DOI:
10.1039/D2NR04173A
(Paper)
Nanoscale, 2022,
14, 17222-17229
Green synthesis of highly stable CsPbBr3 perovskite nanocrystals using natural deep eutectic solvents as solvents and surface ligands†
Received
28th July 2022
, Accepted 10th October 2022
First published on 11th October 2022
Abstract
Perovskite nanocrystals (PNCs) have attracted widespread attention as promising materials for the optoelectronic field due to their remarkable photophysical properties and structural tunability. However, their poor stability and the use of toxic organic solvents in the preparation process have severely restricted their practical applications. Herein, a facile, rapid and toxic organic solvent-free synthesis strategy of CsPbBr3 PNCs was developed for the first time via the ligand-assisted reprecipitation (LARP) method using natural deep eutectic solvents (NADESs) as solvents and surface ligands. In this method, the NADESs not only functioned as solvents for green synthesis, but also served simultaneously as surface ligands of CsPbBr3 PNCs to significantly improve their optical properties and stability. The as-synthesized CsPbBr3 PNCs exhibited high photoluminescence quantum yield (PLQY, ∼96.8%), narrow full width at half-maximum (FWHM, ∼18.8 nm) and a high stability that retained 82.9% of PL intensity after 70 days. This work provides a new strategy for the green synthesis of PNCs, which promises feasibility for the industrial large-scale synthesis of high-quality PNCs.
Introduction
In recent years, perovskite nanocrystals (PNCs) have received much attention because of their excellent optoelectronic properties, such as narrow full width at half-maximum (FWHM), high photoluminescence quantum yield (PLQY), low exciton binding energy, tunable band gap, and low defect density.1–3 They show great potential for application in the fields of light-emitting diodes (LED),4,5 solar cells,6–8 lasers9 and photocatalysis.10–12 Currently, the most common synthesis methods for PNCs are the hot-injection method13 and the ligand-assisted reprecipitation (LARP) method.14,15 In the LARP method, PNCs are obtained through the regulation of volume and concentration of solvents and precursors. Precursors are firstly dissolved in good solvents, such as dimethyl sulfoxide (DMSO) and dimethylformamide (DMF), and then transferred into poor solvents (antisolvents), such as toluene and n-octane, to form a supersaturated solution and precipitate the crystals under the assistance of ligands, such as oleic acid (OA) and oleylamine (OAm). This method is promising due to its ease of operation, low equipment requirement and mild operational temperatures. However, there are two disadvantages of the LARP method. On the one hand, a large amount of toxic organic solvents is inevitably used, which significantly limits their applications. On the other hand, the proton exchange between OA and OAm may induce the ligands to be easily lost from the surface of PNCs during the purification process or storage period, resulting in poor stability of PNCs.
Recently, great efforts have been devoted to developing effective ways to solve the above problems. Li16et al. developed a novel low-toxicity antisolvent synthesis (LTAS) process based on tetraethyl orthosilicate (TEOS) to obtain monodispersed and luminescent all-inorganic PNCs. Wang's17 group proposed a facile method to synthesize green CsPbBr3 PNCs using the ionic liquid (IL) 1-butyl-3-methylimidazolium bromide ([Bmim]Br). Luo18et al. recently reported the synthesis of blue-emission CsPbBr3 PNCs with the assistance of hydrophobic ILs. However, ILs have some disadvantages, such as complex preparation processes, high cost and poor biocompatibility.19,20 In addition, Tan21et al. proposed a novel strategy using octylphosphonic acid (OPA) as the capping ligand to solve the problem of ligands loss and improve the stability of CsPbX3 PNCs. Mishra22et al. reported bromopropane as a novel bromine precursor for the completely amine-free colloidal synthesis of CsPbBr3 PNCs. Research on the direction of amine-free synthesis emerges in an endless stream.23–25
Deep eutectic solvents (DESs), introduced by Abbott26et al. in 2003, have been regarded as a new class of green solvents. DESs are low-eutectic mixtures of compounds composed of a hydrogen bond donor (HBA) and a hydrogen bond acceptor (HBD).27 They possess not only similar favorable properties to ILs, but also the advantage of being easy to prepare, cost-effective, and biodegradable.28,29 Among them, natural deep eutectic solvents (NADESs), composed of natural compounds such as carboxylic acids, sugars, alcohols, amines and amino acids, have attracted more attention because they fully meet the green chemistry principles.30 As the most promising alternative to toxic organic solvents, NADESs have been applied successfully in the fields of catalysis,31,32 extraction,33,34 electrochemistry35,36 and organic synthesis.37,38 Furthermore, the –COOH, –OH, and –NH2 groups in these natural compounds can coordinate with metal ions, which provides feasibility for using NADESs as ligands. To the best of our knowledge, NADESs used simultaneously as solvents and surface ligands in the synthesis of PNCs have not been reported so far.
Herein, a facile, rapid and toxic organic solvent-free synthesis strategy for CsPbBr3 PNCs was developed for the first time via the ligand-assisted reprecipitation (LARP) method using NADESs as solvents and surface ligands. First, NADESs, consisting of thymol as the HBA and L-lactic acid (Thy
:
LacA) and n-octanol (Thy
:
Oct) as the HBD, were used as good solvents, and a NADES consisting of thymol as the HBA and decanoic acid (Thy
:
DecA) as the HBD was used as antisolvent for the green synthesis of NADES-CsPbBr3 PNCs. Moreover, the –COOH and –OH groups in Thy
:
Oct and Thy
:
DecA can polychelate with Pb2+ and provide a strong interaction with CsPbBr3 PNCs. Thereby, Thy
:
Oct and Thy
:
DecA can also act as surface ligands to significantly enhance the optical properties and stability of CsPbBr3 PNCs. This facile, rapid and green synthesis method of PNCs will provide a new avenue for their sustainable industrial production in various fields.
