DOI:
10.1039/D2NR05610K
(Review Article)
Nanoscale, 2023,
15, 4682-4693
Silicon photonics interfaced with microelectronics for integrated photonic quantum technologies: a new era in advanced quantum computers and quantum communications?
Received
10th October 2022
, Accepted 17th December 2022
First published on 23rd December 2022
Abstract
Silicon photonics is rapidly evolving as an advanced chip framework for implementing quantum technologies. With the help of silicon photonics, general-purpose programmable networks with hundreds of discrete components have been developed. These networks can compute quantum states generated on-chip as well as more extraordinary functions like quantum transmission and random number generation. In particular, the interfacing of silicon photonics with complementary metal oxide semiconductor (CMOS) microelectronics enables us to build miniaturized quantum devices for next-generation sensing, communication, and generating randomness for assembling quantum computers. In this review, we assess the significance of silicon photonics and its interfacing with microelectronics for achieving the technology milestones in the next generation of quantum computers and quantum communication. To this end, especially, we have provided an overview of the mechanism of a homodyne detector and the latest state-of-the-art of measuring squeezed light along with its integration on a photonic chip. Finally, we present an outlook on future studies that are considered beneficial for the wide implementation of silicon photonics for distinct data-driven applications with maximum throughput.
I. Introduction
Silicon photonics (SiP) is considered instrumental in advancing cutting-edge chip platforms for quantum computing.1,2 This technology platform is being used to make general programmable networks with hundreds of separate components that can compute quantum states generated on-chip, as well as more specific applications like the creation of random numbers of quantum and quantum transmission.3,4 Quantum information can be programmed in different various states during quantum communication. However, photonic states have gained significant attention for quantum communication as their lack of interaction with the external environment, low noise, and light-speed transmission.5 Based on previous research, it has been observed that quantum communication requires only one quantum channel for information transmission.6 Quantum information processing (QIP) utilizes the photon states for information processing, computation, storage, and transmission.7 Silicon photonics assist any device in realizing the QIP task with the utilization of single-qubit operations,8,9 and fundamentally it has proven that entanglement operations need to be appropriate for implementing QIP with quantum control.10,11
Recent advancements in silicon photonics have resulted in high-performance building blocks including detectors, modulators, multiplexer (Mux)/de-multiplexer (Demux), and switches, which are ideal for optoelectronic integration with CMOS systems for optical interconnections.12,13 Furthermore, silicon photonics is recognized as a pioneering technology that has driven advanced breakthroughs in the area of data communication, telecommunication, sensing, and security.14,15 The significant element behind silicon photonics is its capability of realizing highly integrated, small, and photonic sub-systems. An important factor for the scalability of these systems can be achieved by applying CMOS-based microfabrication technologies.16,17 Moreover, silicon photonics with CMOS electronics empowers the realization of low-cost devices that can achieve high-speed sensing and also perform complex computations efficiently.18,19
Fig. 1 shows a schematic illustration of silicon photonics with CMOS for realizing low-cost devices and achieving complex computing-based operations. In the area of the data center, communication, computer, and telecom, silicon photonics assist in building devices that are capable of implementing high-speed data communication with low power consumption. It is believed that the core-to-core communication will be improved in processor technology by shifting from multicore to many-core communications.20,21 As each core operates at speeds of a gigabyte per second (GB s−1), photonic interconnection integrated into an intra-chip achieves and handles GB s−1 or terabytes per second (TBps).22,23 Because of its unique signal propagation method, the photonic interconnection will be able to reach such a maximum speed without signal interference or power consumption concerns.24,25 Concerning broader application perspectives, it is believed that the inclusion of quantum computing in the data center will empower security and networking aspects. For example, quantum networking will enable the direct ability to interact with the gigantic computational power of quantum computers and the exchange of information while remaining within the realm of the quantum world.26 The transformation of data teleportation will allow for highly scalable and energy-efficient networks while also preserving data security at every physical layer via quantum encryption. As a result, quantum computing in transportation allows us to imagine more sustainable cities where cars, buses, and pedestrians are routed in real-time in the safest, most efficient ways.27 The advantages of quantum computing are manifold. For instance, routing optimization has the following applications: it decreases the amount of time people spend in traffic, increases safety, lowers pollution, and helps city planners better visualise the impact of large-scale infrastructure projects like public transportation. Furthermore, quantum computing has a significant impact on basic sciences as well, where it can deliver a multitude of functions such as energy efficiency and carbon management in the area of renewable energy.28 In renewable energy sectors, quantum computing is useful for the optimization of materials and the infrastructure in the energy sector with simulation for complex energy system processes.
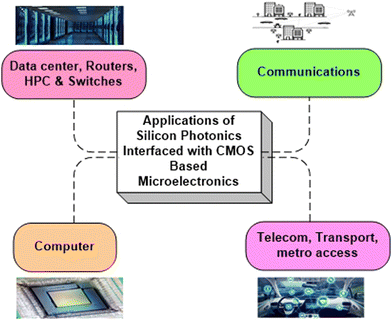 |
| Fig. 1 Applications of silicon photonics with CMOS-based microelectronics. | |
The integration of silicon photonics and electronics is categorized into two types namely: hybrid integration29 and monolithic integration.30 Photonic components are equipped on a single chip in hybrid integration.31 In monolithic integration, photonic devices and electronics are linked on a single chip in monolithic integration.32 In monolithic integration, silicon is recognized as a material that is significantly suitable for the integration of optics and photonics.33 During the integration process, for achieving the maximum level of photonic function integration, light needs to be limited in submicron waveguides with a medium (Δn ∼ 0.5) to large (Δn ∼ 2) refractive index between the clap and the cladding.34 The majority of experiments relied on the utilization of silicon on insulator (SOI) substrates because CMOS technology has been adopted by them.35
In this review, we present the mechanism of interfacing silicon photonics with microelectronics and discuss the integration of photonics with electronic parts. The different methodologies that are involved during integration are briefly addressed. In the first step, the measurement of squeezing light is addressed as it significantly assists in building modern quantum technology. Subsequently, we discuss the integration of a homodyne detector on a photonic chip. The mechanism for utilizing a homodyne detector for amalgamating a silicon microelectronics chip with a photonic chip is also presented, where a homodyne detector device is utilized for squeezing the fiber-coupled source.
The organization of the article is as follows: section II covers an overview of silicon photonics; section III covers the measuring squeezed light; section IV covers the homodyne detector; section V covers the interfacing of microelectronics with silicon photonics; section VI covers the squeezing source with fiber-coupled; and section VII covers the future perspectives along with conclusion in the final section.
II. Overview of silicon photonics
Photonic devices are extensively utilized in a variety of fields, including physics, energy, and information technology.36 Among the several materials of option, silicon has attracted the attention of both the academics and industry. In order to achieve a high integration density, cost-effectiveness, and the maximum yield, silicon photonics relies on the traditional CMOS manufacturing process.37 The three matured silicone family substances that are frequently used in photonics research and development are silicon nitride (Si3N4), silicon, and silica (SiO2).38Fig. 2 illustrates the material absorption features of the three materials. The silicon material possesses the minimum wavelength absorption ranging from 1.1–8.5 micrometer (μm) to the mid-infrared (MIR) and near-infrared (NIR) regions.39,40
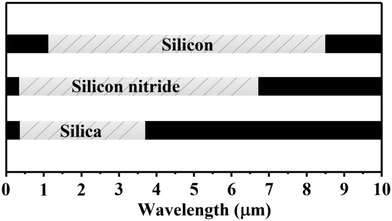 |
| Fig. 2 Material absorption characteristics reproduced with permission41 Copyright 2020, Wiley. | |
Silicon nitride is a material that can be used to implement silicon photonics at wavelengths less than 1.1 μm.41 It is concluded by researchers that silica is an appropriate element for planar lightwave circuits (PLCs) as it features a low propagation loss.42 The refractive indices and non-linear coefficients for SiO2, Si3N4, and silicon materials in the spectrum of NIR are described in Table 1. Silicon products have the maximum refractive index, resulting in a maximum index contrast for silicone waveguides and an extremely compact footprint. The high silicon thermo-optic (TO) coefficient enables silicon wavelength systems very sensitive to differences in the temperature specifically for micro-activity devices.
