAS PubMed
.
- Z. Li, J. Huang and J. Wu, Biomater. Sci., 2021, 9, 574–589 RSC
.
- F. Mo, K. Jiang, D. Zhao, Y. Wang, J. Song and W. Tan, Adv. Drug Delivery Rev., 2021, 168, 79–98 CrossRef CAS PubMed
.
- R. Xing, Y. Liu, Q. Zou and X. Yan, Nanoscale, 2019, 11, 22182–22195 RSC
.
- Y. Guo, J. Tang, C. Yao and D. Yang, Wiley Interdiscip. Rev.: Nanomed. Nanobiotechnol., 2022, 14, e1753 CAS
.
- P. Kumar, B. Liu and G. Behl, Macromol. Biosci., 2019, 19, 1900071 CrossRef PubMed
.
- S. Bernhard and M. W. Tibbitt, Adv. Drug Delivery Rev., 2021, 171, 240–256 CrossRef CAS PubMed
.
- S. Basu, S. Pacelli and A. Paul, Acta Biomater., 2020, 105, 159–169 CrossRef CAS PubMed
.
- F. Sabir, M. Zeeshan, U. Laraib, M. Barani, A. Rahdar, M. Cucchiarini and S. Pandey, Cancers, 2021, 13, 3396 CrossRef CAS PubMed
.
- B. Yang, B. Zhou, C. Li, X. Li, Z. Shi, Y. Li, C. Zhu, X. Li, Y. Hua, Y. Pan, J. He, T. Cao, Y. Sun, W. Liu, M. Ge, Y. R. Yang, Y. Dong and D. Liu, Angew. Chem., Int. Ed., 2022, 61, e202202520 CAS
.
- F. Li, J. Tang, J. Geng, D. Luo and D. Yang, Prog. Polym. Sci., 2019, 98, 101163 CrossRef CAS
.
- H. A. Assi, M. Garavís, C. González and M. J. Damha, Nucleic Acids Res., 2018, 46, 8038–8056 CrossRef CAS PubMed
.
- S. Rhee, Z. J. Han, K. Liu, H. T. Miles and D. R. Davies, Biochemistry, 1999, 38, 16810–16815 CrossRef CAS PubMed
.
- M. Esguerra, L. Nilsson and A. Villa, Nucleic Acids Res., 2014, 42, 11329–11338 CrossRef CAS PubMed
.
- J. Spiegel, S. Adhikari and S. Balasubramanian, Trends Chem., 2020, 2, 123–136 CrossRef CAS PubMed
.
- M. Guéron and J. L. Leroy, Curr. Opin. Struct. Biol., 2000, 10, 326–331 CrossRef PubMed
.
- W. Guo, C. H. Lu, X. J. Qi, R. Orbach, M. Fadeev, H. H. Yang and I. Willner, Angew. Chem., Int. Ed., 2014, 53, 10134–10138 CrossRef CAS PubMed
.
- E. Hasuike, A. M. Akimoto, R. Kuroda, K. Matsukawa, Y. Hiruta, H. Kanazawa and R. Yoshida, Chem. Commun., 2017, 53, 3142–3144 RSC
.
- J. L. Huppert and S. Balasubramanian, Nucleic Acids Res., 2007, 35, 406–413 CrossRef CAS PubMed
.
- H. K. Lau and K. L. Kiick, Biomacromolecules, 2015, 16, 28–42 CrossRef CAS PubMed
.
- S. Nagahara and T. Matsuda, Polym. Gels Networks, 1996, 4, 111–127 CrossRef CAS
.
- L. Zhou, X. Jiao, S. Liu, M. Hao, S. Cheng, P. Zhang and Y. Wen, J. Mater. Chem. B, 2020, 8, 1991–2009 RSC
.
- W. Guo, C. H. Lu, R. Orbach, F. Wang, X. J. Qi, A. Cecconello, D. Seliktar and I. Willner, Adv. Mater., 2015, 27, 73–78 CrossRef CAS PubMed
.
- C. Li, A. Faulkner-Jones, A. R. Dun, J. Jin, P. Chen, Y. Xing, Z. Yang, Z. Li, W. Shu, D. Liu and R. R. Duncan, Angew. Chem., 2015, 127, 4029–4033 CrossRef
.
- S. H. Um, J. B. Lee, N. Park, S. Y. Kwon, C. C. Umbach and D. Luo, Nat. Mater., 2006, 5, 797–801 CrossRef CAS PubMed
.