Experimental
Reagents and solutions
DL-Menthol (C10H20O, 98%), thymol (C10H14O, 98%), L-lactic acid (C3H6O3, 90%), n-octanoic acid (C8H16O2, 99%), decanoic acid (C10H20O2, 99%), lauric acid (C12H24O2, 98%), oleic acid (C18H34O2, AR), ethanol (C2H6O, 99%), n-butanol (C4H10O, 99%), n-hexanol (C6H14O, 99%), n-octanol (C8H18O, 99%), and cesium bromide (CsBr, 99.5%) were obtained from Aladdin Industrial Co. Ltd (Shanghai, China). n-Decanol (C10H22O, 98%), lead(II) bromide (PbBr2, 99%), and oleylamine (C18H37N, 80–90%) were purchased from Macklin Biochemical Technology Co. Ltd. (Shanghai, China). All reagents and solutions were used as received without further purification.
Apparatus
Fourier transform infrared (FTIR) spectra (NICOLET iS 10) and 1H NMR (Avance 500 MHz; Bruker) were applied to determine the formation of NADESs. The phase of the NADES-CsPbBr3 PNCs was identified via an X-ray diffractometer (XRD, Rigaku Ultima IV). Ultraviolet–visible (UV-vis) absorption spectra were tested using a UV-vis spectrophotometer (Specord plus 200). Photoluminescence (PL) emission spectra were recorded using a spectrometer (Techcomp FL970 Plus). NADES-CsPbBr3 PNCs were characterized using luminescence decay curves and photoluminescence quantum yields (PLQY; FLS980, Edinburgh) under excitation at 365 nm. Transmission electron microscopy (TEM) images and high-resolution TEM images were taken on a JEM-2100.
Preparation of NADESs
In this study, different NADESs were prepared by the following steps. Firstly, the [HBA
:
HBD] of all NADESs was mixed at a molar ratio of 1
:
1, and using DL-menthol and thymol as the HBA, combined with eight different HBDs comprising L-lactic acid, n-octanoic acid, decanoic acid, lauric acid, ethanol, n-butanol, n-hexanol and n-octanol. Secondly, the mixture was heated and stirred at 80 °C and 700 rpm for 90 minutes until a clear homogeneous liquid was obtained, which indicated the formation of NADESs. The as-obtained NADESs were as follows: DL-menthol
:
L-lactic acid (Man
:
LacA), DL-menthol
:
n-octanoic acid (Man
:
OctA), DL-menthol
:
decanoic acid (Man
:
DecA), DL-menthol
:
lauric acid (Man
:
LauA), thymol
:
n-octanoic acid (Thy
:
OctA), thymol
:
lauric acid (Thy
:
LauA), thymol
:
ethanol (Thy
:
Eth), thymol
:
n-butanol (Thy
:
But), thymol
:
n-hexanol (Thy
:
Hex), Thy
:
LacA, Thy
:
Oct and Thy
:
DecA, as shown in Table S1.†
Synthesis of NADES-CsPbBr3 PNCs
The synthesis processes of NADESs and NADES-CsPbBr3 PNCs are illustrated in Fig. 1. and the NADESs used are listed in Table S2.† In a typical procedure, under vigorous stirring at 50 °C, 0.02 mmol of PbBr2, 100 μL of OA and 75 μL of OAm were dissolved in 1 mL of Thy
:
Oct, and 0.3 mmol of CsBr were dissolved in 1 mL of Thy
:
Lac to form Pb- and Cs-precursor solutions. Then, 600 μL of Pb-precursor and 119 μL of Cs-precursor solution (molar ratio of Cs
:
Pb = 3
:
1) was quickly added to 3 mL of Thy
:
DecA at the same time under vigorous stirring, and bright blue-green PL emission was observed immediately. After 5 hours, the CsPbBr3 colloidal solution was centrifuged at 10
000 rpm for 10 min to obtain NADES-CsPbBr3 PNCs. Finally, the product was washed and stored in n-hexane.
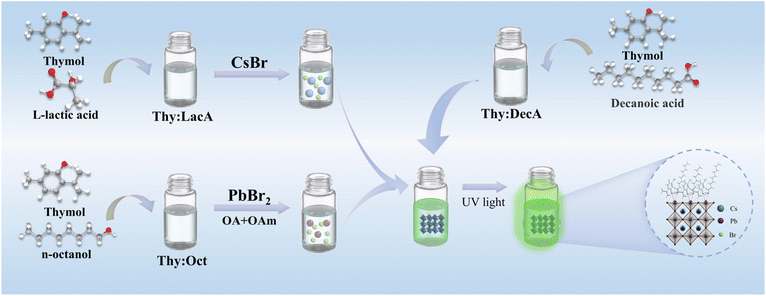 |
| Fig. 1 Schematic illustration of synthetic procedure for NADES-CsPbBr3 PNCs. | |
Fabrication of the white LED device
First, the as-synthesized NADES-CsPbBr3 PNCs powders were blended with silicone gel A and B (A
:
B = 1
:
4) and mixed by stirring to obtain a fine dispersion of NADES-CsPbBr3 PNCs. Then the mixture was vacuumed to discharge air bubbles. A WLED device was constructed by combining a 480 nm GaN LED blue chip with the green-emitting NADES-CsPbBr3 PNC glass and commercial red-emitting KSF phosphor.