Table 1 Optical properties of SiO2, Si3N4, and silicon materials
Material |
Index of refraction |
Kerr non-linearity [m2 W−1] |
TO coefficient [K−1] |
Silica42 |
1.444 |
2.2 × 10−20 |
0.95 × 10−5 |
Silicon nitride43 |
2.0 |
2.45 × 10−19 |
2.45 × 10−5 |
Silicon44 |
3.467 |
4.4 × 10−18 |
1.86 × 10−4 |
Silicon photonics provides an optical path for all communication blocks and connections. It allows for the deployment of greater silicon IC equipment. The low losses (<1 dB m−1 loss achieved), as the fundamental component of the silicon photonic chip connecting all photonic components, enabling the creation of an optical chip by a mixture and match of several functional devices.45 Silicon, silicon-nitride, and silica are included in these waveguides depending on the application.46,47 Chip-based applications employ silicon photonics as a basic building component for waveguide connections using an optical fiber to create an optical input/output(I/O).48,49 The input and output connectors can be vertical or threshold, with tape to broaden the mode and properly align mono-mode fibers.
The monolithic silicon platform may integrate and interconnect photonic devices with diverse functions, such as photodetectors and modulators, depending on the waveguide system.50 Furthermore, heterogeneous integration using III–V materials gives the chip's optical lasers and amplifiers, the necessary rise channels. An essential part of silicon photonics was the optical modulator, which was crucial to the success of the promotion of the technology. A variety of systems have been established to offer rapid speed light modulation from an electrical source. The Mach–Zehnder modulator (MZM) is the most popular technique proved in mass production. This modulator is extremely responsive to the temperature and has a very broad modulation bandwidth. To considerably expand the bandwidth of optical modulators, heterogeneous integration permits the use of III–V materials and lithium niobate.
III. Measuring squeezed light
Photonic technology for quantum information processing focuses heavily on squeezed light sources.51 Squeezing is crucial for quantum computing and quantum sensing. These light sources are dependent on waveguides with periodic poles and are mounted on platforms with low-index contrast.52 The development of modern quantum technology is largely dependent on silicon photonics taking advantage of its sophisticated photonic chip platform.53 A monolithic assembly of both the quantum hardware and the corresponding microelectronics for classical control and readout is regarded to be the key to enhancing this type of photonic quantum technology.54 Researchers have demonstrated that hundreds of separate components may be used to handle quantum states synthesized on-chip using general-purpose programmable networking.55 However, device scalability along with performance is somewhat limited due to the classical interface hardware that is still employed for integrated quantum photonics with large-footprint discrete electronics.56,57 Integration of silicon quantum photonics or photonic quantum technologies with monolithic CMOS circuits is required to achieve the desired device functionality and scalability.58–60 The adoption of CMOS compatibility is thought to minimize the overall device footprint, allowing maximum performance classical control, and readout resources to scale with quantum technology.61 Therefore, current research in photonic quantum technology focuses on exploiting the unique properties of quantum physics and technology.62 The goal is to create innovative routes to take the current state-of-the-art in computing, communication, and measurement to the next level. Taking advantage of silicon photonics which uses light as a carrier of information in silicon microchips, new avenues are being explored for developing these next-generation technologies.63 To this end, squeezed light, which is a quantum effect, is considered very useful to realize these advanced technologies. It is believed that the application of squeezed light can play a pivotal role in quantum communications and quantum computers.
Current research and development efforts are aimed at improving the ways of measuring squeezed light which is considered to have a big impact on these technologies.64 Measuring squeezed light requires advanced detectors and these detectors require to be specially engineered for ultra-low electronic noise to identify the weak quantum features of light.65 Developing this capability could significantly boost the processing speed of emergent information technologies like optical computers and light-based communications.66
Furthermore, the higher bandwidth of the detector can result in much faster performance with calculations and information transmission. However, the principle challenge is the limitation of these detectors to measure the speed of signals, which is required to measure about one thousand million cycles per second to achieve the goal.67 An advanced concept in measuring squeezed light on the basis of homodyne detector and chip-scale approach. It is concluded the homodyne detector performance is improved.68,69
IV. Homodyne detector
Homodyne detectors are used to estimate quantum states and to represent quantum processes. They are essentially hardware that is intended to measure light in a sensitive manner.70 Quantum-secured communication, coherent Ising machines, quantum state and process tomography, dual-comb spectroscopy, random number generation, and quantum computing are some of the applications of homodyne detectors.71 Furthermore, research has revealed that homodyne detectors can be used to improve the performance of recently suggested optical neural networks that operate below the Landauer limit.72 However, as we described in the beginning, the speed performance of many applications is usually constrained by the utilization of electronic circuits and non-monolithic photonics.73
Recent research advances have suggested the use of integrated photodiodes that are considered promising for increasing the homodyne detector bandwidth that could potentially result in tens of gigahertz (GHz) speed performance enhancement.74 Researchers have shown such potential applications for classical silicon photonic interconnects. It has also been shown that for shot-noise-constrained applications including quantum technology, the integrated approach is usually limited to 150 megahertz (MHz) due to the implementation of transimpedance amplifiers (TIAs) with discrete electronic components.75 TIAs are necessary to magnify the feeble photocurrent subtraction of the two photodiodes with minimum noise. This configuration results in parasitic capacitance, which increases the noise of the circuit. This subsequently limits the bandwidth of the overall detector, which prevents the ∼10 s GHz raw performance of integrated photodiodes.
Researchers showed that the optical integration of a homodyne detector onto a silicon photonics chip and the resulting device was shown to be compact and operated at high speeds up to 150 MHz with low noise.76 A low-cost system for continuous-variable quantum key distribution (CV-QKD) is implemented with a fiber optical communication infrastructure on a silicon photonic chip and achieved a secret key rate of 0.14 kbps over a simulated distance of 100 km in fiber.77 On-chip quantum tomography of coherent states using the homodyne detector was used to characterize the general quantum states of light with a quantum random number output of 1.2 Gbps.69 The vacuum condition of the electromagnetic field was measured, and offline post-processing was used to achieve this. Furthermore, the generated random numbers were demonstrated to pass all of the NIST test suite's statistical tests. Fig. 3(a) shows the optical input characterization setup with a 1550 nm CW laser source. A 99/1 beam splitter transmits 99 percentage of light on the LO channel and the remaining portion into the signal channel. The PM package includes a variable optical attenuator (VOA) and an off-chip phase modulator. These are used to adjust the phase and amplitude of coherent states when the tomography of coherent states is performed.
