DOI:
10.1039/D1TA08252C
(Perspective)
J. Mater. Chem. A, 2022,
10, 5878-5888
Single-atom-based catalysts for photoelectrocatalysis: challenges and opportunities
Received
25th September 2021
, Accepted 30th November 2021
First published on 30th November 2021
Abstract
Photoelectrocatalysis (PEC) has recently emerged as a promising strategy for utilizing solar energy due to its unique features in combining the merits of electrocatalysis and photocatalysis in solar energy harvesting, charge kinetics and catalytic reactions. However, it still encounters bottlenecks of scarce reaction sites and low product selectivity, restricting its development toward practical applications. Over the past decade, single-atom-based catalysts (SACs) with atomically dispersed metal sites have demonstrated immense potential in many catalytic reactions. In comparison with their nanoparticles (NPs) or bulk counterparts, the SACs normally have enormous surface active sites and trigger unique surface reactions, which, if rationally designed, can open up wide possibilities for PEC. Here, we overview the challenges and opportunities of SACs in PEC. We first summarize the advantages of SACs in enhancing the adsorption of reactants, charge transfer, catalytic selectivity, and catalytic activity. Then we discuss the rational design of single-atom active sites in the photoelectrochemical system. In the end, challenges and perspectives regarding the fundamental research and development of single-atom catalysts in PEC are also proposed. We foresee that this timely perspective can provide some important insights for researchers in this field and accelerate the development of PEC.
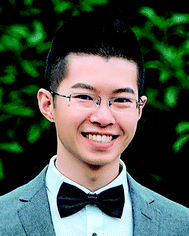 Dong Liu | Dong Liu obtained his B.S. in chemical physics in 2012 and PhD in inorganic chemistry under the tutelage of Professor Yujie Xiong in 2017, both from the University of Science and Technology of China (USTC). From 2018 to 2021, he worked as a Postdoctoral Research Fellow at Nanyang Technological University, Singapore. He is currently a Research Professor at the USTC. His research interests focus on developing a photo(electro)chemical system for solar-driven production of fuels and valuable chemicals. |
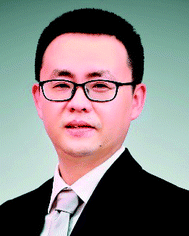 Yujie Xiong | Yujie Xiong received his B.S. in chemical physics in 2000 and PhD in inorganic chemistry in 2004, both from the University of Science and Technology of China (USTC). From 2004 to 2009, he worked as a Postdoctoral Fellow at the University of Washington in Seattle and as a Research Associate at the University of Illinois at Urbana-Champaign, respectively. He was the Principal Scientist of the National Nanotechnology Infrastructure Network (NSF-NNIN) site at Washington University in St. Louis in 2009–2011. He joined the USTC faculty in 2011, and currently is the Chair Professor of Chemistry. His research centers on solar-driven artificial carbon cycle. |
Introduction
The solar-to-chemical energy conversion has been regarded as one major solution to addressing current energy and environmental issues.1–3 Stimulated by solar light, photogenerated electrons and holes can participate in redox reactions to realize the energy conversion.4,5 Solar-driven redox reactions are generally achieved via three pathways, i.e., photocatalysis, photoelectrocatalysis, and photothermal catalysis. Photoelectrocatalysis (PEC) is a promising technology for solar-to-chemical energy conversion, which incorporates the merits of electrocatalysis and photocatalysis in solar energy harvesting, charge kinetics and catalytic reactions.5–7 External bias or built-in photovoltage in the PEC system can promote the separation of photogenerated charges, invigorate the charges, increase their densities, and prolong their lifetimes. Abundant energetic photogenerated charges can activate reactants and motivate the chemical transformation. Nevertheless, PEC still encounters bottlenecks of scarce active sites and low catalytic selectivity, limiting its development.8–11
Single-atom-based catalysts (SACs) have recently emerged as a new class of catalysts with excellent activity and selectivity for various catalytic reactions,12–14 which offer new opportunities for overcoming the limitations of the PEC system. The catalytic properties of SACs highly depend on the electronic structures of metal single atoms (SAs), the metal–support interaction, and the coordination environment.15 These factors are critical to the adsorption, activation and catalytic reactions of reactants and intermediates in heterocatalysis. Maximal atom utilization efficiency and tuable density of single-atom sites ensure a sufficient quantity of active catalytic centers with cost effectiveness.16 Furthermore, modulated electronic structures by tuning metal–support interaction bring vitality for the activation of adsorbed molecules and the catalytic reactions.17 In the meantime, the specific adsorption configuration of small molecules on single-atom sites can regulate the selectivity of catalytic reactions.18,19 Beyond enhancing the catalytic properties, establishing single-atom sites can also accelerate the charge separation and transfer in electrocatalysis and photocatalysis.
The photoelectrodes work as light-harvesting centers in the photoelectrochemical system and simultaneously catalyze chemical reactions with/without external bias.4 The above-mentioned bottlenecks of PEC restrict the development toward practical applications. Correspondingly, SACs may provide a valuable solution to overcoming these bottlenecks of PEC on active sites and catalytic selectivity.12,20 The introduction of SACs in PEC demands the rational design of photoelectrodes, and in turn, their composition and feasibleness need to be fully considered.21,22 By projecting the practicable scheme of SAC-coupled PEC, the high density of active sites and modulated electronic structures of SACs together with adequate photogenerated charges in PEC can notably boost the efficiency of solar-to-chemical energy conversion. As such, the activity and selectivity of photoelectrochemical reactions can be pertinently improved by the establishment of SACs in PEC.
To date, the application of SACs in PEC has been explored with only a few attempts.23–25 Effectual integration of SACs in PEC still needs adequate research and precise regulation toward practical applications. This perspective summarizes the advantageous features of SACs in enhancing charge transfer, catalytic selectivity, and catalytic activity, which may compensate the shortcomings of general PEC reactions. Then recent progress regarding single-atom-based photoelectrochemical applications will be summarized, and, on this basis, we propose potential design principles and strategies for future development of SACs in PEC. In the end, we discuss the challenges and perspectives regarding fundamental research and advances to provide insights and guidelines for this emerging field.
Advantages of SACs for heterocatalysis
Heterocatalysis involves three essential steps: the adsorption of reactants from a fluid phase, the surface catalytic reaction of adsorbed species, and the desorption of products into the fluid phase. The total efficiency of heterocatalysis is determined by the efficiency of each step.26 PEC is one type of heterocatalysis which contains similar steps for catalytic reactions as mentioned above. Specifically, directional adsorption of reactants, efficient charge separation and transfer, adequate activation of adsorbed species, selective catalytic transformation, and effective mass transfer are critical to the entire catalytic reaction. For these key steps in heterocatalysis, the unique geometric and electronic properties of SACs can play to their advantages.27 SACs provide a powerful solution to achieve desirable chemical reactions with high activity and selectivity. This section will discuss the merits of SACs demonstrated in heterocatalysis, which can potentially take effect in photoelectrocatalysis.