Results and discussion
Characterization of NADESs
NADESs were formed by the hydrogen bond between HBA and HBD. To prove the formation of Thy
:
Oct, the FTIR and 1H NMR characterizations of Thy
:
Oct and their constituents were performed, as shown in Fig. 2. From the FTIR spectra of Thy
:
Oct and its constituents (Fig. 2a), the broad absorption bands associated with the stretching vibrations of O–H groups were observed in the range 3100–3400 cm−1. Compared with the O–H stretching bands of thymol and octanol respectively located at 3231.40 cm−1 and 3317.54 cm−1, the O–H stretching band of Thy
:
Oct, located at 3342.30 cm−1, was shifted to a higher wavenumber. This result could be regarded as the contribution of the hydrogen bond formation between HBA and HBD, which indicated the formation of Thy
:
Oct. The 1H NMR spectra of Thy
:
Oct and its constituents are presented in Fig. 2b. The characteristic H resonance peaks of thymol and octanol can be observed in spectrum of Thy
:
Oct. Furthermore, the peaks of the –OH of thymol from δ = 4.78 to δ = 6.79 and the –OH group of octanol from δ = 3.74 to δ = 2.88 can be observed. The chemical shift value changes because the formation of hydrogen bonds changes the electron density around the hydrogen nucleus, verifying the formation of Thy
:
Oct. Similarly, as shown in Fig. S1 and S2,† the formation of Thy
:
LacA and Thy
:
DecA can also be proved by FT-IR and 1H NMR.39,40
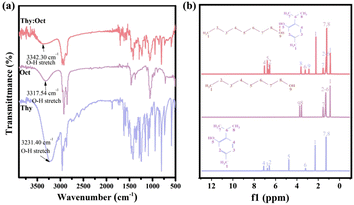 |
| Fig. 2 (a) The FT-IR spectra of thymol, n-octanol and Thy : Oct; (b) 1H NMR spectra of thymol, n-octanol and Thy : Oct. | |
The polarity of the NADESs can be evaluated based on the solvatochromic method. Nile red solvatochromic dyes comprise one of the most widely used probes that has been extensively employed to measure the polarity of NADESs.41 The solvatochromic parameter ENR can be calculated using the following equation:
where
ENR is in kcal mol
−1 and
λmax is the wavelength of the maximum absorption of the probe in the NADESs. High
ENR values correspond to lower polarity and low
ENR values correspond to higher polarity of NADESs. The absorbance spectra of Nile red dissolved in Thy
![[thin space (1/6-em)]](https://www.rsc.org/images/entities/char_2009.gif)
:
![[thin space (1/6-em)]](https://www.rsc.org/images/entities/char_2009.gif)
LacA, Thy
![[thin space (1/6-em)]](https://www.rsc.org/images/entities/char_2009.gif)
:
![[thin space (1/6-em)]](https://www.rsc.org/images/entities/char_2009.gif)
Oct and Thy
![[thin space (1/6-em)]](https://www.rsc.org/images/entities/char_2009.gif)
:
![[thin space (1/6-em)]](https://www.rsc.org/images/entities/char_2009.gif)
DecA is shown in Fig. S3.
† The
λmax values were located at 592 nm, 566 nm and 563 nm respectively. The polarity values of Thy
![[thin space (1/6-em)]](https://www.rsc.org/images/entities/char_2009.gif)
:
![[thin space (1/6-em)]](https://www.rsc.org/images/entities/char_2009.gif)
LacA, Thy
![[thin space (1/6-em)]](https://www.rsc.org/images/entities/char_2009.gif)
:
![[thin space (1/6-em)]](https://www.rsc.org/images/entities/char_2009.gif)
Oct and Thy
![[thin space (1/6-em)]](https://www.rsc.org/images/entities/char_2009.gif)
:
![[thin space (1/6-em)]](https://www.rsc.org/images/entities/char_2009.gif)
DecA as calculated by
ENR scales were 48.3 kcal mol
−1, 50.5 kcal mol
−1 and 50.7 kcal mol
−1 respectively.
Characterization of NADES-CsPbBr3 PNCs
The phase of NDES-CsPbBr3 NCs were conducted via X-ray diffraction (XRD) (Fig. 3a). The diffraction peaks of NADES-CsPbBr3 PNCs and typical CsPbBr3 PNCs were well coincident with a monoclinic phase of CsPbBr3 (PDF card no. 00-018-0364). The diffraction peaks located at 2
θ = 15.1°, 21.5°, 30.7°, 34.2°, 37.6° and 43.7° can be assigned to (001), (110), (200), (210), (211) and (202) crystal planes, respectively. Furthermore, compared with the typical LARP-synthesized CsPbBr3 PNCs, NADES-CsPbBr3 PNCs exhibited higher and sharper peaks indicating NADES-CsPbBr3 PNCs have better crystalline quality. The transmission electron microscopy (TEM) graph of NADES-CsPbBr3 PNCs indicated that NADES-CsPbBr3 PNCs possess a cubic shape and is well dispersed (Fig. 3b and c). Moreover, the high-resolution TEM image (inset of Fig. 3c) revealed a highly ordered crystalline lattice and the lattice spacing was calculated to be 0.29 nm, which corresponds to the (200) lattice space of monoclinic CsPbBr3 PNCs. The size ranged from 7 to 18 nm and the average widths were around 11.8 nm as illustrated in Fig. 3d.