- Y. Xing, E. Cheng, Y. Yang, P. Chen, T. Zhang, Y. Sun, Z. Yang and D. Liu, Adv. Mater., 2011, 23, 1117–1121 CrossRef CAS PubMed
.
- J. B. Lee, S. Peng, D. Yang, Y. H. Roh, H. Funabashi, N. Park, E. J. Rice, L. Chen, R. Long, M. Wu and D. Luo, Nat. Nanotechnol., 2012, 7, 816–820 CrossRef CAS PubMed
.
- Y. Huang, W. Xu, G. Liu and L. Tian, Chem. Commun., 2017, 53, 3038–3041 RSC
.
- W. Xu, Y. Huang, H. Zhao, P. Li, G. Liu, J. Li, C. Zhu and L. Tian, Chem. – Eur. J., 2017, 23, 18276–18281 CrossRef CAS PubMed
.
- A. Bacolla, G. Wang and K. M. Vasquez, PLoS Genet., 2015, 11, e1005696 CrossRef PubMed
.
- S. van de Wall, K. C. M. Santegoets, E. J. H. van Houtum, C. Büll and G. J. Adema, Trends Immunol., 2020, 41, 274–285 CrossRef CAS PubMed
.
- T. Tang, X. Huang, G. Zhang, Z. Hong, X. Bai and T. Liang, Signal Transduction Targeted Ther., 2021, 6, 1–13 CrossRef PubMed
.
- S. X. Lu, E. De Neef, J. D. Thomas, E. Sabio, B. Rousseau, M. Gigoux, D. A. Knorr, B. Greenbaum, Y. Elhanati, S. J. Hogg, A. Chow, A. Ghosh, A. Xie, D. Zamarin, D. Cui, C. Erickson, M. Singer, H. Cho, E. Wang, B. Lu, B. H. Durham, H. Shah, D. Chowell, A. M. Gabel, Y. Shen, J. Liu, J. Jin, M. C. Rhodes, R. E. Taylor, H. Molina, J. D. Wolchok, T. Merghoub, L. A. Diaz, O. Abdel-Wahab and R. K. Bradley, Cell, 2021, 184, 4032–4047 CrossRef CAS PubMed
.
- A. Singh and N. A. Peppas, Adv. Mater., 2014, 26, 6530–6541 CrossRef CAS PubMed
.
- J. Gačanin, C. V. Synatschke, T. Weil, J. Gačanin, C. V. Synatschke and T. Weil, Adv. Funct. Mater., 2020, 30, 1906253 CrossRef
.
- L. Shi, Y. Liu, M. Li and Z. Luo, FEBS J., 2022, 289, 3655–3665 CrossRef CAS PubMed
.
- C. Yao, C. Zhu, J. Tang, J. Ou, R. Zhang and D. Yang, J. Am. Chem. Soc., 2021, 143, 19330–19340 CrossRef CAS PubMed
.
- S. Iqbal, F. Ahmed and H. Xiong, Chem. Eng. J., 2021, 420, 130384 CrossRef CAS
.
- L. J. Deng, M. Qi, N. Li, Y. H. Lei, D. M. Zhang and J. X. Chen, J. Leukocyte Biol., 2020, 108, 493–508 CrossRef CAS PubMed
.
- X. Dong, P. Pan, D. W. Zheng, P. Bao, X. Zeng and X. Z. Zhang, Sci. Adv., 2020, 6, e1590 CrossRef PubMed
.
- P. M. Chen, W. Y. Pan, C. Y. Wu, C. Y. Yeh, C. Korupalli, P. K. Luo, C. J. Chou, W. T. Chia and H. W. Sung, Biomaterials, 2020, 230, 119629 CrossRef CAS PubMed
.
- Y. Wu, Q. Li, G. Shim and Y. K. Oh, J. Controlled Release, 2021, 330, 540–553 CrossRef CAS PubMed
.
- C. Yao, R. Zhang, J. Tang and D. Yang, Nat. Protoc., 2021, 16, 5460–5483 CrossRef CAS PubMed
.
- Q. Hu, K. Dong, J. Ming, W. Yang, H. Wang, X. Xiao and T. Huang, Mater. Today Chem., 2022, 23, 100680 CrossRef CAS
.