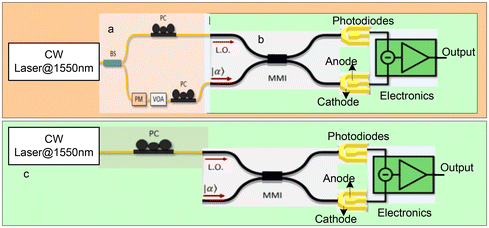 |
| Fig. 3 Schematic depiction of the homodyne detector setup.69 | |
As demonstrated in Fig. 3(b), the silicon photonics homodyne detector is shown with its beam-splitting operation. Using a multi-mode interferometer (MMI) with two on-chip Ge photodiodes, this is achieved. An off-chip TIA amplifies the currents by subtracting them from each other. Fig. 3(c) shows an approach for generating random numbers using optical vacuum states as an input. It is based on the homodyne detector's bottom port, which is closed because no optical beam is available. The LO is then infused from the top port, where the optical power of the LO at the photodiodes can be maximized using a polarization controller. Researchers used the iSiPP25G IMEC technology to construct the reported photonics device on an SOI chip as part of a multi-project wafer run organized by IMEC foundry services (Fig. 3).78 The beam was split using a multi-mode interference (MMI) device with two single-mode input waveguides and two identical single-mode output waveguides.78 This was performed by applying a 1550 nm laser source to one of the waveguides in the MMI and using a grating coupler to couple the laser to that waveguide79 and if the laser is attenuated, it serves as the source of the coherent state that is monitored using a detector. Moreover, we have illustrated the technical attributes of the homodyne detector in Table 2. A die-level balanced homodyne receiver with a 40 dB CMRR up to 1 GHz is presented for coherent optical access and continuous-variable quantum applications.80 A 3 GHz bandwidth die-level balanced homodyne detector with a significant quantum-to-classical noise removal of 19.1 dB was obtained.81 A customized integrated TIA constructed in a 100 nm GaAs pHEMT technology was utilized in this co-integrated balanced homodyne detector.82 A hybrid packaging was employed to amalgamate an InGaAs homodyne detector, a high-bandwidth TIA, and a photonic integrated circuit optimized for vacuum state quantum random number generator (QRNG) implementation.83
Table 2 Technical attributes of the homodyne detector
Attributes |
Details |
Electrical filter for channel selection |
Yes |
Local oscillator laser at a receiver |
Yes |
Polarization control |
Yes |
Narrowband filtering |
No |
Balanced optical receivers for quadrature detection |
2 |
Number of degrees of freedom |
1 (magnitude) |
Electrical bandwidth required for a balanced optical receiver |
∼Rs |
V. Interfacing of silicon microelectronics with silicon photonics
Researchers recently showed that integrating silicon nanophotonics with microelectronics improved the performance of homodyne detectors. These were used to measure non-classical light whilst miniaturizing the detector's footprint to sub-mm. This was achieved in CMOS-compatible technology for the scale-up of the process.84 Nanophotonics on s germanium and silicon was interconnected using integrated silicon and germanium amplifiers (Fig. 4).85 A homodyne detector's overall capacitance was reduced, resulting in faster quantum light measurement.85 The detector was implemented with a 3 dB bandwidth of 1.7 GHz and a shot noise limit of 9 GHz, as well as a 0.84 mm2 minimized necessary footprint. Using a CW laser, researchers squeezed light sources from 100 MHz to 9 GHz and measured the spectrum using the detector; moreover, it is also used to perform state tomography.86 Fast homodyne detectors utilized for quantum optics of continuous-variable were developed as a result of this work. These results pave the way for the development of photonic quantum devices with complete stack integration.
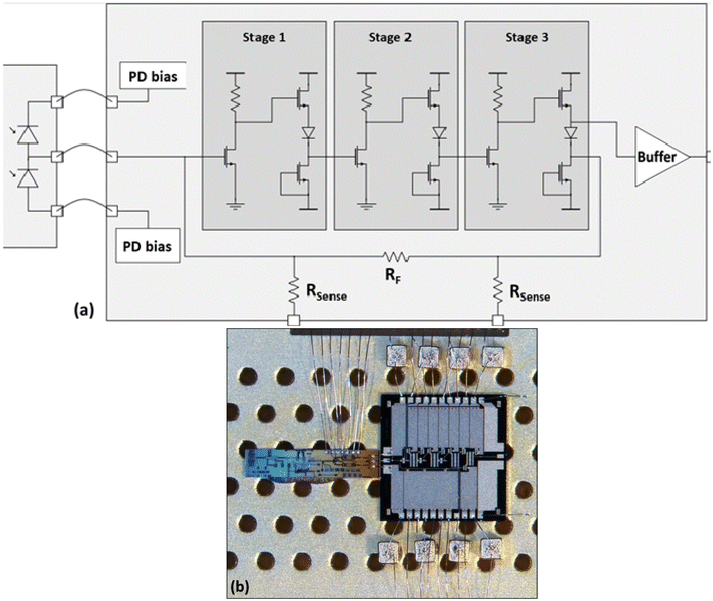 |
| Fig. 4 (a) TIA schematic. (b) Micrograph of the manufactured devices; on the left side is the photonic IC and the on the right side is the TIA.85 | |
The Integration of two silicon chips resulted in the creation of the photonic quantum device. At record-breaking speeds, this setup was utilized to calculate the peculiar features of squeezed quantum light. It was shown that coupling a silicon-photonic homodyne detector with a microelectronics amplifier can improve its performance. The 1.7 GHz bandwidth achieved was an order of magnitude higher than the earlier fastest incorporated silicon–germanium experiment. In all conventional homodyne detection, the measured shot noise above 9 GHz revealed the possibility for an order of magnitude larger bandwidth than the present one.87 The schematic of the amplifier is shown in Fig. 4(a), with each stage consisting of a common source amplifier guided by a source follower with a current source and a level shifting Schottky diode. The voltages are sensed by two large resistors, so they do not affect the circuit's high-frequency behavior. This voltage difference is used to tune the MZM on the photonic integrated circuit (PIC) to achieve appropriate balancing. The manufactured devices are shown in Fig. 4(b), with the PIC on the left and the TIA on the right.
VI. Squeezing source with fiber-coupled waveguide chip
Measurement of squeezed light and states formed from squeezed light using non-Gaussian techniques is required in quantum optics applications.9 Researchers evaluated broadband squeezed light created from a fiber-coupled periodically poled lithium niobate (LN) ridge waveguide chip using a homodyne detection device (Fig. 5).88 CW pump laser is concentrated at 1560.61 nm. It is initially boosted with an erbium-doped fibre amplifier (EDFA) before being separated into squeezing and local oscillator paths. SHG pump power can be adjusted independently of LO with 90% attenuation.89 As a result, residual 780 nm light cannot reach the homodyne detector.90 ESA noise powers were then recorded above 1 s with an 8 MHz resolution bandwidth in zero-span mode. A frequency doubling stage, a parametric downconverter, an amplified telecom pump laser, and a parametric downconverter were used to create the source.91 As a result, the chip's second input was filled with compressed air.
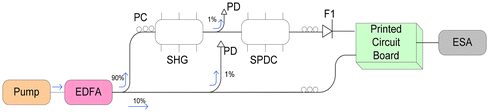 |
| Fig. 5 An integrated detector for squeezing the fiber-coupled source. | |
As a result of the lowering of shot-noise clearing, there is an effective loss in the squeezing at higher frequencies. There was an average of 0.87 0.01 dB of squeezing at 9.2 GHz over a 3 dB bandwidth.74 Anti-squeezing levels and measured squeezing with SHG power are shown that are monitored by employing a 99
:
1 beam splitter in the middle of PPLN modules.92 The squeezed vacuum's reconstructed Wigner function is shown, which is integrated from 0 to 2 GHz. The phase of the local oscillator was examined at 5 Hz utilizing the on-chip phase shifter. By performing homodyne tomography on-chip of the detected squeezed vacuum, the device's ability to rebuild input quantum states was demonstrated with phase dependence.93
VII. Future perspective
In this section, we present the implementation of silicon photonics in the future. Here we discuss the significance of heterogenous integration and monolithic integration. Moreover, the implementation of silicon photonics for high-speed transmission in a 5G network with minimum power consumption is discussed.