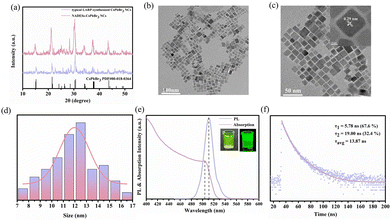 |
| Fig. 3 (a) The XRD patterns of CsPbBr3 PNCs synthesized by two methods; (b and c) TEM images of NADES-CsPbBr3 PNCs. Inset in (c) shows the corresponding HRTEM image; (d) size distribution of NADES-CsPbBr3 PNCs; (e) absorption and PL spectra of NADES-CsPbBr3 PNCs. Inset in (e) shows the photograph of NADES-CsPbBr3 PNCs under daylight (left) and under UV light (right); (f) the decay curve of NADES-CsPbBr3 PNCs. | |
Optical properties of the as-synthesized NADES-CsPbBr3 PNCs were also studied. The UV-vis absorption and PL emission spectra of NADES-CsPbBr3 PNCs are presented in Fig. 3e. The UV-vis absorption spectra showed an obvious shoulder peak at about 504 nm. Meanwhile, a single strong PL peak appeared at around 511 nm with a narrow FWHM of ∼18.8 nm. As shown in Fig. S4,† the as-synthesized NADES-CsPbBr3 NCs exhibited a high PLQY value of ∼96.8%. The inset of Fig. 3e shows a NADES-CsPbBr3 PNC yellow-green solution under daylight, and bright green light emission under UV light. Furthermore, the time-resolved PL measurements were carried out to verify the exciton recombination dynamics, as shown in Fig. 3f. The PL decay could be well fitted by a biexponential function,15
where
A,
A1, and
A2 are constants and
τ1 and
τ2 are two biexponential lifetimes with amplitudes
A1 and
A2, respectively. The average lifetime (
τave) can be calculated as
The obtained NADES-CsPbBr3 PNCs exhibit two lifetimes (τ): τ1 of 5.78 ns accounting for 67.6%, and τ2 of 19.00 ns accounting for 32.4%, respectively. The short decay time (τ1) originated from excitonic radiative recombination, while the long decay time originated from a longer charge trapping/detrapping process.42 The longer τ2 of NADES-CsPbBr3 PNCs is consistent with its higher surface defects, while the smaller proportion of τ2 indicates the low defect density of the synthesized NADES-CsPbBr3 PNCs. However, this may need further spectroscopic study.
The selection of NADESs
The synthesis of CsPbBr3 PNCs by the supersaturation recrystallization method was achieved through dissolving the precursors in a good solvent, and then transferring them into a poor solvent (antisolvents) to form a supersaturated solution and precipitate crystals. Therefore, the solubility of precursors in the related solvents plays an important role in this method. The solubility of CsBr and PbBr2 in 13 kinds of NADESs was investigated and is summarized in Table S3.† As can be seen, CsBr could dissolve in Thy
:
LacA, and PbBr2 could dissolve in four NADESs, comprising Thy
:
Oct, Thy
:
But, Thy
:
Hex and Thy
:
Oct. To further obtain the best dissolve solvents of PbBr2, four different schemes (Table 1) were designed to synthesize NADES-CsPbBr3 PNCs and the PL spectra of the products were investigated (Fig. 4a). As shown in Fig. 4a, the NADES-CsPbBr3 PNCs synthesized via the TLTO scheme exhibited the best optical properties. Hence, Thy
:
LacA and Thy
:
Oct were used as the dissolving solvents for CsBr and PbBr2, respectively. Furthermore, the optimal NADES to be used as an antisolvent was also evaluated. It can be seen from Fig. 4b and Table S4† that the NADES-CsPbBr3 PNC synthesized using Thy
:
DecA as an antisolvent showed the strongest PL intensity. Therefore, Thy
:
DecA was selected as the antisolvent in this experiment.