- J. V. de la Iglesia, R. J. C. Slebos, L. Martin-Gomez, X. Wang, J. K. Teer, A. C. Tan, T. A. Gerke, G. Aden-Buie, T. van Veen, J. Masannat, R. Chaudhary, F. Song, M. Fournier, E. M. Siegel, M. B. Schabath, J. Trad Wadsworth, J. Caudell, L. Harrison, B. M. Wenig, J. Conejo-Garcia, J. C. Hernandez-Prera and C. H. Chung, Clin. Cancer Res., 2020, 26, 1474–1485 CrossRef CAS PubMed
.
- J. Sun, M. Pan, F. Liu, W. Yu, W. Wang, F. Mo, S. Yu, Y. Zhou and X. Liu, ACS Mater. Lett., 2020, 2, 1606–1614 CrossRef CAS
.
- M. M. H. Sohel, Life Sci., 2020, 248, 117473 CrossRef CAS PubMed
.
- A. Echle, N. T. Rindtorff, T. J. Brinker, T. Luedde, A. T. Pearson and J. N. Kather, Br. J. Cancer, 2020, 124, 686–696 CrossRef PubMed
.
- A. Khanmohammadi, A. Aghaie, E. Vahedi, A. Qazvini, M. Ghanei, A. Afkhami, A. Hajian and H. Bagheri, Talanta, 2020, 206, 120251 CrossRef CAS PubMed
.
- J. L. da Silva, N. C. Cardoso Nunes, P. Izetti, G. G. de Mesquita and A. C. de Melo, Crit. Rev. Oncol. Hematol., 2020, 145, 102855 CrossRef PubMed
.
- K. Barzaman, J. Karami, Z. Zarei, A. Hosseinzadeh, M. H. Kazemi, S. Moradi-Kalbolandi, E. Safari and L. Farahmand, Int. Immunopharmacol., 2020, 84, 106535 CrossRef CAS PubMed
.
- X. Mao, S. Pan, D. Zhou, X. He and Y. Zhang, Sens. Actuators, B, 2019, 285, 385–390 CrossRef CAS
.
- S. Sun, H. Xu, Y. Yang, L. Wang, L. Ye, H. Jiang, C. Xue, Z. Shen and Z. S. Wu, Chem. Eng. J., 2022, 428, 131150 CrossRef CAS
.
- Y. Si, L. Li, N. Wang, J. Zheng, R. Yang and J. Li, ACS Appl. Mater. Interfaces, 2019, 11, 7792–7799 CrossRef CAS PubMed
.
- Y. Si, L. Xu, N. Wang, J. Zheng, R. Yang and J. Li, Anal. Chem., 2020, 92, 2649–2655 CrossRef CAS PubMed
.
- H. Wang, H. Wang, Y. Li, C. Jiang, D. Chen, Y. Wen and Z. Li, Sens. Actuators, B, 2020, 313, 128036 CrossRef CAS
.
- N. Xu, N. Ma, X. Yang, G. Ling, J. Yu and P. Zhang, Eur. Polym. J., 2020, 137, 109951 CrossRef CAS
.
- S. Mohammadi, S. Mohammadi and A. Salimi, Talanta, 2021, 224, 121895 CrossRef CAS PubMed
.
- A. Chakraborty, S. P. Ravi, Y. Shamiya, C. Cui and A. Paul, Chem. Soc. Rev., 2021, 50, 7779–7819 RSC
.
- K. Sun, P. Chen, S. Yan, W. Yuan, Y. Wang, X. Li, L. Dou, C. Zhao, J. Zhang, Q. Wang, Z. Fu, L. Wei, Z. Xin, Z. Tang, Y. Yan, Y. Peng, B. Ying, J. Chen and J. Geng, ACS Appl. Mater. Interfaces, 2021, 13, 21030–21039 CrossRef CAS PubMed
.
- W. H. Chang, Y. F. Lee, Y. W. Liu, I. Willner and W. C. Liao, Nanoscale, 2021, 13, 16799–16808 RSC
.
- X. Ji, H. Lv, X. Sun and C. Ding, Chem. Commun., 2019, 55, 15101–15104 RSC
.
- Y. Wang, Y. Fang, Y. Zhu, S. Bi, Y. Liu and H. Ju, Chem. Sci., 2022, 13, 2062–2070 RSC
.
- S. Liu, Y. Yang, M. Shi, H. Shi, D. Mao, X. Mao and Y. Zhang, ACS Sens., 2022, 7, 658–665 CrossRef CAS PubMed
.