Modulated electronic structures
Electronic structures of catalysts are crucial to the overall catalytic process.28–30 The adsorption bond strength of reactants or intermediates, the charge kinetics and the catalyst activity have been proven to be highly related to the characteristics of the surface electronic structure of catalysts.31,32 After the metal SAs are anchored or coordinated with the support, the strong metal–support interaction can regulate the electronic structures of SAs due to the redistribution of charge density.33–35 In the meantime, metal species and their oxidation states, coordination micro-environment and strain engineering can also affect the electronic structures of SACs, thereby altering the catalytic performance.34,35 For instance, Lu et al. revealed the support effect on the electronic structures of Pt SA-based catalysts both experimentally and theoretically, and found that Pt atoms in Pt1/Co3O4 had the largest 5d state depletion leading to extreme catalytic activity and excellent stability for hydrolytic dehydrogenation.36 For solar-to-chemical energy conversion, Zhou et al. found that the deployment of Au SAs in Cd1−xS modulated the electronic structures of Au atoms, which could promote the charge transfer and offer sufficient electrons for photocatalytic CO2 reduction reaction.30 In another case, band engineering of PtII–C3N4 photocatalysts by introducing Pt SAs was proven to be an efficient solution to modify the band energy levels and shift the valence band maximum level to promote water oxidation.37 Similarly, rational design of Ta3N5 photoanodes by gradient Mg doping could improve the PEC water oxidation performance due to band engineering.38 Overall, coupling the modulated electronic structures of SACs with the band engineering of photoelectrodes will facilitate the PEC reactions by improving different steps of photoelectrocatalysis.
Improved adsorption of reactants
Adsorption of reactants from a fluid phase is the first essential step in heterocatalysis. Generally, there are two forms of adsorption: chemisorption and physisorption, via chemical bonding or van der Waals forces between molecules and the catalyst surface, respectively. The strength and the orientation of chemisorption are critical for the subsequent activation and dissociation of adsorbed intermediates.39 The geometry, composition, atomic arrangements and electronic structures of the catalysts, as well as the configuration of molecules, directly administrate the chemisorption process of reactants on the surface of the catalysts.40 The incorporation of single metal atoms in SACs affects the symmetry of the d-band states and the filling due to the metal–support interaction. The unique electronic and structural properties in SACs can facilitate the strengthened and directional chemisorption of the reactants.41 From the perspective of the electronic structure, SACs composed of different metals could lead to varied catalytic performance.32 Parkinson et al. revealed the adsorption of the probe CO molecule on different model single-atom catalysts Me1/Fe3O4 (001) (Me: Cu, Ag, Au, Ni, Pd, Pt, Rh, or Ir) through experimental characterization studies and density functional theory (DFT) calculations.42 Coordinating the metal atoms with a support alters their electronic structure. The charge transfer from different single metal sites to the support affects the d-states of the metal, the strength of the metal–CO bond and the adsorption energy of CO on single metal sites. In addition to the electronic structure, the unique geometric structure of SACs can also direct the adsorption of reactants on the atomically dispersed active sites. Ohno and Liu et al. inferred that the spatial and electronic features of SACs directly influenced the adsorption configuration of O2 molecules on atomically dispersed Sb, as shown in Fig. 1a and b, thereby affecting the reaction pathway and the selectivity of photocatalytic O2 reduction reaction.19 In addition, first-principles simulation indicated that CO2 was adsorbed on isolated Cu sites with the adsorption energy of −0.463 eV (Fig. 1c), while the adsorption of CO2 on the neighboring Cu pairs was noticeably weaker (−0.308 eV) (Fig. 1d).18 The rational design of SACs can offer favorable configurations and advantageous adsorption energy of chemisorbed molecules on single-atom active sites, to further improve the catalytic activity and selectivity of PEC.43
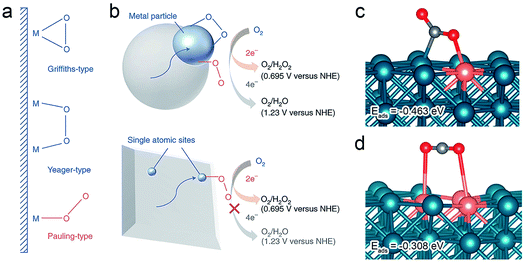 |
| Fig. 1 (a) Schematic structures of O2 adsorption on the metal surface. (b) Oxygen reduction reactions on a metal particle (top) and an isolated atomic site (bottom).19 Reproduced with permission from ref. 19. Copyright 2021, Springer Nature. (c and d) First-principles simulation for the most favorable configurations and the corresponding adsorption energies of CO2 at an isolated Cu atom (c) and two neighboring Cu atoms (d).18 Reproduced with permission from ref. 18. Copyright 2017, American Chemical Society. | |
Promoted charge separation and transfer
Anchored or coordinated with the support, single metal atoms can form strong interactions with their support.44 The metal–support interaction arises with the orbital rehybridization and charge transfer across the interface. This strong metal–support interaction in SACs is beneficial to charge separation and transfer, which can redistribute the charge density around the metal sites with the formation of new chemical bonds.45 Redistribution of d-band electrons and charge transfer between the SAs and the supports can stabilize the single active sites and boost the catalytic performances.46 Sun and Botton et al. proved that charge transfer from the Pt SAs to the N atoms could generate unoccupied 5d orbital density of states of the Pt atoms, which was the reason for the excellent hydrogen evolution performance of Pt SAs/N-doped graphene nanosheets.47 Adsorption and dissociation of molecules also benefit from the charge transfer from the single metal sites. The low O–O dissociation energy barrier and enhanced oxygen reduction reaction efficiency were attributed to the distinct charge transfer from the Nb SAs to the two O atoms, characterized by spin-polarized first-principles calculations, as illustrated in Fig. 2a.48
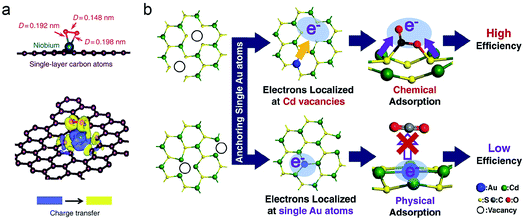 |
| Fig. 2 (a) Adsorption of O2 on single Nb sites and the corresponding electron density distribution with the charge flow from the Nb atom (blue) to the O atoms (yellow).48 Reproduced with permission from ref. 48. Copyright 2013, Nature Publishing Group. (b) Scheme of single Au atoms in CdS with Cd or S vacancies with an alternative adsorption configuration.30 Reproduced with permission from ref. 30. Copyright 2021, Springer Nature. | |
Promoted charge separation and transfer in SACs can provide sufficient charges to actuate the chemical reactions. Compared to Au nanoclusters distributed on the Cd1−xS support, the hybridization between Au 5d and S 2p orbitals in Au–S bonds is substantially stronger for Au SAs/Cd1−xS, which accelerates charge transfer on the surface and provides more sufficient electrons available for photocatalytic CO2 reduction reaction, as shown in Fig. 2b.30 In addition, the isolated single-atom active sites can trap photogenerated charges via chemical bonding in SACs, which reduces undesired charge recombination at the interface toward efficient photocatalysis.49 Steady-state photoluminescence (PL) spectroscopy and surface photovoltage (SPV) spectroscopy can examine the charge separation, recombination and transfer process.50,51 After introducing Co SAs onto partially oxidized graphene nanosheets or Bi3O4Br atomic layers, quenched PL intensity and enhanced SPV intensity were obtained, respectively, indicating increased charge separation and transfer efficiency regardless of different chemical bonds between single metal atoms and the support (Fig. 3).50,51 As a technology combining the features of electrocatalysis and photocatalysis in solar energy harvesting, the efficiency of PEC highly depends on the separation of photogenerated charges and the charge transfer between the support and catalytically active sites.6 SACs possessing the above advantages can exhibit competence in charge separation and transfer for efficient photoelectrocatalysis.