(a) Heterogeneous integration
The silicon photonics manufacturing mechanism is crucial for the future progress and integration of the device.94 Low-loss waveguides are important to unite the different components of optical in the PIC. The PIC capacity to scale for many components is considerably enhanced by having access to both SiO2-based waveguides and Si3N4-based waveguides.95 Many applications require a Si3N4-based waveguide for coarse wavelength division multiplexer (CWDM) and de- multiplexer, where it enables for temperature-insensitive components.96 In many different designs, Ge photodiodes are highly dependable. The potential yield and dependability required for scaling are driven by the ongoing procedure control of these important components. Juniper and Intel have shown diverse integrations of III–V materials such as InP.97 PICs with integrated lasers are evaluated as a possibility for switch capacity to fall below 200 Tbps in the next decade. Temperature-insensitive lasers with Si3N4 cavities and Si3N4 AWGs significantly encourage to the implementation of dense wavelength division multiplexing (DWDM) systems when compared to the CWDM systems that are currently employed in data centers.98,99 Hybrid and heterogeneous integration has made significant progress.100 In these areas, the capabilities of silicon and Si3N4 in terms of processing (better lithography and higher temperatures) are being utilized to accomplish magnitude loss minimization (low to 0.1 dB m−1).101
(b) Monolithic integration
Monolithic integration of optical lasers and gain has been a goal in the silicon photonics industry for the past ten years.102 The achievement of this goal is a constraint with distinct problems, namely: (a) variation in the coefficients of thermal expansion increases to strain cracking and fault migration throughout cooldown; (b) a Si substrate was used to synthesize a polar compound with anti-phase domains.103 The first constraints can be mainly overcome by better templates and greater containment in the separate heterostructure of the containment (SCH), which in the past lowered the overall epitaxial thickness from 8 μm to merely 4 μm. The eradication of the antiphase domain is implemented in GaAs, GaP, and AIAs layers.104
(c) Adoption of silicon photonics for data drive applications
Silicon photonics, both as data transmission and sensing technologies, will play a key role in the data-intensive computation facilitated by distributed edge computing and 5G networks.105,106 The silicon photonics connectivity will gain case applications in the backhaul radio transportation network and radio baseband during the radio access network transitions for maximum throughput.107 Silicon photonic elements are also becoming a significant computing platform, especially, as Moore's law suffers from the utilization of artificial intelligence (AI) and machine learning (ML) applications with workloads and power consumption.108,109
Another growing topic in silicon photonics is programmable photonic circuits, which employ interoperability in significant and sophisticated Si PICs for diverse optical activities and improve the device setup and control.110,111 Ultimately, silicon photonics is one of a handful of potential approaches to fulfill quantum computing. Fig. 6 briefly demonstrates the approach of integrating silicon photonics with microelectronics for developing future-generation computers based on quantum technology for achieving high performance and communication. In the future, the wide deployment of silicon photonics assists to achieve high-throughput data-driven applications.
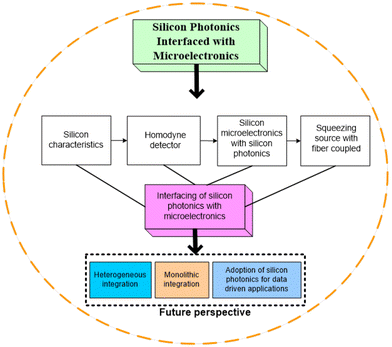 |
| Fig. 6 Graphical view of interfacing silicon photonics with microelectronics. | |
VIII. Conclusion
Silicon photonics interfaced with CMOS microelectronics empowers the implementation of faster data transfer over long distances with low power and high throughput. At present, electrical I/O is used for interconnection between the server board and processors. Every bit of speed-up comes at the cost of significantly increasing power usage. Recent research advances in combining a silicon photonic chip with a silicon microelectronics chip have shown huge promise in taking quantum photonic technology to the next level. Effective measurements of quantum light and the development of advanced detectors and hardware seem to be possible. Many new applications that include advanced quantum computing and quantum communications are anticipated as a result of such advances.
Conflicts of interest
The authors declare that there is no conflict of interest regarding the publication of this paper.
References
- W. Bogaerts and L. Chrostowski, Silicon photonics circuit design: methods, tools and challenges, Laser Photonics Rev., 2018, 12, 1700237, DOI:10.1002/lpor.201700237.
- T. D. Ladd, F. Jelezko, R. Laflamme, Y. Nakamura, C. Monroe and J. L. O'Brien, Quantum computers, Nature, 2010, 464, 45–53, DOI:10.1038/nature08812.
- P. Sibson, C. Erven, M. Godfrey, S. Miki, T. Yamashita, M. Fujiwara, M. Sasaki, H. Terai, M. G. Tanner and C. M. Natarajan, Chip-based quantum key distribution, Nat. Commun., 2017, 8, 1–6, DOI:10.1038/ncomms13984.
-
L. Chrostowski and M. Hochberg, Silicon photonics design: from devices to systems, Cambridge University Press, 2015. DOI:10.1017/CBO9781316084168.
- F. Flamini and N. Spagnolo, Photonic quantum information processing : a review, 2019, 82, 016001, DOI:10.1088/1361-6633/aad5b2.
-
G. L. Long, Quantum Secure Direct Communication: Principles, Current Status, Perspectives, IEEE Veh. Technol. Conf., 2017, DOI:10.1109/VTCSpring.2017.8108697.
- S. Slussarenko and G. J. Pryde, Photonic quantum information processing: A concise review, Appl. Phys. Rev., 2019, 6, 41303, DOI:10.1063/1.5115814.
-
R. R. Tucci, An introduction to Cartan's KAK decomposition for QC programmers, arXiv, 2005, Preprint, Quant-Ph/0507171. DOI:10.48550/arXiv.quant-ph/0507171.
- N. Khaneja and S. J. Glaser, Cartan decomposition of SU (2n) and control of spin systems, Chem. Phys., 2001, 267, 11–23, DOI:10.1016/S0301-0104(01)00318-4.
- P. J. Shadbolt, M. R. Verde, A. Peruzzo, A. Politi, A. Laing, M. Lobino, J. C. F. Matthews, M. G. Thompson and J. L. O. Brien, Generating, manipulating and measuring entanglement and mixture with a reconfigurable photonic circuit, Nat. Photonics, 2011, 6, 45–49, DOI:10.1038/nphoton.2011.283.
- J. Wang, S. Paesani, Y. Ding, R. Santagati, P. Skrzypczyk, A. Salavrakos, J. Tura, R. Augusiak, L. Mančinska, D. Bacco, D. Bonneau, J. W. Silverstone, Q. Gong, A. Acín, K. Rottwitt, L. K. Oxenløwe, J. L. O'Brien, A. Laing and M. G. Thompson, Multidimensional quantum entanglement with large-scale integrated optics, Science, 2018, 360, 285–291, DOI:10.1126/science.aar7053.