- Q. Wang, Y. Hu, N. Jiang, J. Wang, M. Yu and X. Zhuang, Bioconjugate Chem., 2020, 31, 813–820 CrossRef CAS PubMed
.
- Y. F. Chen, M. W. Hsu, Y. C. Su, H. M. Chang, C. H. Chang and J. S. Jan, Mater. Sci. Eng., B, 2020, 114, 111025 CrossRef CAS PubMed
.
- X. Huang, N. T. Blum, J. Lin, J. Shi, C. Zhang and P. Huang, Mater. Horiz., 2021, 8, 78–101 RSC
.
- J. Zhang, Y. Guo, G. Pan, P. Wang, Y. Li, X. Zhu and C. Zhang, ACS Appl. Mater. Interfaces, 2020, 12, 21441–21449 CrossRef CAS PubMed
.
- C. Yao, H. Tang, W. Wu, J. Tang, W. Guo, D. Luo and D. Yang, J. Am. Chem. Soc., 2020, 142, 3422–3429 CrossRef CAS PubMed
.
- R. M. Borum, C. Moore, Y. Mantri, M. Xu, J. V. Jokerst, R. M. Borum, C. Moore, M. Xu, J. V. Jokerst and Y. Mantri, Adv. Sci., 2022, 2204330, DOI:10.1002/advs.202204330
.
- K. Lee, T. Kim, Y. M. Kim, K. Yang, I. Choi and Y. H. Roh, Macromol. Rapid Commun., 2021, 42, 2000457 CrossRef CAS PubMed
.
- J. Yan, H. Zou, W. Zhou, X. Yuan, Z. Li, X. Ma, C. Liu, Y. Wang, J. M. Rosenholm, W. Cui, X. Qu and H. Zhang, Biomater. Sci., 2022, 10, 4119–4125 RSC
.
- L. Yue, S. Wang, V. Wulf and I. Willner, Nat. Commun., 2019, 10, 1–10 CrossRef CAS PubMed
.
- L. Ding, J. Li, C. Wu, F. Yan, X. Li and S. Zhang, J. Mater. Chem. B, 2020, 8, 3527–3533 RSC
.
- X. Xu, Y. Jiang and C. Lu, Anal. Chem., 2022, 94, 10221–10226 CrossRef CAS PubMed
.
- K. Baek, A. D. Noblett, P. Ren and L. J. Suggs, Biomater. Sci., 2020, 8, 3130–3137 RSC
.
- X. Gao, S. Li, F. Ding, X. Liu, Y. Wu, J. Li, J. Feng, X. Zhu and C. Zhang, Adv. Mater., 2021, 33, 2006116 CrossRef CAS PubMed
.
- H. Zhang, S. Ba, J. Y. Lee, J. Xie, T. P. Loh and T. Li, Nano Lett., 2020, 20, 8399–8407 CrossRef CAS PubMed
.
- X. Fu, T. Chen, Y. Song, C. Feng, H. Chen, Q. Zhang, G. Chen and X. Zhu, Small, 2021, 17, 2101224 CrossRef CAS PubMed
.
- M. H. Ayoubi-Joshaghani, K. Seidi, M. Azizi, M. Jaymand, T. Javaheri, R. Jahanban-Esfahlan and M. R. Hamblin, Adv. Funct. Mater., 2020, 30, 2004098 CrossRef CAS
.
- J. A. Duran-Mota, J. Q. Yani, B. D. Almquist, S. Borrós and N. Oliva, ACS Biomater. Sci. Eng., 2021, 7, 4347–4361 CrossRef CAS PubMed
.
- J. Shi, Z. Shi, Y. Dong, F. Wu and D. Liu, ACS Appl. Bio Mater., 2020, 3, 2827–2837 CrossRef CAS PubMed
.
- Y. Chen and S. Shi, RSC Adv., 2022, 12, 30310–30320 RSC
.
- J. Li, C. Zheng, S. Cansiz, C. Wu, J. Xu, C. Cui, Y. Liu, W. Hou, Y. Wang, L. Zhang, I. T. Teng, H. H. Yang and W. Tan, J. Am. Chem. Soc., 2015, 137, 1412–1415 CrossRef CAS PubMed
.
- X. Wu, T. Wu, J. Liu and B. Ding, Adv. Healthcare Mater., 2020, 9, 2001046 CrossRef CAS PubMed
.