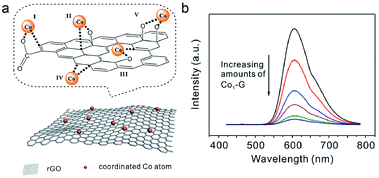 |
| Fig. 3 (a) Scheme of single Co atoms coordinated on partially oxidized graphene nanosheets (Co1–G). (b) Steady-state PL spectra of an aqueous solution containing [Ru(bpy)3]Cl2 with increasing Co1–G nanosheets.50 Reproduced with permission from ref. 50. Copyright 2018, John Wiley and Sons. | |
Activation of reactants and selective catalytic reactions
Effective catalytic activation is the step following adsorption of reactants and charge transfer, which requires establishing abundant active sites on the surface of catalysts. Isolated metal atoms in SACs are regarded as the primary active sites, which can enhance satisfactory adsorption and catalytic sites if rationally designed.17 The density of isolated metal atoms is a key indicator to the catalytic reactions. SACs with tunable metal loading have been studied to achieve optimal catalytic performance and stability. An atomically dispersed Ir catalyst with 41.6 wt% metal loading was obtained by Wang et al. based on crosslinking and self-assembly of graphene quantum dots (QDs).52 Numerous anchoring sites guarantee the sufficiency of active sites, and, at the same time, spacing between the dispersed metal atoms can prevent their aggregation. Specific surface area is a routine index to characterize a catalyst. For SACs, the surface area may not be that important in comparison with the performance. For example, introducing Sb SAs in polymer carbon nitride nanosheets reduced the surface area by 1/7.78 times, but the activity per area was greatly enhanced by more than 1900 times.19 In addition to the number of active sites, the catalytic efficacy per active site also directs the overall catalytic performance of SACs. Turnover frequency (TOF) is a major parameter for evaluating the intrinsic activity per active site.50,53 Owing to the unique coordination and electronic properties, SACs exhibit tens or hundreds of times higher TOF than the counterpart catalysts without single metal atoms. The TOF of Pd1–Ti0.87O2 with isolated Pd SAs is 11
110 h−1 for Suzuki coupling reactions (Fig. 4a), more than 200 times that of PdCl2 and Pd(OAc)2 catalysts.54 Hence it can be seen that SACs are competent for various catalytic reactions with tunable active sites and enhanced catalytic activity per site.
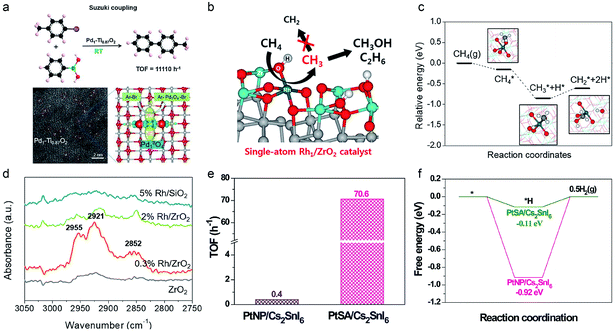 |
| Fig. 4 (a) Scheme of the single-atom Pd site on the Ti0.87O2 nanosheet for room-temperature Suzuki coupling reactions.54 Reproduced with permission from ref. 54. Copyright 2021, Chinese Chemical Society. (b) Scheme of the single-atom Rh site on the ZrO2 catalyst for selective methane conversion. (c) Energy diagram of methane activation of the intermediates. (d) Diffuse reflectance infrared Fourier transform spectroscopy spectra (DRIFT) of CH4 adsorbed on bare ZrO2, 0.3 wt% Rh/ZrO2 (Rh1/ZrO2), 2 wt% Rh/ZrO2, and 5 wt% Rh/SiO2.55 Reproduced with permission from ref. 55. Copyright 2017, American Chemical Society. (e) TOF and (f) calculated energy profile of PtSA/Cs2SnI6 and PtNP/Cs2SnI6 catalysts for photocatalytic H2 evolution, respectively.56 Reproduced with permission from ref. 56. Copyright 2021, Springer Nature. | |
Catalysts generally perform by offering an alternative pathway involving an advantageous transition state and lower activation energy.57 However, many catalytic reactions, such as CO2 reduction, O2 reduction, methane oxidation and biomass conversion, encounter problems of poor selectivity with complex products or over-reactions.55,58–60 The regulation and optimization of selective catalytic reactions are significant to obtain the desired product in the industrial process. Improved adsorption and promoted charge transfer in SACs can facilitate the selective catalytic transformation by stabilizing the intermediates.17,61 For example, stabilization of CH3 intermediates is crucial for direct methane conversion into value-added products. Cleavage of the first C–H bond of methane is the rate-determining step with the highest activation energy. Fig. 4b–d show the experimental results and DFT calculation of the selective methane conversion on Rh1/ZrO2, which confirmed that Rh SAs dispersed on the ZrO2 surface can effectively stabilize the CH3 intermediates and activate methane selectively compared to Rh NPs/ZrO2 catalysts.55 In addition to stabilizing the intermediates, SACs can also lower the activation energy barrier and alter the selectivity of some representative catalytic reactions.57 Anchoring Pt–I3 single-atom sites on all-inorganic Cs2SnI6 perovskite can greatly reduce the energy barrier of hydrogen production to 0.11 eV, compared to that of Pt NPs on Cs2SnI6 (0.92 eV), and the TOF was enhanced by 176.5-fold, as shown in Fig. 4e and f.56 For solar-driven activation of CO2, Co SAs/Bi3O4Br could energetically stabilize the COOH* intermediates to increase the selectivity to CO, probed by in situ Fourier transform infrared (FTIR) spectroscopy.51 DFT calculation indicated that the introduction of Co SAs could lower the CO2 activation energy barrier, and tune the rate-limiting step to CO* desorption instead of COOH* formation. As a result, the establishment of plentiful single-atom sites can particularly improve different steps in catalysis and boost the overall activity and selectivity, benefiting from the unique properties of SACs.62,63
Progress of SACs for photoelectrocatalysis
Photoelectrocatalysis involves semiconductor-based photocatalysis with an applied bias or internal photovoltage. PEC combines the merits of electrocatalysis and photocatalysis in solar energy harvesting, charge kinetics and catalytic reactions into one integrated system.5,64 Driven by solar light and external bias or internal photovoltage, the separation and transfer efficiency of photogenerated charges can be dramatically enhanced in PEC. Abundant photogenerated electrons and holes can activate chemical reactions, such as water splitting, CO2 reduction and biomass conversion, to realize artificial photosynthesis of high value-added chemicals.59,64,65 However, in comparison with electrocatalysis and photocatalysis, scarce reaction sites and poor product selectivity restrict the development of PEC. Among many potential solutions, the deployment of SACs could bring new possibilities to PEC. Over the past decade, SACs have demonstrated immense potential in many catalytic reactions both experimentally and theoretically.66–68 SACs exhibit unique advantages for promoting adsorption, activation and selective transformation of reactants, which may suit the remedy to the corresponding case in PEC. Inspired by the rational design and excellent performance of SACs in electrocatalysis and photocatalysis, the first single-atom-based PEC reaction was revealed in 2017.23 Nevertheless, the application of SACs in the PEC system is still rare. The progress of SACs in PEC will be discussed in further detail below (Table 1).