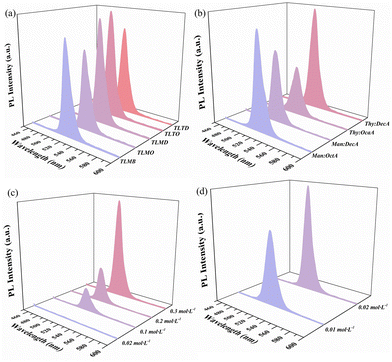 |
| Fig. 4 (a) PL spectra of NADES-CsPbBr3 PNCs synthesized by different schemes; (b) PL spectra of NADES-CsPbBr3 PNCs synthesized by different antisolvents; (c) PL spectra of NADES-CsPbBr3 PNCs synthesized by different concentration of Cs-precursor; (d) PL spectra of NADES-CsPbBr3 PNCs synthesized by different concentrations of Pb-precursor. | |
Table 1 Different combination schemes used to synthesize NADES-CsPbBr3 PNCs
Name |
CsBr |
PbBr2 |
Antisolvent |
TLTE |
Thy : LacA (0.3 mol·L−1) |
Thy : Eth (0.02 mol·L−1) |
Thy : DecA (3 mL) |
TLTB |
Thy : LacA (0.3 mol·L−1) |
Thy : But (0.02 mol·L−1) |
Thy : DecA (3 mL) |
TLTH |
Thy : LacA (0.3 mol·L−1) |
Thy : Hex (0.02 mol·L−1) |
Thy : DecA (3 mL) |
TLTO |
Thy : LacA (0.3 mol·L−1) |
Thy : Oct (0.02 mol·L−1) |
Thy : DecA (3 mL) |
Effect of the concentration of Cs- and Pb-precursor solutions
As the structural disintegration of PNCs is likely to occur in a good solvent, it is necessary to use highly concentrated precursor solutions to reduce the amount of good solvent required in the LARP method. To investigate the influence of the concentration of Cs- and Pb-precursor solutions on the luminescence intensity of NADES-CsPbBr3 PNCs in this method, a series of experiments were studied using various concentrations of Cs- and Pb-precursor solutions (Table S5 and S6†). Fig. 4c and d showed that the luminescence intensity of NADES-CsPbBr3 PNCs was increased with the increase in the concentration of Cs- and Pb-precursor solutions, and the highest intensity was obtained at up to 0.3 mol L−1 Cs-precursor solutions and 0.02 mol L−1 Pb-precursor solutions, respectively.
Optimum molar ratios of Pb- and Cs-precursor
The molar ratios of Pb- and Cs-precursor (nCs/nPb) significantly affect the phase transformation of PNCs. Fig. 5 presents the XRD patterns of PNCs with different nCs/nPb ratios (1
:
3, 1
:
1 and 3
:
1). The phase of the PNCs gradually changed from CsPb2Br5 to CsPbBr3 PNCs with the increase in nCs/nPb. Different from the pure phase of CsPbBr3 PNCs formed in the typical method, mixed phases of CsPb2Br5 and CsPbBr3 PNCs were obtained with nCs/nPb = 1
:
1 in this method. This was probably caused by the strong coordination between Cs+ and Thy
:
LacA. When nCs/nPb reached 3
:
1, the pure phase of CsPbBr3 PNCs was obtained, for which the XRD pattern was assigned to three dimensional CsPbBr3 (PDF card no.00-018-0364). Hence, nCs/nPb = 3
:
1 was selected in our experiment.
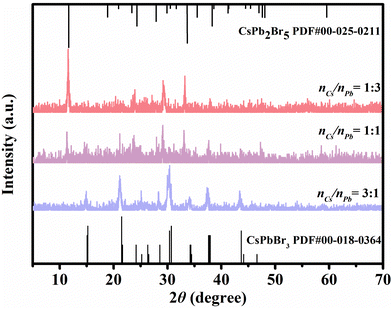 |
| Fig. 5 XRD patterns of PNCs synthesized with different molar ratios of CsBr and PbBr2. | |
Stability of NADES-CsPbBr3 PNCs
To avoid the reduction of the applied device performance by the residual organic ligands, purification processes of PNCs are necessary. Unfortunately, in the typical LARP-synthesized CsPbBr3 PNCs, the shift of the UV-vis peak and the PL quenching phenomenon were observed because of severe aggregation of PNCs due to insufficient surface ligands after only two washing cycles (Fig. S5†). According to previous reports,43,44 the OA/OAm ligands were easily washed off because of their proton exchange and weak coordination ability with PNCs. In contrast, NADES-CsPbBr3 PNCs exhibited much higher stability during purification processes. NADESs have a stronger coordination ability with PNCs and act as better surface ligands, which prevents the aggregation of PNCs. As shown in Fig. 6a and b, the UV-vis absorption and PL spectra of NADES-CsPbBr3 PNCs barely changed after five cycles of the same washing processes.
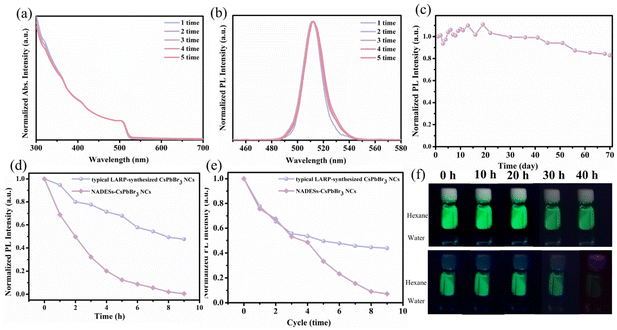 |
| Fig. 6 (a) The UV-vis patterns of NADES-CsPbBr3 NC by different centrifugation times; (b) PL spectra of NADES-CsPbBr3 PNCs by different centrifugation times; (c) time stability of NADES-CsPbBr3 PNCs; (d) light stability of CsPbBr3 PNCs; (e) thermal stability of CsPbBr3 PNCs; (f) water stability of CsPbBr3 PNCs. Above is NADES-CsPbBr3 PNCs; below is typical LARP-synthesized CsPbBr3 PNCs. Upper layer, hexane; bottom layer, water. | |
The storage, light, thermal and water stability of NADES-CsPbBr3 PNCs were further determined. The storage stability of the NADES-CsPbBr3 PNCs was evaluated in ambient conditions and the results are shown in Fig. 6c. It can be seen that the PL emission intensity could preserve 82.9% retention after 70 days. As shown in Fig. 6d, the typical LARP-synthesized CsPbBr3 PNCs only kept 0.6% of the initial PL intensity under irradiation with a UV lamp after 9 h, while 47.6% of the initial PL intensity of NADES-CsPbBr3 PNCs was still reserved. In addition, as exhibited in Fig. 6e, the typical LARP-synthesized CsPbBr3 PNCs kept 0.7% of the initial PL intensity after nine heating cycles at 85 °C, while the PL intensity of NADES-CsPbBr3 PNCs could still be maintained at 43.8%. Finally, the water stability of CsPbBr3 PNCs was tested by 2 mL of hexane solution put on the top of 1 mL of water (Fig. 6f). For the typical LARP-synthesized CsPbBr3 PNCs, green fluorescence disappeared completely after 40 h, while the NADES-CsPbBr3 PNCs maintained bright fluorescence. All these results demonstrate that, compared to the typical LARP-synthesized CsPbBr3 PNCs, the stability of NADES-CsPbBr3 PNCs was significantly improved.