- H. Subbaraman, X. Xu, A. Hosseini, X. Zhang, Y. Zhang, D. Kwong and R. T. Chen, Recent advances in silicon-based passive and active optical interconnects, Opt. Express, 2015, 23, 2487, DOI:10.1364/oe.23.002487.
- S. Feng, T. Lei, H. Chen, H. Cai, X. Luo and A. W. Poon, Silicon photonics: from a microresonator perspective, Laser Photonics Rev., 2012, 6, 145–177, DOI:10.1002/lpor.201100020.
- B. Jalali and S. Fathpour, Silicon photonics, J. Lightwave Technol., 2006, 24, 4600–4615, DOI:10.1109/JLT.2006.885782.
- Y. Arakawa, T. Nakamura, Y. Urino and T. Fujita, Silicon photonics for next generation system integration platform, IEEE Commun. Mag., 2013, 51, 72–77, DOI:10.1109/MCOM.2013.6476868.
-
T. Pinguet, S. Gloeckner, G. Masini and A. Mekis, CMOS photonics: a platform for optoelectronics integration, in Silicon Photonics II, 2011, pp. 187–216. DOI:10.1007/978-3-642-10506-7_8.
-
L. Tsybeskov, D. J. Lockwood and M. Ichikawa, Silicon photonics: CMOS going optical [scanning the issue], Proc. IEEE, 2009, 97, 1161–1165, DOI:10.1109/JPROC.2009.2021052.
-
X. Chen, M. M. Milosevic, S. Stanković, S. Reynolds, T. D. Bucio, K. Li, D. J. Thomson, F. Gardes and G. T. Reed, The emergence of silicon photonics as a flexible technology platform, Proc. IEEE, 2018, 106, 2101–2116, DOI:10.1109/JPROC.2018.2854372.
- P. Dong, Y. K. Chen, G. H. Duan and D. T. Neilson, Silicon photonic devices and integrated circuits, Nanophotonics, 2014, 3, 215–228, DOI:10.1515/nanoph-2013-0023.
- J. Wang, F. Sciarrino, A. Laing and M. G. Thompson, Integrated photonic quantum technologies, Nat. Photonics, 2020, 14, 273–284, DOI:10.1038/s41566-019-0532-1.
-
Q. Feng, D. Ban, H. Li and W. Dou, Optical burst switching for many-core processor-to-memory photonic networks, in: 2011 6th Int. Conf. Comput. Sci. Educ., IEEE, 2011, pp. 541–546. DOI:10.1109/ICCSE.2011.6028697.
- C. García-Meca, S. Lechago, A. Brimont, A. Griol, S. Mas, L. Sánchez, L. Bellieres, N. S. Losilla and J. Martí, On-chip wireless silicon photonics: From reconfigurable interconnects to lab-on-chip devices, Light: Sci. Appl., 2017, 6, 1–11, DOI:10.1038/lsa.2017.53.
- S. J. B. Yoo, Future prospects of silicon photonics in next generation communication and computing systems, Electron. Lett., 2009, 45, 584–588, DOI:10.1049/el.2009.1304.
-
L. Wosinski, Z. Wang, F. Lou, D. Dai, S. Lourdudoss and L. Thylen, Advanced silicon device technologies for optical interconnects, in Optoelectron. Integr. Circuits XIV, International Society for Optics and Photonics, 2012, p. 826506. DOI:10.1117/12.909919.
- J. Chan, G. Hendry, A. Biberman and K. Bergman, Architectural exploration of chip-scale photonic interconnection network designs using physical-layer analysis, J. Lightwave Technol., 2010, 28, 1305–1315 Search PubMed.
-
K. Qazi and I. Aizenberg, Towards quantum computing algorithms for datacenter workload predictions, in: 2018 IEEE 11th Int. Conf. Cloud Comput., IEEE, 2018, pp. 900–903. DOI:10.1109/CLOUD.2018.00131.
- C. H. V. Cooper, Exploring Potential Applications of Quantum Computing in Transportation Modelling, IEEE Trans. Intell. Transp. Syst., 2022, 23, 14712–14720, DOI:10.1109/TITS.2021.3132161.
- H. P. Paudel, M. Syamlal, S. E. Crawford, Y.-L. Lee, R. A. Shugayev, P. Lu, P. R. Ohodnicki, D. Mollot and Y. Duan, Quantum Computing and Simulations for Energy Applications: Review and Perspective, ACS Eng. Au, 2022, 2, 151–196, DOI:10.1021/acsengineeringau.1c00033.
- S. Chakravarty, M. Teng, R. Safian and L. Zhuang, Hybrid material integration in silicon photonic integrated circuits, J. Semicond., 2021, 42, 041303, DOI:10.1088/1674-4926/42/4/041303.
-
M. Paniccia, A. Liu, N. Izhaky and A. Barkai, Integration challenge of silicon photonics with microelectronics, in IEEE Int. Conf. Gr. IV Photonics, 2005. 2nd, 2005, pp. 20–22. DOI:10.1109/GROUP4.2005.1516389.
- K. Sharma and V. K. Sehgal, Modern architecture for photonic networks-on-chip, J. Supercomput., 2020, 76, 9901–9921, DOI:10.1007/s11227-020-03220-2.
-
S. Li, N. G. Tarr and N. Y. Winnie, Monolithic integration of opto-electronics by silicon photonics foundry service, in Smart Photonic Optoelectron. Integr. Circuits XXI, International Society for Optics and Photonics, 2019, p. 1092217. DOI:10.1117/12.2510477.
- B. G. Lee, A. V. Rylyakov, W. M. J. Green, S. Assefa, C. W. Baks, R. Rimolo-Donadio, D. M. Kuchta, M. H. Khater, T. Barwicz and C. Reinholm, Monolithic silicon integration of scaled photonic switch fabrics, CMOS logic, and device driver circuits, J. Lightwave Technol., 2013, 32, 743–751, DOI:10.1109/JLT.2013.2280400.
- R. Marchetti, C. Lacava, L. Carroll, K. Gradkowski and P. Minzioni, Coupling strategies for silicon photonics integrated chips [Invited], Photonics Res., 2019, 7, 201, DOI:10.1364/prj.7.000201.
-
W. Bogaerts and S. K. Selvaraja, in 13 - Silicon-on-insulator (SOI) technology for photonic integrated circuits (PICs), ed. O. Kononchuk and B.-Y. B. T.-S.-O.-I. (SOI) T. Nguyen, Woodhead Publishing, 2014, pp. 395–434. DOI:10.1533/9780857099259.2.395.
-
G. Moody, V. J. Sorger, P. W. Juodawlkis, W. Loh, C. Sorace-Agaskar, M. Davanco, L. Chang, J. E. Bowers, N. Quack, C. Galland, I. Aharonovich, M. A. Wolff, C. Schuck, N. Sinclair, M. Lončar, T. Komljenovic, D. Weld, S. Mookherjea, S. Buckley, M. Radulaski, S. Reitzenstein, B. Pingault, B. Machielse, D. Mukhopadhyay, A. Akimov, A. Zheltikov, G. S. Agarwal, K. Srinivasan, J. Lu, H. X. Tang, W. Jiang, T. P. McKenna, A. H. Safavi-Naeini, S. Steinhauer, A. W. Elshaari, V. Zwiller, P. S. Davids, N. Martinez, M. Gehl, J. Chiaverini, K. K. Mehta, J. Romero, N. B. Lingaraju, A. M. Weiner, D. Peace, R. Cernansky, M. Lobino, E. Diamanti, L. T. Vidarte and R. M. Camacho, Roadmap on Integrated Quantum Photonics, 2021. https://arxiv.org/abs/2102.03323.