Table 1 A summary of SACs for PEC applications
SACs |
Substrate |
Roles of SACs in PEC |
PEC application |
Improved performance |
Reference |
Ir-anion |
NiOx@α-Fe2O3 |
Oxidation cocatalyst |
Water oxidation |
∼2-fold |
23
|
Co SAs |
g-C3N4/PCS |
Photoanode |
Water oxidation |
∼5-fold |
25
|
Ni-NC |
α-Fe2O3 |
Photoanode |
Water oxidation |
2.2-fold |
69
|
Pt SAs |
CdS |
Photoanode |
Enzyme-linked immunoassay |
|
70
|
Pt1/N–Ni |
Cu2O |
Photocathode |
Water reduction |
4.7-fold |
24
|
Ru SAs |
Cu2O |
Cathode |
N2 reduction |
∼12-fold |
71
|
CoPor–N3 |
Carbon cloth |
Photocathode |
CO2 reduction |
|
72
|
Co SAs |
Carbon |
Cathode |
N2 reduction |
|
73
|
Cui et al. first reported atomically dispersed Ni–Ir sites for PEC oxygen evolution in 2017. Soluble monomeric hexahydroxyiridate [Ir(OH)6]2− anions could electrostatically adsorb to the positively charged NiOx sites anchored on the hematite film photoanode (Fig. 5a and b).23 In such a PEC system, the photocurrent density was increased by about 2-fold at 1.23 V vs. RHE, and the onset potential was reduced by about 200 mV after the creation of Ni–Ir sites in the hematite photoanode. Atomically dispersed Ni sites acted as a movable bridge for continuous hole transfer from the hematite support to the Ir center. As a result, enhanced solar-driven oxygen evolution performance with a faradaic yield of 94% was achieved after rationally integrating the soluble molecular catalysts with atomically dispersed NiOx in a PEC system. Similarly, modifying single Ni sites/carbon nanosheets on the α-Fe2O3 photoanode could facilitate charge separation and transfer at the interface and provide sufficient active sites for oxygen evolution (Fig. 5c).69 In parallel to the metal oxide semiconductor support, single metal atoms can also bridge porous carbon spheres (PCS) with graphitic carbon nitride (g-C3N4), as illustrated in Fig. 5d.25 The strong coupling effect of PCS/Co SAs/g-C3N4, together with promoted charge separation/transfer and improved surface reaction dynamics, resulted in enhanced PEC water splitting activity.
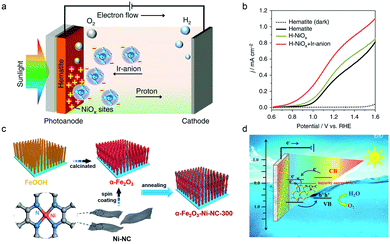 |
| Fig. 5 (a) Schematic illustration of the preparation process of the Ni–Ir site-based PEC system. Reproduced with permission from ref. 23. Copyright 2017, Springer Nature. (b) Linear sweep voltammograms of different photoanodes under AM 1.5 irradiation.23 (c) Scheme of fabrication procedures for α-Fe2O3 and α-Fe2O3–Ni-NC-300 films.69 Reproduced with permission from ref. 69. Copyright 2017, John Wiley and Sons. (d) The performance enhancement mechanism of PCS/Co SAs/g-C3N4.25 Reproduced with permission from ref. 25. Copyright 2019, Elsevier. | |
SACs can also be utilized for PEC reduction reactions.71–73 As shown in Fig. 6a and b, the Cu2O nanowire (NW) photocathode has been retrofitted with Pt1/N–Ni (Pt SAs supported by a porous nickel framework on nickel foam) as a co-catalyst for hydrogen production.24 In comparison with pristine Cu2O NW and Pt NP decorated N–Ni/Cu2O NW photocathodes, the photocurrent density of the Pt1/N–Ni/Cu2O NW photocathode was increased by about 4.7 and 2 times for PEC water reduction, respectively. The Pt1/N–Ni/Cu2O NW photocathode could produce a high photocurrent density of 11.9 mA cm−2 at 0 V vs. RHE and a high photo-to-hydrogen conversion efficiency of 1.75% due to the low charge transfer resistance at the interface and excellent electrochemical hydrogen evolution activity of the Pt1/N–Ni co-catalyst. It is worth noting that SACs can also work in a photoelectrochemical biosensing platform to perform an enzyme-free immunosorbent assay.70 Anchoring Pt SAs on CdS nanorods could remarkably enhance the photoelectrochemical biosensing signal. The strong metal–support interaction between Pt SAs and the CdS nanorod support alters the charge distribution and increases the charge density of CdS, thereby promoting the charge separation and transfer. As a result, the Pt SA–CdS nanorod photoelectrode showed excellent photoelectrochemical detection sensitivity and selectivity.
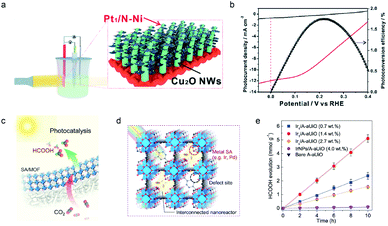 |
| Fig. 6 (a) Schematic illustration and (b) PEC performance of Pt1/N–Ni/Cu2O NWs for water reduction.24 Reproduced with permission from ref. 24. Copyright 2021, The Royal Society of Chemistry. The scheme of the (c) MOF membrane and (d) MOF structures with single metal atoms for photocatalytic CO2 reduction. (e) Time course of HCOOH evolution on A-aUiO, Ir1/A-aUiO, Irx/A-aUiO and IrNPs/A-aUiO catalysts.74 Reproduced with permission from ref. 74. Copyright 2021, Springer Nature. | |
To better understand the mechanisms, photoelectrochemical measurements have been investigated to assess the separation and transfer of photogenerated charges of photocatalysts. Introduction of SACs to photocatalysts can effectively suppress the recombination of photogenerated charges, thereby increasing the photocurrent density. For example, Wang et al. demonstrated that Ir SAs/NH2–UiO-66 (A-aUiO) metal–organic framework (MOF) membranes could effectively and selectively actuate CO2 photoreduction to HCOOH (Fig. 6c–e).74 An enhanced photocurrent density was obtained by the Ir species/A-aUiO depositing photoelectrode compared to pristine A-aUiO. When the size of Ir species was shrunk from nanoparticles to clusters (Irx) and single atoms (Ir1), the formic acid evolution rate increased from 0.01 mmol gcat−1 h−1 to 0.16 mmol gcat−1 h−1 and 0.51 mmol gcat−1 h−1, and the selectivity of HCOOH was improved from 16.5% to 79.8% and nearly 100%, respectively. Similarly, Ag, Au, Pt, Co and Er SAs supported by g-C3N4, MOFs/covalent organic frameworks (COFs), TiO2, CdS and other semiconductor nanomaterials could boost the photocurrent density and the total photocatalytic performance compared to their counterparts without single metal active sites.30,49,75–83 From these few cases, SACs have demonstrated their potentials in PEC applications. The rational deployment and fundamental investigation of SACs in PEC deserve further study.
Challenges and opportunities of SACs for photoelectrocatalysis
As mentioned in the preceding sections, SACs exhibit unique properties in heterocatalysis.20 Outstanding catalytic performance with maximal atom utilization efficiency and cost effectiveness make SACs promising in practical industrial processes. To realize solar-to-chemical transformation, PEC is a technology for competently utilizing solar energy and upgrading chemicals.9 Proven by experimental results and theoretical simulations, the implementation of SACs may remedy the deficiencies of PEC in scarce reaction sites and poor selectivity. Some studies have achieved the application of SACs in PEC according to recent reports. However, the integration of SACs in PEC still encounters practical challenges. For this reason, this perspective will propose some potential solutions and outlook for the development of SACs in PEC in this section.