Formation mechanism of NADES-CsPbBr3 PNCs
To further understand the formation mechanism of NADES-CsPbBr3 PNCs, the role of NADESs in this method is discussed. On the one hand, NADESs were used as solvents in the LARP method. To measure the dissolving capacity of three NADESs for CsBr and PbBr2, their polarity was evaluated through the Nile red polarity scale. As a result, CsBr can directly dissolved in Thy
:
LacA of high polarity, and PbBr2 can dissolve in Thy
:
Oct of lower polarity with the help of the coordination of OA/OAm. Meanwhile, Thy
:
DecA of the lowest polarity can be used as an antisolvent, leading to the mixture becoming a supersaturated solution and precipitating crystals to form NADES-CsPbBr3 PNCs. On the other hand, NADESs were also used as surface ligands of CsPbBr3 PNCs. For the synthesis of PNCs, introducing some organic molecules is necessary, which can strongly interact with ions and control crystal growth kinetics to reduce the defect density.45 To verify the feasibility of using NADESs as surface ligands, the crystal growth kinetics and 1H NMR characterization of NADES-CsPbBr3 PNCs were performed. It can be seen from Fig. S6,† that the fluorescence of NADES-CsPbBr3 PNCs changed from blue to green and the intensity gradually increased with the reaction time and reached the highest value at 5 h. The main reason for the difference from the rapid crystallization of typical synthetic PNCs is that the –COOH and –OH groups of NADESs can strongly coordinate with the Pb of CsPbBr3 PNCs. From the 1H NMR spectra of NADES-CsPbBr3 PNCs OA and OAm (Fig. 7), it can be found that there is no carbon–carbon double bond characteristic hydrogen peak (δ = 5.37) of OA and OAm in NADES-CsPbBr3 PNCs, which indicates that not oleylamine oleate but NADESs can act as surface ligands to effectively passivate PNCs.
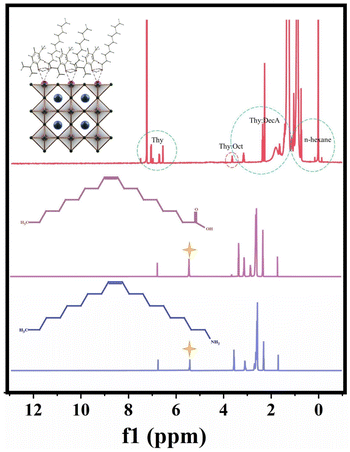 |
| Fig. 7
1H NMR spectra of NADES-CsPbBr3 PNCs, OA and OAm. | |
Light-emitting devices
Finally, the obtained NADES-CsPbBr3 NCs with a bright pure green emission were applied for fabricating a WLED lamp based on a commercially available 460 nm GaN LED blue chip (the inset in Fig. 8a). Fig. 8a shows the typical electroluminescence (EL) spectra of the WLED at a driving current of 20 mA. To further investigate the potential applications of NADES-CsPbBr3 NCs, we measured the luminous efficiency of the WLED driven by different currents, as shown in Fig. S7.† The highest power efficiency reached 55.61 Lm W−1 under a 20 mA current. The color coordinates of the sample were labeled as a red dot with a corresponding color coordinate of (0.3164, 0.3242) in the Commission International de l'Eclairage (CIE) chromaticity diagram (Fig. 8b). Therefore, the obtained NADES-CsPbBr3 PNCs reveal their potential commercial application in the light field.
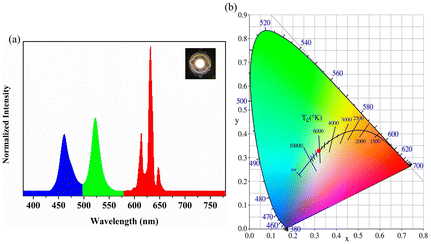 |
| Fig. 8 (a) PL emission spectrum. The inset shows the photograph of device in open states; (b) CIE coordinates of NADES-CsPbBr3 PNCs in CIE space. | |
Conclusions
In summary, the work focuses on designing a toxic organic solvent-free approach to the synthesis of CsPbBr3 PNCs based on NADESs, for meeting the environment-friendly requirements to achieve the preparation of high-performance LED chips. The as-prepared NADES-CsPbBr3 PNCs exhibit a high PLQY of ∼96.8%, a narrow FWHM of ∼18.8 nm and excellent stability. NADESs can not only use function as solvents, but also serve as a surface ligand to effectively reduce defects for the green synthesis of highly stable, bright-luminous NADES-CsPbBr3 PNCs. Besides, a WLED lamp was fabricated to verify its practical application. Compared to the traditional LARP method, our method is simple and eco-friendly, which is desirable for the large-scale production of perovskite, and opens a new synthesis pathway to green exploitation of perovskite materials.