- H. H. Radamson, H. Zhu, Z. Wu, X. He, H. Lin, J. Liu, J. Xiang, Z. Kong, W. Xiong and J. Li, State of the art and future perspectives in advanced CMOS technology, Nanomaterials, 2020, 10, 1555, DOI:10.3390/nano10081555.
- H. Nejadriahi, S. Pappert, Y. Fainman and P. Yu, Efficient and compact thermo-optic phase shifter in silicon-rich silicon nitride, Opt. Lett., 2021, 46, 4646–4649, DOI:10.1364/OL.431757.
- H. Lin, Z. Luo, T. Gu, L. C. Kimerling, K. Wada, A. Agarwal and J. Hu, Mid-infrared integrated photonics on silicon: a perspective, Nanophotonics, 2018, 7, 393–420, DOI:10.1515/nanoph-2017-0085.
- K. Zhang, L. Zhang, L. Han, L. Wang, Z. Chen and H. Xing, Recent progress and challenges based on two- dimensional material photodetectors Recent progress and challenges based on two-dimensional material photodetectors, 2020, 5, 1901153, DOI:10.1088/2632-959X/abd45b.
- Y. Su, Y. Zhang, C. Qiu, X. Guo and L. Sun, Silicon photonic platform for passive waveguide devices: Materials, fabrication, and applications, Adv. Mater. Technol., 2020, 5, 1901153, DOI:10.1002/admt.201901153.
- T. Miya, Silica-based planar lightwave circuits: passive and thermally active devices, IEEE J. Sel. Top. Quantum Electron., 2000, 6, 38–45, DOI:10.1109/2944.736076.
- A. Arbabi and L. L. Goddard, Measurements of the refractive indices and thermo-optic coefficients of Si 3 N 4 and SiO x using microring resonances, Opt. Lett., 2013, 38, 3878–3881, DOI:10.1364/OL.38.003878.
- P. E. Barclay, K. Srinivasan and O. Painter, Nonlinear response of silicon photonic crystal microresonators excited via an integrated waveguide and fiber taper, Opt. Express, 2005, 13, 801–820, DOI:10.1364/OPEX.13.000801.
- M. J. R. Heck, J. F. Bauters, M. L. Davenport, D. T. Spencer and J. E. Bowers, Ultra–low loss waveguide platform and its integration with silicon photonics, Laser Photonics Rev., 2014, 8, 667–686, DOI:10.1002/lpor.201300183.
- S. Bogdanov, M. Y. Shalaginov, A. Boltasseva and V. M. Shalaev, Material platforms for integrated quantum photonics, Opt. Mater. Express, 2017, 7, 111–132, DOI:10.1364/OME.7.000111.
-
A. Rahim, T. Spuesens, R. Baets and W. Bogaerts, Open-access silicon photonics: Current status and emerging initiatives, Proc. IEEE, 2018, 106, 2313–2330, DOI:10.1109/JPROC.2018.2878686.
- A. L. Gaeta, M. Lipson and T. J. Kippenberg, Photonic-chip-based frequency combs, Nat. Photonics, 2019, 13, 158–169, DOI:10.1038/s41566-019-0358-x.
-
Y. Ding, D. Bacco, D. Llewellyn, I. Faruque, S. Paesani, M. Galili, A. Laing, K. Rottwitt, M. Thompson and J. Wang, Silicon Photonics for Quantum Communication, in: 2019 21st Int. Conf. Transparent Opt. Networks, IEEE, 2019, pp. 1–4. DOI:10.1109/ICTON.2019.8840038.
- N. Margalit, C. Xiang, S. M. Bowers, A. Bjorlin, R. Blum and J. E. Bowers, Perspective on the future of silicon photonics and electronics, Appl. Phys. Lett., 2021, 118, 220501, DOI:10.1063/5.0050117.
- G. Moody, L. Chang, T. J. Steiner and J. E. Bowers, Chip-scale nonlinear photonics for quantum light generation, AVS Quantum Sci., 2020, 2, 41702, DOI:10.1116/5.0020684.
- Y. Zhang, M. Menotti, K. Tan, V. D. Vaidya, D. H. Mahler, L. G. Helt, L. Zatti, M. Liscidini, B. Morrison and Z. Vernon, Squeezed light from a nanophotonic molecule, Nat. Commun., 2021, 12, 1–6, DOI:10.1038/s41467-021-22540-2.
- A. W. Elshaari, W. Pernice, K. Srinivasan, O. Benson and V. Zwiller, Hybrid integrated quantum photonic circuits, Nat. Photonics, 2020, 14, 285–298, DOI:10.1038/s41566-020-0609-x.
- K. Xu, Silicon electro-optic micro-modulator fabricated in standard CMOS technology as components for all silicon monolithic integrated optoelectronic systems, J. Micromech. Microeng., 2021, 31, 54001, DOI:10.1088/1361-6439/abf333.
- B. Chen, H. Wu, C. Xin, D. Dai and L. Tong, Flexible integration of free-standing nanowires into silicon photonics, Nat. Commun., 2017, 8, 1–7, DOI:10.1038/s41467-017-00038-0.
- S. Paesani, Y. Ding, R. Santagati, L. Chakhmakhchyan, C. Vigliar, K. Rottwitt, L. K. Oxenløwe, J. Wang, M. G. Thompson and A. Laing, Generation and sampling of quantum states of light in a silicon chip, Nat. Phys., 2019, 15, 925–929, DOI:10.1038/s41567-019-0567-8.
- E. Suhir, Fiber optics engineering: physical design for reliability, Facta Universitatis. Series: Electronics and Energetic, 2014, 27, 153–182, DOI:10.2298/FUEE1402153S.
- E. Suhir, Analytical thermal stress modeling in electronics and photonics engineering: Application of the concept of interfacial compliance, J. Therm. Stress, 2019, 42, 29–48, DOI:10.1080/01495739.2018.1525331.
- E. Suhir and R. Ghaffarian, Electron Device Subjected to Temperature Cycling: Predicted Time-to-Failure, J. Electron. Mater., 2019, 48, 778–779, DOI:10.1007/s11664-018-06867-z.
- C. Wang, M. Zhang, X. Chen, M. Bertrand, A. Shams-Ansari, S. Chandrasekhar, P. Winzer and M. Lončar, Integrated lithium niobate electro-optic modulators operating at CMOS-compatible voltages, Nature, 2018, 562, 101–104, DOI:10.1038/s41586-018-0551-y.
- B. Szelag, K. Hassan, L. Adelmini, E. Ghegin, P. Rodriguez, F. Nemouchi, P. Brianceau, E. Vermande, A. Schembri and D. Carrara, Hybrid III–V/silicon technology for laser integration on a 200-mm fully CMOS-compatible silicon photonics platform, IEEE J. Sel. Top. Quantum Electron., 2019, 25, 1–10, DOI:10.1109/JSTQE.2019.2904445.
- G. Kurizki, P. Bertet, Y. Kubo, K. Mølmer, D. Petrosyan, P. Rabl and J. Schmiedmayer, Quantum technologies with hybrid systems, Proc. Natl. Acad. Sci. U. S. A., 2015, 112, 3866–3873, DOI:10.1073/pnas.1419326112.