- J. Song, H. Zhang, D. Wang, J. Wang, J. Zhou, Z. Zhang, J. Wang, Y. Hu, Q. Xu, C. Xie, W. Lu and M. Liu, J. Controlled Release, 2021, 338, 583–592 CrossRef CAS PubMed
.
- Y. Lin, Y. Huang, Y. Yang, L. Jiang, C. Xing, J. Li, C. Lu and H. Yang, Anal. Chem., 2020, 92, 15179–15186 CrossRef CAS PubMed
.
- S. Walia, A. R. Chandrasekaran, B. Chakraborty and D. Bhatia, ACS Appl. Bio Mater., 2021, 4, 5392–5404 CrossRef CAS PubMed
.
- X. Wang, L. Qiu, C. Wang, Z. Gao, S. Zhou, P. Cui, P. Jiang, H. Hu, X. Ni, X. Du, J. Wang and J. Xia, Biomater. Sci., 2022, 10, 654–664 RSC
.
- L. Shen, P. Wang and Y. Ke, Adv. Healthcare Mater., 2021, 10, 2002205 CrossRef CAS PubMed
.
- Z. Xie, T. Fan, J. An, W. Choi, Y. Duo, Y. Ge, B. Zhang, G. Nie, N. Xie, T. Zheng, Y. Chen, H. Zhang and J. S. Kim, Chem. Soc. Rev., 2020, 49, 8065–8087 RSC
.
- Z. Huang, L. Qiu, T. Zhang and W. Tan, Matter, 2021, 4, 461–489 CrossRef CAS
.
- Y. Guo, Q. Zhang, Q. Zhu, J. Gao, X. Zhu, H. Yu, Y. Li and C. Zhang, Sci. Adv., 2022, 8, 2941 CrossRef PubMed
.
- F. Ding, X. Gao, X. Huang, H. Ge, M. Xie, J. Qian, J. Song, Y. Li, X. Zhu and C. Zhang, Biomaterials, 2020, 245, 119976 CrossRef CAS PubMed
.
- L. Zhou, W. Pi, M. Hao, Y. Li, H. An, Q. Li, P. Zhang and Y. Wen, Biomater. Sci., 2021, 9, 4904–4921 RSC
.
- B. Liu, J. Sun, J. Zhu, B. Li, C. Ma, X. Gu, K. Liu, H. Zhang, F. Wang, J. Su and Y. Yang, Adv. Mater., 2020, 32, 2004460 CrossRef CAS PubMed
.
- S. Jiang, Z. Ge, S. Mou, H. Yan and C. Fan, Chem, 2021, 7, 1156–1179 CAS
.
- C. Wang, X. Liu, V. Wulf, M. Vázquez-González, M. Fadeev and I. Willner, ACS Nano, 2019, 13, 3424–3433 CrossRef CAS PubMed
.
- Z. Xie, J. Shen, H. Sun, J. Li and X. Wang, Biomed. Pharmacother., 2021, 137, 111333 CrossRef CAS PubMed
.
- Q. Zhang, Y. Guo, L. Zhu, X. Liu, J. Yang, Y. Li, X. Zhu and C. Zhang, Biomater. Sci., 2021, 9, 4755–4764 RSC
.
- X. Ma, S. J. Li, Y. Liu, T. Zhang, P. Xue, Y. Kang, Z. J. Sun and Z. Xu, Chem. Soc. Rev., 2022, 51, 5136–5174 RSC
.
- Y. Chao, Q. Chen and Z. Liu, Adv. Funct. Mater., 2020, 30, 1902785 CrossRef CAS
.
- Q. Wang, Y. Qu, Z. Zhang, H. Huang, Y. Xu, F. Shen, L. Wang, Q. Wang, Y. Qu, Z. Zhang, H. Huang, Y. Xu, F. Shen, L. Wang and L. Sun, Gels, 2022, 8, 400 CrossRef CAS PubMed
.
- S. Liu, Q. Jiang, X. Zhao, R. Zhao, Y. Wang, Y. Wang, J. Liu, Y. Shang, S. Zhao, T. Wu, Y. Zhang, G. Nie and B. Ding, Nat. Mater., 2020, 20, 421–430 CrossRef PubMed
.
- J. Lee, Q. V. Le, G. Yang and Y. K. Oh, Biomaterials, 2019, 218, 119359 CrossRef CAS PubMed
.