Author contributions
Heng Lu: conceptualization, methodology, investigation, writing – original draft, formal analysis. Xiaohong Tan: methodology and resources. Guobin Huang: data curation, writing – review and editing. Shaoru Wu: investigation. Yanmei Zhou: visualization and supervision. Junying Zhang: investigation. Qiaowen Zheng: investigation and editing. Tianju Chen: visualization. Feiming Li: editing. Zhixiong Cai: editing. Jingbin Zeng: methodology, investigation and editing. Maosheng Zhang: supervision, project administration, funding acquisition.
Conflicts of interest
The authors declare that they have no known competing financial interests or personal relationships that could have appeared to influence the work reported in this paper.
Acknowledgements
This work was supported by the National Natural Science Foundation of China (nos 21904055 and 22004055), Natural Science Foundation of Fujian Province, China (nos 2021H6033, 2020J05165 and 2020J05164), Science and Technology Projects of the Education Department, Fujian Province of China (no. JK2017032), and the Natural Science Foundation of Zhangzhou City, China (no. ZZ2019J02).
References
- G. Huang, Y. Zhou, F. Li, X. Tan, Z. Cai, D. Luo, T. Chen and M. Zhang, Sens. Actuators, B, 2021, 347, 130618 CrossRef CAS.
- J. Song, T. Fang, J. Li, L. Xu, F. Zhang, B. Han, Q. Shan and H. Zeng, Adv. Mater., 2018, 30, 1805409 CrossRef PubMed.
- G. Xing, N. Mathews, S. S. Lim, N. Yantara, X. Liu, D. Sabba, M. Grätzel, S. Mhaisalkar and T. C. Sum, Nat. Mater., 2014, 13, 476–480 CrossRef CAS PubMed.
- Y. Zhou, S. Wu, G. Huang, J. Zeng, B. E. Meteku, X. Tan, H. Lu, F. Li, Z. Cai, X. Wang and M. Zhang, J. Alloys Compd., 2022, 918, 165565 CrossRef CAS.
- K. Lin, J. Xing, L. N. Quan, F. P. G. de Arquer, X. Gong, J. Lu, L. Xie, W. Zhao, D. Zhang, C. Yan, W. Li, X. Liu, Y. Lu, J. Kirman, E. H. Sargent, Q. Xiong and Z. Wei, Nature, 2018, 562, 245–248 CrossRef CAS PubMed.
- J. Ali, Y. Li, P. Gao, T. Hao, J. Song, Q. Zhang, L. Zhu, J. Wang, W. Feng, H. Hu and F. Liu, Nanoscale, 2020, 12, 5719–5745 RSC.
- K. Fu, C. T. Nelson, M. C. Scott, A. Minor, N. Mathews and L. H. Wong, Nanoscale, 2016, 8, 4181–4193 RSC.
- S. Ghimire and C. Klinke, Nanoscale, 2021, 13, 12394–12422 RSC.
- L. Lei, Q. Dong, K. Gundogdu and F. So, Adv. Funct. Mater., 2021, 31, 2010144 CrossRef CAS.
- M. Palabathuni, S. Akhil, R. Singh and N. Mishra, ACS Appl. Nano Mater., 2022, 5, 10097–10117 CrossRef CAS.
- S. Akhil, V. G. V. Dutt, R. Singh and N. Mishra, J. Phys. Chem. C, 2021, 125, 22133–22141 CrossRef CAS.
- S. Akhil, V. G. V. Dutt and N. Mishra, Nanoscale Adv., 2021, 3, 2547–2553 RSC.
- L. Protesescu, S. Yakunin, M. I. Bodnarchuk, F. Krieg, R. Caputo, C. H. Hendon, R. X. Yang, A. Walsh and M. V. Kovalenko, Nano Lett., 2015, 15, 3692–3696 CrossRef CAS PubMed.
- X. Li, Y. Wu, S. Zhang, B. Cai, Y. Gu, J. Song and H. Zeng, Adv. Funct. Mater., 2016, 26, 2435–2445 CrossRef CAS.
- F. Zhang, H. Zhong, C. Chen, X.-G. Wu, X. Hu, H. Huang, J. Han, B. Zou and Y. Dong, ACS Nano, 2015, 9, 4533–4542 CrossRef CAS PubMed.
- W. Li, W. Deng, X. Fan, F. Chun, M. Xie, C. Luo, S. Yang, H. Osman, C. Liu, X. Zheng and W. Yang, Ceram. Int., 2018, 44, 18123–18128 CrossRef CAS.
- T. Chen, Y. Xu, Z. Xie, W. Jiang, L. Wang and W. Jiang, Nanoscale, 2020, 12, 9569–9580 RSC.
- H. Luo, Y. Huang, H. Liu, B. Zhang and J. Song, Chem. Eng. J., 2022, 430, 132790 CrossRef CAS.
- M. T. Clough, K. Geyer, P. A. Hunt, S. Son, U. Vagt and T. Welton, Green Chem., 2015, 17, 231–243 RSC.