- Z. Wang, A. Abbasi, U. Dave, A. De Groote, S. Kumari, B. Kunert, C. Merckling, M. Pantouvaki, Y. Shi and B. Tian, Novel light source integration approaches for silicon photonics, Laser Photonics Rev., 2017, 11, 1700063, DOI:10.1002/lpor.201700063.
- A. Acín, I. Bloch, H. Buhrman, T. Calarco, C. Eichler, J. Eisert, D. Esteve, N. Gisin, S. J. Glaser and F. Jelezko, The quantum technologies roadmap: a European community view, New J. Phys., 2018, 20, 80201, DOI:10.1088/1367-2630/aad1ea.
- Y. Gong, R. Kumar, A. Wonfor, S. Ren, R. V. Penty and I. H. White, Secure optical communication using a quantum alarm, Light: Sci. Appl., 2020, 9, 1–10, DOI:10.1038/s41377-020-00409-1.
- D. Awschalom, K. K. Berggren, H. Bernien, S. Bhave, L. D. Carr, P. Davids, S. E. Economou, D. Englund, A. Faraon and M. Fejer, Development of quantum interconnects (quics) for next-generation information technologies, PRX Quantum, 2021, 2, 17002, DOI:10.1103/PRXQuantum.2.017002.
- A. H. Atabaki, S. Moazeni, F. Pavanello, H. Gevorgyan, J. Notaros, L. Alloatti, M. T. Wade, C. Sun, S. A. Kruger and H. Meng, Integrating photonics with silicon nanoelectronics for the next generation of systems on a chip, Nature, 2018, 556, 349–354, DOI:10.1038/s41586-018-0028-z.
- G. Huang, W. Deng, H. Tan and G. Cheng, Generation of squeezed states and single-phonon states via homodyne detection and photon subtraction on the filtered output of an optomechanical cavity, Phys. Rev. A, 2019, 99, 43819, DOI:10.1103/PhysRevA.99.043819.
- F. Raffaelli, G. Ferranti, D. H. Mahler, P. Sibson, J. E. Kennard, A. Santamato, G. Sinclair, D. Bonneau, M. G. Thompson and J. C. F. Matthews, A homodyne detector integrated onto a photonic chip for measuring quantum states and generating random numbers, Quantum Sci. Technol., 2018, 3, 25003, DOI:10.1088/2058-9565/aaa38f.
- A. Zavatta, S. Viciani and M. Bellini, Tomographic reconstruction of the single-photon Fock state by high-frequency homodyne detection, Phys. Rev. A, 2004, 70, 53821, DOI:10.1103/PhysRevA.70.053821.
- L. Caspani, C. Xiong, B. J. Eggleton, D. Bajoni, M. Liscidini, M. Galli, R. Morandotti and D. J. Moss, Integrated sources of photon quantum states based on nonlinear optics, Light: Sci. Appl., 2017, 6, 1–7, DOI:10.1038/lsa.2017.100.
- R. Hamerly, L. Bernstein, A. Sludds, M. Soljačić and D. Englund, Large-scale optical neural networks based on photoelectric multiplication, Phys. Rev. X, 2019, 9, 21032, DOI:10.1103/PhysRevX.9.021032.
- V. Stojanović, R. J. Ram, M. Popović, S. Lin, S. Moazeni, M. Wade, C. Sun, L. Alloatti, A. Atabaki and F. Pavanello, Monolithic silicon-photonic platforms in state-of-the-art CMOS SOI processes, Opt. Express, 2018, 26, 13106–13121, DOI:10.1364/OE.26.013106.
- F. Lenzini, J. Janousek, O. Thearle, M. Villa, B. Haylock, S. Kasture, L. Cui, H.-P. Phan, D. V. Dao and H. Yonezawa, Integrated photonic platform for quantum information with continuous variables, Sci. Adv., 2018, 4, eaat9331, DOI:10.1126/sciadv.aat9331.
-
K.-J. Boller, A. van Rees, Y. Fan, J. Mak, R. E. M. Lammerink, C. A. A. Franken, P. J. M. van der Slot, D. A. I. Marpaung, C. Fallnich and J. P. Epping, Hybrid integrated semiconductor lasers with silicon nitride feedback circuits, in Photonics, Multidisciplinary Digital Publishing Institute, 2020, p. 4. DOI:10.3390/photonics7010004.
- K. Wei, W. Li, H. Tan, Y. Li, H. Min, W.-J. Zhang, H. Li, L. You, Z. Wang and X. Jiang, High-speed measurement-device-independent quantum key distribution with integrated silicon photonics, Phys. Rev. X, 2020, 10, 31030, DOI:10.1103/PhysRevX.10.031030.
- G. Zhang, J. Y. Haw, H. Cai, F. Xu, S. M. Assad, J. F. Fitzsimons, X. Zhou, Y. Zhang, S. Yu and J. Wu, An integrated silicon photonic chip platform for continuous-variable quantum key distribution, Nat. Photonics, 2019, 13, 839–842, DOI:10.1038/s41566-019-0504-5.
-
R. Wang, Silicon Photonics: Overview, Building Block Modeling, Chip Design and Its Driving Integrated Circuits Co-design, 2020. https://www.lib.uidaho.edu/digital/etd/items/wang_idaho_0089e_11466.html.
- Y. Xu and J. Xiao, Ultracompact and high efficient silicon-based polarization splitter-rotator using a partially-etched subwavelength grating coupler, Sci. Rep., 2016, 6, 1–11, DOI:10.1109/ECOC52684.2021.9606023.
-
D. Milovančev, F. Honz, N. Vokić, F. Laudenbach, H. Hübel and B. Schrenk, Ultra-Low Noise Balanced Receiver with> 20 dB Quantum-to-Classical Noise Clearance at 1 GHz, in 2021 Eur. Conf. Opt. Commun., IEEE, 2021, pp. 1–4. DOI:10.1038/srep27949.
-
F. Honz, D. Milovančev, N. Vokić, C. Pacher and B. Schrenk, Broadband Balanced Homodyne Detector for High-Rate (> 10 Gb/s) Vacuum-Noise Quantum Random Number Generation, in 2021 Eur. Conf. Opt. Commun., IEEE, 2021, pp. 1–4. DOI:10.1109/ECOC52684.2021.9605899.
- C. Bruynsteen, M. Vanhoecke, J. Bauwelinck and X. Yin, Integrated balanced homodyne photonic–electronic detector for beyond 20 GHz shot-noise-limited measurements, Optica, 2021, 8, 1146–1152, DOI:10.1364/OPTICA.420973.
- B. Bai, J. Huang, G.-R. Qiao, Y.-Q. Nie, W. Tang, T. Chu, J. Zhang and J.-W. Pan, 18.8 Gbps real-time quantum random number generator with a photonic integrated chip, Appl. Phys. Lett., 2021, 118, 264001, DOI:10.1063/5.0056027.
- T. Barwicz and Y. Taira, Low-cost interfacing of fibers to nanophotonic waveguides: design for fabrication and assembly tolerances, IEEE Photonics J., 2014, 6, 1–18, DOI:10.1109/JPHOT.2014.2331251.
-
J. F. Tasker, J. Frazer, G. Ferranti, E. J. Allen, L. F. Brunel, S. Tanzilli, V. D'Auria and J. C. F. Matthews, 9∼ GHz measurement of squeezed light by interfacing silicon photonics and integrated electronics, arXiv, 2020, Preprint, arXiv:2009.14318. DOI:10.48550/arXiv.2009.14318.