Design of SACs in photoelectrodes
SACs with atomically dispersed metal sites need to be braced and stabilized by the support. The electronic and catalytic properties of SACs are determined by the coordination environment of the support and the metal atoms. Rational design and precise modulation of SACs are critical to PEC reactions, since the morphology and composition will directly influence the surface and electronic properties of SACs, thus affecting the adsorption and activation of reactants as well as the overall activity and selectivity of catalytic reactions. Fig. 7 shows two main strategies for designing SAC based photoelectrodes in PEC. The first approach is the deployment of SACs as a photoelectrode (Fig. 7a). The main module of the PEC system is the photoelectrode, which is usually a semiconductor grown on a conductive substrate, such as TiO2 nanowires and BiVO4 nanoarrays on fluorine-doped tin oxide (FTO) glasses.59,84 The selection of the support of SACs is one of the key factors for the operative integration of SACs in PEC (Fig. 7a). Establishing SACs in the PEC system requires semiconductor supports grown on conductive substrates, which not only stabilize SACs but also absorb solar light to generate electron–hole pairs and transfer charges. Single metal atoms can be supported by many typical metal oxide supports, such as Fe2O3, TiO2, WO3, ZnO and indium tin oxide.67,85–90 These semiconductor metal oxide materials can be used as photoelectrodes when fabricated on the conductive substrate, in the shape of films, nanowires, nanoflakes and others. Subsequently, the single metal atoms can be deployed on the surface of these semiconductor supports by wet chemical strategies or pyrolysis methods. Strong metal–support interactions between the single metal atoms and the semiconductor metal oxide supports will lead to the redistribution of electrons and promote charge transfer.91 Predictably, the introduction of atomically dispersed metal atoms to the semiconductor metal oxide supports as photoelectrodes can effectively increase the number of active sites, enhance catalytic efficacy per active site, and improve the adsorption of reactants and charge transfer, while maintaining the light absorption ability and adequate photogenerated charges.
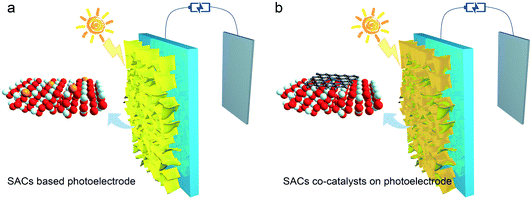 |
| Fig. 7 Schematic illustration of the (a) SAC based photoelectrode and (b) SAC co-catalysts on the photoelectrode in PEC. | |
In addition to the metal oxide support, g-C3N4 is another competent semiconductor support for applying SACs for PEC. SAC based g-C3N4 photocatalysts possess excellent photocatalytic performance.92 The synthetic approaches of g-C3N4 supported SACs are much simpler to operate than metal oxide supported SACs, while growing the g-C3N4 film on a conductive substrate is tricky but achievable. The flexible surface and tunable coordination environment of g-C3N4 as well as the tunable single metal centers can bring more possibilities to PEC applications. In addition to being employed as the photoelectrode, SACs can act as the co-catalysts for redox reactions when rationally deposited on photoelectrodes (Fig. 7b). Graphene and MOFs/COF based SACs have been verified noticeably active for many redox reactions.93–95 Graphene or MOFs/COF derived carbon materials can provide conductive channels to facilitate the charge transfer to the single metal active sites. Bridging the light absorbing photoelectrodes with the reactants, SAC based co-catalysts will trap photogenerated charges from the photoelectrodes, thus actuating the redox reactions.14,77 The improved adsorption and activation of reactants and promoted charge transfer and selective catalytic reactions by SAC based co-catalysts can enhance the overall activity and selectivity of PEC reactions.89 In general, rational design of SACs in photoelectrodes by composing SACs as one component of photoelectrodes or utilizing SACs as co-catalysts can integrate their advantages into the PEC system and significantly boost the PEC performance.
Scheme of the SAC based PEC system
Besides the creation of SACs on photoelectrodes, there exist other approaches to optimize the PEC system. A PEC system is usually composed of two photoelectrodes, or one photoelectrode and one counter electrode. The reactions at the counter electrodes affect the overall performance. As reported, optimized anodic electro-oxidation could lower the working potential for CO2 electroreduction and lower the electricity consumption by up to 53%.96 SACs exhibited excellent catalytic activity and selectivity in electrocatalytic reactions, which can be applied as the counter electrodes to reduce the overpotential and enhance the total production efficiency and economic viability, as illustrated in Fig. 8a.73 For example, the typical anodic reaction in the PEC CO2 reduction system is the oxygen evolution reaction (OER). SACs can optimize the reaction pathway with lower activation energy barriers and improve the activity for the OER. The coupling of SACs as counter electrodes will boost the overall performance of the PEC CO2 reduction system. Photovoltaic–electrocatalysis (PV–EC) is another feasible strategy to realize solar-to-chemical transformation using SACs (Fig. 8b).97,98 Analogous to the roles in PEC, SACs can work as active sites on the electrodes to drive anodic and cathodic reactions in a PV–EC system, thus improving the overall catalytic performance.
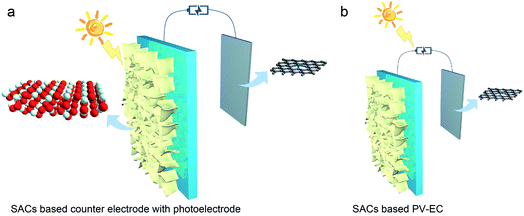 |
| Fig. 8 Schematic illustration of the (a) SAC based counter electrode in PEC and (b) SAC based PV–EC system. | |
Challenges of the SAC based PEC system
From the perspective of practical applications, the adaptability of the metal and support, the fabrication feasibility and the stability are the main challenges for applying SACs in PEC. Based on experimental and theoretical studies, the composition, configuration and coordination environment of metal sites and photoactive semiconductor supports are vital for the SAC based PEC reactions. The coupling effect of strong metal–support interaction and photogenerated charge migration will influence the adsorption and activation of reactants on single metal sites. In-depth studies of the working mechanisms through advanced spectroscopy and theoretical simulation are needed to fundamentally understand the different steps of SAC based PEC applications.99 For manufacturing SACs in PEC, one needs to consider the adaptability and the fabrication feasibility of growing semiconductor supports on conductive substrates and the establishment of atomically dispersed metal atoms on the surface. Reasonably regulating wet chemical and thermal treatment routes can realize the fabrication and effective integration of atomically dispersed metal atoms in PEC. Besides, SACs in PEC confront possible photocorrosion, while effective coordination and strong stabilization by the support can stabilize the atomically dispersed metal atoms.100
Conclusion and outlook
Photoelectrocatalysis is one potent strategy of artificial photosynthesis to harvest solar energy and upgrade chemicals. Practical applications toward industrial processes demand catalytic reactions with high activity, selectivity, stability and cost effectiveness in PEC. Much effort has been devoted to advancing the development of PEC. As mentioned above, SACs, a rising star in the field of catalysis, possess unique geometric and electronic properties.67 The deployment of SACs in the PEC system is a promising solution to resolving the current issues in terms of reaction sites and product selectivity. Improved chemisorption of reactants, promoted charge separation and transfer, optimal reaction pathway, selective catalytic reaction and maximal atom utilization efficiency by SACs can definitely boost the overall catalytic and economic efficiency of PEC as proven by experimental and theoretical results. These properties highly depend on the composition and configuration of SACs and the interaction between the support and metal sites. Effective integration and rational design of SACs are critical to the overall performance of PEC. Therefore, efforts should be made to rationalize and develop SAC based PEC systems to further achieve efficient artificial photosynthesis.