- Q. Wang, X. Dong, H. Zhang, P. Li, X. Lu, M. Wu, W. Zhang, X. Lin, Y. Zheng, Y. Mao, J. Zhang, Y. Lin, X. Chen, D. Chen, J. Wang and J. Xiao, Chem. Eng. J., 2021, 415, 128964 CrossRef CAS
.
- F. Poustchi, H. Amani, Z. Ahmadian, S. V. Niknezhad, S. Mehrabi, H. A. Santos and M. A. Shahbazi, Adv. Healthcare Mater., 2021, 10, 2001571 CrossRef CAS PubMed
.
- J. Li, D. Yuan, X. Zheng, X. Zhang, X. Li and S. Zhang, Sci. China: Chem., 2020, 63, 546–553 CrossRef CAS
.
- X. Xu, W. Ho, X. Zhang, N. Bertrand and O. Farokhzad, Trends Mol. Med., 2015, 21, 223–232 CrossRef CAS
.
- W. Song, P. Song, Y. Sun, Z. Zhang, H. Zhou, X. Zhang and P. He, ACS Biomater. Sci. Eng., 2021, 7, 5165–5174 CrossRef CAS PubMed
.
- A. Rejhová, A. Opattová, A. Čumová, D. Slíva and P. Vodička, Eur. J. Med. Chem., 2018, 144, 582–594 CrossRef PubMed
.
- W. Tang, L. Han, S. Duan, X. Lu, Y. Wang, X. Wu, J. Liu and B. Ding, ACS Appl. Bio Mater., 2021, 4, 7701–7707 CrossRef CAS PubMed
.
- H. Wang, Y. Jin, Y. Tan, H. Zhu, W. Huo, P. Niu, Z. Li, J. Zhang, X. Jie Liang and X. Yang, Biomaterials, 2021, 275, 120992 CrossRef CAS PubMed
.
- J. Zhao, Y. Guo, Z. Tong, R. Zhang, C. Yao and D. Yang, ACS Appl. Bio Mater., 2022, 5, 3795–3805 CrossRef CAS PubMed
.
- J. Zhang, L. Yang, F. Huang, C. Zhao, J. Liu, Y. Zhang and J. Liu, Adv. Healthcare Mater., 2021, 10, 2101190 CrossRef CAS PubMed
.
- P. P. He, X. Du, Y. Cheng, Q. Gao, C. Liu, X. Wang, Y. Wei, Q. Yu and W. Guo, Small, 2022, 18, 2200263 CrossRef CAS PubMed
.
- F. Komura, K. Okuzumi, Y. Takahashi, Y. Takakura and M. Nishikawa, Molecules, 2020, 25, 728 CrossRef CAS PubMed
.
- T. Peng, Z. Deng, J. He, Y. Li, Y. Tan, Y. Peng, X. Q. Wang and W. Tan, Coord. Chem. Rev., 2020, 403, 213080 CrossRef CAS
.
- L. Cheng, X. Wang, F. Gong, T. Liu and Z. Liu, Adv. Mater., 2020, 32, 1902333 CrossRef CAS PubMed
.
- F. G. L. Medeiros Borsagli, J. S. Rodrigues, R. A. Aguiar, A. E. Paiva, J. F. B. Vasquez, W. T. do S. Ramos, P. Allibrandini, E. P. A. Rocha, M. P. Gonçalves and F. E. de Souza, Int. J. Biol. Macromol., 2022, 209, 2109–2118 CrossRef CAS PubMed
.
- K. H. Shen, C. H. Lu, C. Y. Kuo, B. Y. Li and Y. C. Yeh, J. Mater. Chem. B, 2021, 9, 7100–7116 RSC
.
- X. Meng, K. Zhang, W. Dai, Y. Cao, F. Yang, H. Dong and X. Zhang, Chem. Sci., 2018, 9, 7419–7425 RSC
.
- M. Leo, E. Lattuada, D. Caprara, L. Salvatori, A. Vecchione, F. Sciortino, P. Filetici and A. Stoppacciaro, Biomater. Sci., 2022, 10, 1304–1316 RSC
.
- N. Li, X. Y. Wang, M. H. Xiang, J. W. Liu, R. Q. Yu and J. H. Jiang, Anal. Chem., 2019, 91, 11 Search PubMed
.
|
This journal is © The Royal Society of Chemistry 2023 |