- L. Yang, H. Wang, Y.-G. Zu, C. Zhao, L. Zhang, X. Chen and Z. Zhang, Chem. Eng. J., 2011, 172, 705–712 CrossRef CAS.
- Y. Tan, Y. Zou, L. Wu, Q. Huang, D. Yang, M. Chen, M. Ban, C. Wu, T. Wu, S. Bai, T. Song, Q. Zhang and B. Sun, ACS Appl. Mater. Interfaces, 2018, 10, 3784–3792 CrossRef CAS PubMed.
- S. Akhil, V. G. V. Dutt and N. Mishra, Nanoscale, 2021, 13, 13142–13151 RSC.
- S. Akhil, V. G. V. Dutt, R. Singh and N. Mishra, J. Phys. Chem. C, 2022, 126, 10742–10751 CrossRef CAS.
- S. Akhil, V. G. V. Dutt and N. Mishra, ChemNanoMat, 2021, 7, 342–353 CrossRef CAS.
- S. Akhil, V. G. V. Dutt and N. Mishra, Chem. – Eur. J., 2020, 26, 17195–17202 CrossRef CAS PubMed.
- A. P. Abbott, G. Capper, D. L. Davies, R. K. Rasheed and V. Tambyrajah, Chem. Commun., 2003, 1, 70–71 RSC.
- Y. Fan, H. Wu, D. Cai, T. Yang and L. Yang, Sep. Purif. Technol., 2020, 250, 117211 CrossRef CAS.
- A. P. Abbott, D. Boothby, G. Capper, D. L. Davies and R. K. Rasheed, J. Am. Chem. Soc., 2004, 126, 9142–9147 CrossRef CAS PubMed.
- A. Paiva, R. Craveiro, I. Aroso, M. Martins, R. L. Reis and A. R. C. Duarte, ACS Sustainable Chem. Eng., 2014, 2, 1063–1071 CrossRef CAS.
- Y. Dai, J. van Spronsen, G. J. Witkamp, R. Verpoorte and Y. H. Choi, Anal. Chim. Acta, 2013, 766, 61–68 CrossRef CAS PubMed.
- Y. Ma, Y. Li, S. Ali, P. Li, W. Zhang, M. C. R. Rauch, S. J. P. Willot, D. Ribitsch, Y. H. Choi, M. Alcalde, F. Hollmann and Y. Wang, ChemCatChem, 2020, 12, 989–994 CrossRef CAS.
- T.-X. Yang, L.-Q. Zhao, J. Wang, G.-L. Song, H.-M. Liu, H. Cheng and Z. Yang, ACS Sustainable Chem. Eng., 2017, 5, 5713–5722 CrossRef CAS.
- W. Liu, K. Zhang, J. Chen and J. Yu, J. Mol. Liq., 2018, 260, 173–179 CrossRef CAS.
- Á. Santana-Mayor, A. V. Herrera-Herrera, R. Rodríguez-Ramos, B. Socas-Rodríguez and M. Á. Rodríguez-Delgado, ACS Sustainable Chem. Eng., 2021, 9, 2161–2170 CrossRef.
- D. Fuchs, B. C. Bayer, T. Gupta, G. L. Szabo, R. A. Wilhelm, D. Eder, J. C. Meyer, S. Steiner and B. Gollas, ACS Appl. Mater. Interfaces, 2020, 12, 40937–40948 CrossRef CAS PubMed.
- F. J. V. Gomez, M. Espino, M. de los Angeles Fernandez, J. Raba and M. F. Silva, Anal. Chim. Acta, 2016, 936, 91–96 CrossRef CAS PubMed.
- Q. Hu, H. Yu, S. Gong, Q. Han and W. Wu, J. Mater. Chem. C, 2022, 10, 6002–6008 RSC.
- M. Wang, F. Qiao and H. Yan, Green Chem., 2021, 23, 5179–5188 RSC.
- J. Yue, Z. Zhu, J. Yi, H. Li, B. Chen and J. Rao, Food Chem., 2022, 376, 131943 CrossRef CAS PubMed.
- K. Zhu, Q. Wei, H. Li and X. Ren, ACS Sustainable Chem. Eng., 2022, 10, 2125–2135 CrossRef CAS.
- A. K. Dwamena and D. E. Raynie, J. Chem. Eng. Data, 2020, 65, 640–646 CrossRef CAS.
- M. T. Hoang, N. D. Pham, Y. Yang, V. T. Tiong, C. Zhang, K. Gui, H. Chen, J. Chang, J. Wang, D. Golberg, J. Bell and H. Wang, Green Chem., 2020, 22, 3433–3440 RSC.
- Y. Liu, D. Li, L. Zhang, Y. Chen, C. Geng, S. Shi, Z. Zhang, W. Bi and S. Xu, Chem. Mater., 2020, 32, 1904–1913 CrossRef CAS.
- Q. Zhong, M. Cao, Y. Xu, P. Li, Y. Zhang, H. Hu, D. Yang, Y. Xu, L. Wang, Y. Li, X. Zhang and Q. Zhang, Nano Lett., 2019, 19, 4151–4157 CrossRef CAS PubMed.
- Y. Liu, X. Zheng, Y. Fang, Y. Zhou, Z. Ni, X. Xiao, S. Chen and J. Huang, Nat. Commun., 2021, 12, 1686 CrossRef CAS PubMed.
|
This journal is © The Royal Society of Chemistry 2022 |