Based on structural design and performance optimization, it is imperative to achieve in-depth understanding on SAC based PEC. One needs to confirm the structure–function relationships between the SAC based photoelectrodes and the catalytic performance. The extracted structure–function relationships can guide the optimal design of SAC based PEC systems. SAC based PEC reactions involve complex steps, including directional adsorption of reactants, charge redistribution, alternative reaction pathways and selective catalytic reactions. Advanced characterization and theoretical simulation studies are required to comprehensively explore these critical steps, to further optimize SAC based PEC. On this basis, novel device design can realize the effectual utilization of SACs in PEC.
Overall, the implementation of SACs will provide optimal enhancement of catalytic activity and selectivity to compensate for the corresponding shortcomings of PEC. The unique advantages of SACs ensure their promising future applications, particularly for solar-to-chemical transformation. We hope that this perspective highlighting the challenges and opportunities of SACs for PEC will bring new insights and inspire new ideas for this research field.
Conflicts of interest
There are no conflicts to declare.
Acknowledgements
This work was financially supported in part by the National Key R&D Program of China (2020YFA0406103), NSFC (21725102, 51902253, 91961106, and 22005242), CAS Hundred Talent Program, and the Shaanxi Provincial Natural Science Foundation (2020JQ-778).
References
- T. P. Yoon, M. A. Ischay and J. Du, Nat. Chem., 2010, 2, 527–532 Search PubMed.
- P. Christopher, H. Xin, A. Marimuthu and S. Linic, Nat. Mater., 2012, 11, 1044–1050 Search PubMed.
- B. Wang, G. M. Biesold, M. Zhang and Z. Lin, Chem. Soc. Rev., 2021, 50, 6914–6949 Search PubMed.
- V. Augugliaro, G. Camera-Roda, V. Loddo, G. Palmisano, L. Palmisano, J. Soria and S. Yurdakal, J. Phys. Chem. Lett., 2015, 6, 1968–1981 Search PubMed.
- A. Paracchino, V. Laporte, K. Sivula, M. Gratzel and E. Thimsen, Nat. Mater., 2011, 10, 456–461 Search PubMed.
- F. X. Xiao, J. Miao, H. B. Tao, S. F. Hung, H. Y. Wang, H. B. Yang, J. Chen, R. Chen and B. Liu, Small, 2015, 11, 2115–2131 Search PubMed.
- T. Li, J. He, B. Pena and C. P. Berlinguette, Angew. Chem., Int. Ed., 2016, 55, 1769–1772 Search PubMed.
- Y. Yang, S. Wang, Y. Jiao, Z. Wang, M. Xiao, A. Du, Y. Li, J. Wang and L. Wang, Adv. Funct. Mater., 2018, 28, 1805698 Search PubMed.
- N. Zhang, R. Long, C. Gao and Y. Xiong, Sci. China Mater., 2018, 61, 771–805 Search PubMed.
- M. Nazemi and M. A. El-Sayed, Nano Energy, 2019, 63, 103886 Search PubMed.
- S. Zhou, K. Sun, J. Huang, X. Lu, B. Xie, D. Zhang, J. N. Hart, C. Y. Toe, X. Hao and R. Amal, Small, 2021, 17, e2100496 Search PubMed.
- X. Cui, W. Li, P. Ryabchuk, K. Junge and M. Beller, Nat. Catal., 2018, 1, 385–397 Search PubMed.
- X. Li, X. Yang, Y. Huang, T. Zhang and B. Liu, Adv. Mater., 2019, 31, 1902031 Search PubMed.
- C. Gao, J. Low, R. Long, T. Kong, J. Zhu and Y. Xiong, Chem. Rev., 2020, 120, 12175–12216 Search PubMed.
- S. Mitchell, E. Vorobyeva and J. Perez-Ramirez, Angew. Chem., Int. Ed., 2018, 57, 15316–15329 Search PubMed.
- Y. Chen, S. Ji, C. Chen, Q. Peng, D. Wang and Y. Li, Joule, 2018, 2, 1242–1264 Search PubMed.
- M. D. Marcinkowski, M. T. Darby, J. Liu, J. M. Wimble, F. R. Lucci, S. Lee, A. Michaelides, M. Flytzani-Stephanopoulos, M. Stamatakis and E. C. H. Sykes, Nat. Chem., 2018, 10, 325–332 Search PubMed.
- R. Long, Y. Li, Y. Liu, S. Chen, X. Zheng, C. Gao, C. He, N. Chen, Z. Qi, L. Song, J. Jiang, J. Zhu and Y. Xiong, J. Am. Chem. Soc., 2017, 139, 4486–4492 Search PubMed.
- Z. Teng, Q. Zhang, H. Yang, K. Kato, W. Yang, Y.-R. Lu, S. Liu, C. Wang, A. Yamakata, C. Su, B. Liu and T. Ohno, Nat. Catal., 2021, 4, 374–384 Search PubMed.
- X. F. Yang, A. Wang, B. Qiao, J. Li, J. Liu and T. Zhang, Acc. Chem. Res., 2013, 46, 1740–1748 Search PubMed.
- H. Xu, D. Cheng, D. Cao and X. C. Zeng, Nat. Catal., 2018, 1, 339–348 Search PubMed.
- B. Peng, H. Liu, Z. Liu, X. Duan and Y. Huang, J. Phys. Chem. Lett., 2021, 12, 2837–2847 Search PubMed.
- C. Cui, M. Heggen, W. D. Zabka, W. Cui, J. Osterwalder, B. Probst and R. Alberto, Nat. Commun., 2017, 8, 1341 Search PubMed.
- S. Tong, B. Fu, L. Gan and Z. Zhang, J. Mater. Chem. A, 2021, 9, 10731–10738 Search PubMed.
- Q. Song, J. Li, L. Wang, Y. Qin, L. Pang and H. Liu, J. Catal., 2019, 370, 176–185 Search PubMed.
-
M. E. Davis and R. J. Davis, Fundamentals of Chemical Reaction Engineering, McGraw-Hill, New York, NY, 2003 Search PubMed.
- T. Chen, L. Ye and T. W. B. Lo, J. Mater. Chem. A, 2021, 9, 18773–18784 Search PubMed.
- X. Du, J. Huang, J. Zhang, Y. Yan, C. Wu, Y. Hu, C. Yan, T. Lei, W. Chen, C. Fan and J. Xiong, Angew. Chem., Int. Ed., 2019, 58, 4484–4502 Search PubMed.
- C. M. Tian, M. Jiang, D. Tang, L. Qiao, H. Y. Xiao, F. E. Oropeza, J. P. Hofmann, E. J. M. Hensen, A. Tadich, W. Li, D. C. Qi and K. H. L. Zhang, J. Mater. Chem. A, 2019, 7, 11895–11907 Search PubMed.
- Y. Cao, L. Guo, M. Dan, D. E. Doronkin, C. Han, Z. Rao, Y. Liu, J. Meng, Z. Huang, K. Zheng, P. Chen, F. Dong and Y. Zhou, Nat. Commun., 2021, 12, 1675 Search PubMed.
- J. Greeley, J. K. Nørskov and M. Mavrikakis, Annu. Rev. Phys. Chem., 2002, 53, 319–348 Search PubMed.
- A. Nilsson, L. G. M. Pettersson, B. Hammer, T. Bligaard, C. H. Christensen and J. K. Nørskov, Catal. Lett., 2005, 100, 111–114 Search PubMed.
- W. H. Lai, Z. Miao, Y. X. Wang, J. Z. Wang and S. L. Chou, Adv. Energy Mater., 2019, 9, 1900722 Search PubMed.
- G. Meng, J. Zhang, X. Li, D. Wang and Y. Li, Appl. Phys. Rev., 2021, 8, 021321 Search PubMed.