-
F. G. S. L. Brandão, R. Kueng and D. S. França, Fast and robust quantum state tomography from few basis measurements, arXiv, 2020, Preprint, arXiv:2009.08216, DOI:10.48550/arXiv.2009.08216.
- X. Zhang, Y. Zhang, Z. Li, S. Yu and H. Guo, 1.2-GHz balanced homodyne detector for continuous-variable quantum information technology, IEEE Photonics J., 2018, 10, 1–10, DOI:10.1109/JPHOT.2018.2866514.
- O. Alibart, V. D'Auria, M. De Micheli, F. Doutre, F. Kaiser, L. Labonté, T. Lunghi, É. Picholle and S. Tanzilli, Quantum photonics at telecom wavelengths based on lithium niobate waveguides, J. Opt., 2016, 18, 104001, DOI:10.48550/arXiv.1608.01100.
- S. Arahira and H. Murai, Wavelength conversion of incoherent light by sum-frequency generation, Opt. Express, 2014, 22, 12944, DOI:10.1364/oe.22.012944.
-
S. Paesani and A. Laing, Quantum Processors in Silicon Photonics, in Silicon Photonics IV, Springer, 2021, pp. 449–489. DOI:10.1088/2040-8978/18/10/104001 DOI:10.1007/978-3-030-68222-4_11.
- F. Mondain, T. Lunghi, A. Zavatta, E. Gouzien, F. Doutre, M. De Micheli, S. Tanzilli and V. D'Auria, Chip-based squeezing at a telecom wavelength, Photonics Res., 2019, 7, A36–A39, DOI:10.1364/PRJ.7.000A36.
- M. Mehmet, S. Steinlechner, T. Eberle, H. Vahlbruch, A. Thüring, K. Danzmann and R. Schnabel, Observation of cw squeezed light at 1550 nm, Opt. Lett., 2009, 34, 1060, DOI:10.1364/ol.34.001060.
- J. F. Tasker, J. Frazer, G. Ferranti, E. J. Allen, L. F. Brunel, S. Tanzilli, V. D'Auria and J. C. F. Matthews, Silicon photonics interfaced with integrated electronics for 9 GHz measurement of squeezed light, Nat. Photonics, 2021, 15, 11–15, DOI:10.1038/s41566-020-00715-5.
- N. Quack, H. Sattari, A. Y. Takabayashi, Y. Zhang, P. Verheyen, W. Bogaerts, P. Edinger, C. Errando-Herranz and K. B. Gylfason, MEMS-enabled silicon photonic integrated devices and circuits, IEEE J. Quantum Electron., 2019, 56, 1–10, DOI:10.1109/JQE.2019.2946841.
- T. Sharma, J. Wang, B. K. Kaushik, Z. Cheng, R. Kumar, Z. Wei and X. Li, Review of recent progress on silicon nitride-based photonic integrated circuits, IEEE Access, 2020, 8, 195436–195446, DOI:10.1109/ACCESS.2020.3032186.
- S. S. Cheung and M. R. T. Tan, Silicon Nitride (Si3N4) (De-)Multiplexers for 1-μm CWDM Optical Interconnects, J. Lightwave Technol., 2020, 38, 3404–3413, DOI:10.1109/JLT.2019.2956382.
- D. Caimi, P. Tiwari, M. Sousa, K. E. Moselund and C. B. Zota, Heterogeneous Integration of III-V Materials by Direct Wafer Bonding for High-Performance Electronics and Optoelectronics, IEEE Trans. Electron Devices, 2021, 68, 3149–3156, DOI:10.1109/TED.2021.3067273.
-
T. Komljenovic, D. Huang, P. Pintus, M. A. Tran, M. L. Davenport and J. E. Bowers, Photonic integrated circuits using heterogeneous integration on silicon, Proc. IEEE, 2018, 106, 2246–2257, DOI:10.1109/JPROC.2018.2864668.
- C. Xiang, W. Jin, J. Guo, J. D. Peters, M. J. Kennedy, J. Selvidge, P. A. Morton and J. E. Bowers, Narrow-linewidth III-V/Si/Si 3 N 4 laser using multilayer heterogeneous integration, Optica, 2020, 7, 20, DOI:10.1364/optica.384026.
- P. Kaur, A. Boes, G. Ren, T. G. Nguyen, G. Roelkens and A. Mitchell, Hybrid and heterogeneous photonic integration, APL Photonics, 2021, 6, 61102, DOI:10.1063/5.0052700.
- S. Cuyvers, A. Hermans, M. Kiewiet, J. Goyvaerts, G. Roelkens, K. Van Gasse, D. Van Thourhout and B. Kuyken, Heterogeneous integration of Si photodiodes on silicon nitride for near-visible light detection, Opt. Lett., 2022, 47, 937–940, DOI:10.1364/OL.447636.
- A. Giuglea, G. Belfiore, M. M. Khafaji, R. Henker and F. Ellinger, A 37-Gb/s Monolithically Integrated Electro-Optical Transmitter in a Silicon Photonics 250-nm BiCMOS Process, J. Lightwave Technol., 2022, 40, 2080–2086, DOI:10.1364/JLT.40.002080.
- K. Nikolaidou, H. M. Oliveira, S. Cardoso, P. P. Freitas, V. Chu and J. P. Conde, Monolithic Integration of Multi-Spectral Optical Interference Filter Array on Thin Film Amorphous Silicon Photodiodes, IEEE Sens. J., 2022, 22, 5636–5643, DOI:10.1109/JSEN.2022.3150228.
- Y. Park, Y.-G. Roh and H. Jeon, GaAs/AIAs-oxide omnidirectional reflector, Proc. SPIE, 2003, 5000, 266–270, DOI:10.1117/12.479485.
- A. Kumar, M. Gupta and R. Singh, Topological integrated circuits for 5G and 6G, Nat. Electron., 2022, 1–2, DOI:10.1038/s41928-022-00775-1.
- R. Sabella, Silicon Photonics for 5G and Future Networks, IEEE J. Sel. Top. Quantum Electron., 2020, 26, 1–11, DOI:10.1109/JSTQE.2019.2948501.
- J. C. Adcock, J. Bao, Y. Chi, X. Chen, D. Bacco, Q. Gong, L. K. Oxenløwe, J. Wang and Y. Ding, Advances in silicon quantum photonics, IEEE J. Sel. Top. Quantum Electron., 2020, 27, 1–24, DOI:10.1109/JSTQE.2020.3025737.
-
S.-H. Chang, Patent Portfolio Analysis of the Synergy between Machine Learning and Photonics, in Photonics, Multidisciplinary Digital Publishing Institute, 2022, p. 33. DOI:10.3390/photonics9010033.
- B. J. Shastri, A. N. Tait, T. Ferreira de Lima, W. H. P. Pernice, H. Bhaskaran, C. D. Wright and P. R. Prucnal, Photonics for artificial intelligence and neuromorphic computing, Nat. Photonics, 2021, 15, 102–114, DOI:10.1038/s41566-020-00754-y.
- W. Bogaerts, D. Pérez, J. Capmany, D. A. B. Miller, J. Poon, D. Englund, F. Morichetti and A. Melloni, Programmable photonic circuits, Nature, 2020, 586, 207–216, DOI:10.1038/S41586-020-2764-0.
- F. Kaiser, B. Fedrici, A. Zavatta, V. D'Auria and S. Tanzilli, A fully guided-wave squeezing experiment for fiber quantum networks, Optica, 2016, 3, 362–365, DOI:10.1364/OPTICA.3.000362.
|
This journal is © The Royal Society of Chemistry 2023 |