- Y. Zhu, W. Peng, Y. Li, G. Zhang, F. Zhang and X. Fan, Small Methods, 2019, 3, 1800438 Search PubMed.
- J. Li, Q. Guan, H. Wu, W. Liu, Y. Lin, Z. Sun, X. Ye, X. Zheng, H. Pan, J. Zhu, S. Chen, W. Zhang, S. Wei and J. Lu, J. Am. Chem. Soc., 2019, 141, 14515–14519 Search PubMed.
- H. Su, W. Che, F. Tang, W. Cheng, X. Zhao, H. Zhang and Q. Liu, J. Phys. Chem. C, 2018, 122, 21108–21114 Search PubMed.
- Y. Xiao, C. Feng, J. Fu, F. Wang, C. Li, V. F. Kunzelmann, C.-M. Jiang, M. Nakabayashi, N. Shibata, I. D. Sharp, K. Domen and Y. Li, Nat. Catal., 2020, 3, 932–940 Search PubMed.
- M. M. Millet, G. Algara-Siller, S. Wrabetz, A. Mazheika, F. Girgsdies, D. Teschner, F. Seitz, A. Tarasov, S. V. Levchenko, R. Schlogl and E. Frei, J. Am. Chem. Soc., 2019, 141, 2451–2461 Search PubMed.
- M. D. Hossain, Y. Huang, T. H. Yu, W. A. Goddard III and Z. Luo, Nat. Commun., 2020, 11, 2256 Search PubMed.
- Z. Jakub, J. Hulva, M. Meier, R. Bliem, F. Kraushofer, M. Setvin, M. Schmid, U. Diebold, C. Franchini and G. S. Parkinson, Angew. Chem., Int. Ed., 2019, 58, 13961–13968 Search PubMed.
- J. Hulva, M. Meier, R. Bliem, Z. Jakub, F. Kraushofer, M. Schmid, U. Diebold, C. Franchini and G. S. Parkinson, Science, 2021, 371, 375–379 Search PubMed.
- W. Guo, Z. Wang, X. Wang and Y. Wu, Adv. Mater., 2021, 33, 2004287 Search PubMed.
- B. Han, Y. Guo, Y. Huang, W. Xi, J. Xu, J. Luo, H. Qi, Y. Ren, X. Liu, B. Qiao and T. Zhang, Angew. Chem., Int. Ed., 2020, 59, 11824–11829 Search PubMed.
- P. Hu, Z. Huang, Z. Amghouz, M. Makkee, F. Xu, F. Kapteijn, A. Dikhtiarenko, Y. Chen, X. Gu and X. Tang, Angew. Chem., Int. Ed., 2014, 53, 3418–3421 Search PubMed.
- B. Qiao, J.-X. Liang, A. Wang, C.-Q. Xu, J. Li, T. Zhang and J. J. Liu, Nano Res., 2015, 8, 2913–2924 Search PubMed.
- N. Cheng, S. Stambula, D. Wang, M. N. Banis, J. Liu, A. Riese, B. Xiao, R. Li, T. K. Sham, L. M. Liu, G. A. Botton and X. Sun, Nat. Commun., 2016, 7, 13638 Search PubMed.
- X. Zhang, J. Guo, P. Guan, C. Liu, H. Huang, F. Xue, X. Dong, S. J. Pennycook and M. F. Chisholm, Nat. Commun., 2013, 4, 1924 Search PubMed.
- X. Fang, Q. Shang, Y. Wang, L. Jiao, T. Yao, Y. Li, Q. Zhang, Y. Luo and H. L. Jiang, Adv. Mater., 2018, 30, 1705112 Search PubMed.
- C. Gao, S. Chen, Y. Wang, J. Wang, X. Zheng, J. Zhu, L. Song, W. Zhang and Y. Xiong, Adv. Mater., 2018, 30, 1704624 Search PubMed.
- J. Di, C. Chen, S. Z. Yang, S. Chen, M. Duan, J. Xiong, C. Zhu, R. Long, W. Hao, Z. Chi, H. Chen, Y. X. Weng, J. Xia, L. Song, S. Li, H. Li and Z. Liu, Nat. Commun., 2019, 10, 2840 Search PubMed.
- C. Xia, Y. Qiu, Y. Xia, P. Zhu, G. King, X. Zhang, Z. Wu, J. Y. T. Kim, D. A. Cullen, D. Zheng, P. Li, M. Shakouri, E. Heredia, P. Cui, H. N. Alshareef, Y. Hu and H. Wang, Nat. Chem., 2021, 13, 887–894 Search PubMed.
- G. Bae, H. Kim, H. Choi, P. Jeong, D. H. Kim, H. C. Kwon, K. S. Lee, M. Choi, H. S. Oh, F. Jaouen and C. H. Choi, JACS Au, 2021, 1, 586–597 Search PubMed.
- Y. Jin, F. Lu, D. Yi, J. Li, F. Zhang, T. Sheng, F. Zhan, Y. n. Duan, G. Huang, J. Dong, B. Zhou, X. Wang and J. Yao, CCS Chem., 2021, 3, 1453–1462 Search PubMed.
- Y. Kwon, T. Y. Kim, G. Kwon, J. Yi and H. Lee, J. Am. Chem. Soc., 2017, 139, 17694–17699 Search PubMed.
- P. Zhou, H. Chen, Y. Chao, Q. Zhang, W. Zhang, F. Lv, L. Gu, Q. Zhao, N. Wang, J. Wang and S. Guo, Nat. Commun., 2021, 12, 4412 Search PubMed.
- S. Yang, J. Kim, Y. J. Tak, A. Soon and H. Lee, Angew. Chem., Int. Ed., 2016, 55, 2058–2062 Search PubMed.
- Y. Wang, J. Liu, Y. Wang, A. M. Al-Enizi and G. Zheng, Small, 2017, 13, 1701809 Search PubMed.
- D. Liu, J. C. Liu, W. Cai, J. Ma, H. B. Yang, H. Xiao, J. Li, Y. Xiong, Y. Huang and B. Liu, Nat. Commun., 2019, 10, 1779 Search PubMed.
- M. Xiao, J. Zhu, G. Li, N. Li, S. Li, Z. P. Cano, L. Ma, P. Cui, P. Xu, G. Jiang, H. Jin, S. Wang, T. Wu, J. Lu, A. Yu, D. Su and Z. Chen, Angew. Chem., Int. Ed., 2019, 58, 9640–9645 Search PubMed.
- L. Nie, D. Mei, H. Xiong, B. Peng, Z. Ren, X. I. P. Hernandez, A. DeLaRiva, M. Wang, M. H. Engelhard, L. Kovarik, A. K. Datye and Y. Wang, Science, 2017, 358, 1419–1423 Search PubMed.
- S. Back, J. Lim, N. Y. Kim, Y. H. Kim and Y. Jung, Chem. Sci., 2017, 8, 1090–1096 Search PubMed.
- Y. Xu, M. Chu, F. Liu, X. Wang, Y. Liu, M. Cao, J. Gong, J. Luo, H. Lin, Y. Li and Q. Zhang, Nano Lett., 2020, 20, 6865–6872 Search PubMed.
- I. Roger, M. A. Shipman and M. D. Symes, Nat. Rev. Chem., 2017, 1, 0003 Search PubMed.
- J. L. White, M. F. Baruch, J. E. Pander III, Y. Hu, I. C. Fortmeyer, J. E. Park, T. Zhang, K. Liao, J. Gu, Y. Yan, T. W. Shaw, E. Abelev and A. B. Bocarsly, Chem. Rev., 2015, 115, 12888–12935 Search PubMed.
- B. Xia, Y. Zhang, J. Ran, M. Jaroniec and S. Z. Qiao, ACS Cent. Sci., 2021, 7, 39–54 Search PubMed.
- S. K. Kaiser, Z. Chen, D. Faust Akl, S. Mitchell and J. Perez-Ramirez, Chem. Rev., 2020, 120, 11703–11809 Search PubMed.
- C. Zhu, S. Fu, Q. Shi, D. Du and Y. Lin, Angew. Chem., Int. Ed., 2017, 56, 13944–13960 Search PubMed.
- G. Yang, Y. Li, H. Lin, X. Ren, D. Philo, Q. Wang, Y. He, F. Ichihara, S. Luo, S. Wang and J. Ye, Small Methods, 2020, 4, 2000577 Search PubMed.
- Y. Qin, J. Wen, L. Zheng, H. Yan, L. Jiao, X. Wang, X. Cai, Y. Wu, G. Chen, L. Chen, L. Hu, W. Gu and C. Zhu, Nano Lett., 2021, 21, 1879–1887 Search PubMed.
- J. Zhang, G. Zhang, H. Lan, H. Liu and J. Qu, Chem. Eng. J., 2022, 428, 130373 Search PubMed.
- T. Wang, L. Guo, H. Pei, S. Chen, R. Li, J. Zhang and T. Peng, Small, 2021, 2102957 Search PubMed.
- W. Wang, S. Zhang, Y. Liu, L.-R. Zheng, G. Wang, Y. Zhang, H. Zhang and H. Zhao, Chin. Chem. Lett., 2021, 32, 805–810 Search PubMed.
- Y. C. Hao, L. W. Chen, J. Li, Y. Guo, X. Su, M. Shu, Q. Zhang, W. Y. Gao, S. Li, Z. L. Yu, L. Gu, X. Feng, A. X. Yin, R. Si, Y. W. Zhang, B. Wang and C. H. Yan, Nat. Commun., 2021, 12, 2682 Search PubMed.
- Y. Wang, X. Zhao, D. Cao, Y. Wang and Y. Zhu, Appl. Catal., B, 2017, 211, 79–88 Search PubMed.
- F. Wang, Y. Wang, Y. Feng, Y. Zeng, Z. Xie, Q. Zhang, Y. Su, P. Chen, Y. Liu, K. Yao, W. Lv and G. Liu, Appl. Catal., B, 2018, 221, 510–520 Search PubMed.
- L. Yi, F. Lan, J. Li and C. Zhao, ACS Sustainable Chem. Eng., 2018, 6, 12766–12775 Search PubMed.
- J. Li, H. Huang, P. Liu, X. Song, D. Mei, Y. Tang, X. Wang and C. Zhong, J. Catal., 2019, 375, 351–360 Search PubMed.
- X. H. Jiang, L. S. Zhang, H. Y. Liu, D. S. Wu, F. Y. Wu, L. Tian, L. L. Liu, J. P. Zou, S. L. Luo and B. B. Chen, Angew. Chem., Int. Ed., 2020, 59, 23112–23116 Search PubMed.
- Z. Zeng, Y. Su, X. Quan, W. Choi, G. Zhang, N. Liu, B. Kim, S. Chen, H. Yu and S. Zhang, Nano Energy, 2020, 69, 104409 Search PubMed.
- M. Kou, W. Liu, Y. Wang, J. Huang, Y. Chen, Y. Zhou, Y. Chen, M. Ma, K. Lei, H. Xie, P. K. Wong and L. Ye, Appl. Catal., B, 2021, 291, 120146 Search PubMed.
- J.-H. Zhang, W. Yang, M. Zhang, H.-J. Wang, R. Si, D.-C. Zhong and T.-B. Lu, Nano Energy, 2021, 80, 105542 Search PubMed.
- Z. Han, Y. Zhao, G. Gao, W. Zhang, Y. Qu, H. Zhu, P. Zhu and G. Wang, Small, 2021, 17, 2102089 Search PubMed.
- J. Deng, Y. Su, D. Liu, P. Yang, B. Liu and C. Liu, Chem. Rev., 2019, 119, 9221–9259 Search PubMed.
- H. V. Thang, G. Pacchioni, L. DeRita and P. Christopher, J. Catal., 2018, 367, 104–114 Search PubMed.
- B. Han, R. Lang, H. Tang, J. Xu, X.-K. Gu, B. Qiao and J. Liu, Chin. J. Catal., 2019, 40, 1847–1853 Search PubMed.
- R. Gao, J. Wang, Z.-F. Huang, R. Zhang, W. Wang, L. Pan, J. Zhang, W. Zhu, X. Zhang, C. Shi, J. Lim and J.-J. Zou, Nat. Energy, 2021, 6, 614–623 Search PubMed.
- J. Park, S. Lee, H. E. Kim, A. Cho, S. Kim, Y. Ye, J. W. Han, H. Lee, J. H. Jang and J. Lee, Angew. Chem., Int. Ed., 2019, 58, 16038–16042 Search PubMed.
- G. Cha, I. Hwang, S. Hejazi, A. S. Dobrota, I. A. Pasti, B. Osuagwu, H. Kim, J. Will, T. Yokosawa, Z. Badura, S. Kment, S. Mohajernia, A. Mazare, N. V. Skorodumova, E. Spiecker and P. Schmuki, iScience, 2021, 24, 102938 Search PubMed.
- D. Lebedev, R. Ezhov, J. Heras-Domingo, A. Comas-Vives, N. Kaeffer, M. Willinger, X. Solans-Monfort, X. Huang, Y. Pushkar and C. Copéret, ACS Cent. Sci., 2020, 6, 1189–1198 Search PubMed.
- C. Cheng, W. H. Fang, R. Long and O. V. Prezhdo, JACS Au, 2021, 1, 550–559 Search PubMed.
- L. Zeng, C. Dai, B. Liu and C. Xue, J. Mater. Chem. A, 2019, 7, 24217–24221 Search PubMed.
- J. N. Tiwari, A. N. Singh, S. Sultan and K. S. Kim, Adv. Energy Mater., 2020, 10, 2000280 Search PubMed.
- M. B. Gawande, P. Fornasiero and R. Zbořil, ACS Catal., 2020, 10, 2231–2259 Search PubMed.
- T. Sun, L. Xu, D. Wang and Y. Li, Nano Res., 2019, 12, 2067–2080 Search PubMed.
- S. Verma, S. Lu and P. J. A. Kenis, Nat. Energy, 2019, 4, 466–474 Search PubMed.
- Y. Shi, C. Zhao, H. Wei, J. Guo, S. Liang, A. Wang, T. Zhang, J. Liu and T. Ma, Adv. Mater., 2014, 26, 8147–8153 Search PubMed.
- N. Li, C. Zhu, J. Zhang, H. Jing, J. Hu, C. Hao and Y. Shi, Chem. Commun., 2021, 57, 5302–5305 Search PubMed.
- H. T. Lien, S. T. Chang, P. T. Chen, D. P. Wong, Y. C. Chang, Y. R. Lu, C. L. Dong, C. H. Wang, K. H. Chen and L. C. Chen, Nat. Commun., 2020, 11, 4233 Search PubMed.
- J. Jones, H. Xiong, A. T. DeLaRiva, E. J. Peterson, H. Pham, S. R. Challa, G. Qi, S. Oh, M. H. Wiebenga, X. I. Pereira Hernandez, Y. Wang and A. K. Datye, Science, 2016, 353, 150–154 Search PubMed.
|
This journal is © The Royal Society of Chemistry 2022 |