DOI:
10.1039/D2ME00066K
(Review Article)
Mol. Syst. Des. Eng., 2022,
7, 1017-1029
Digital light processing 3D printing of hydrogels: a minireview
Received
17th April 2022
, Accepted 30th June 2022
First published on 1st July 2022
Abstract
The rapid development of three-dimensional (3D) printing of hydrogel materials into sophisticated constructs has led to considerable progress in the fields of tissue engineering, soft actuators/robots, flexible electronics, etc. Notably, digital light processing (DLP) based 3D printing provides a potential strategy to achieve high resolution and elaborate 3D structures that are important for specific applications. In this review, we highlight the recent advances in the field of DLP printing of hydrogels with emphasis on the selection of materials, structure designs, and printing technologies. We then discuss the applications of DLP printed hydrogel constructs in biomedicine, tissue engineering, medical devices, soft actuators, and flexible electronics. Finally, the current challenges and future perspectives of DLP printing of hydrogels are also discussed.
Design, System, Application
This is a review paper, in which we give an overview of the recent advances in the field of digital light processing 3D printing of hydrogels with emphases on printing technologies, materials selection, structure designs, and representative applications. We do not report new materials, new designs, or new applications in this paper.
|
1. Introduction
Three-dimensional (3D) printing, also known as additive manufacturing (AM), is an emerging rapid manufacturing technology first developed in the late 1980s.1 The essence of 3D printing is the process of stacking raw materials into the corresponding solid devices according to a 3D digital model predesigned using computer modeling software.2,3 Owing to the dimensions and flexibility in endowing materials with micro- to nano-scale structural architectures and new functionalities beyond those of their bulky counterparts,4 3D printing has received growing attention and will continue to favor broad applications including biomedical devices, intelligent systems, energy devices, and soft robotics.5–8 With the continuous in-depth research on the forming mechanisms and material systems, various 3D printing technologies have been developed in recent years, including fused deposition modelling (FDM),9,10 direct ink writing (DIW),11–13 direct inkjet printing (DIP),14,15 selective laser sintering (SLS),16,17 stereolithography apparatus (SLA),18 digital light processing (DLP)13,19 and so on.20–22
Among these printing technologies, DLP belonging to light-based printing utilizes projection light to polymerize precursors to obtain pre-designed architectures.6,23 In particular, the printing speed of this technology ranges from 25 to 1000 mm min−1, and relies chiefly on the intensity of light power and the sensitivity of the printing material. The resolution of printed structures based on DLP has reached as high as 1 μm by focusing the projected light through an optical system.24 Therefore, DLP printing has attracted growing research interest due to its high printing speed, high resolution, and low cost.13,25–27 In spite of extensive progress, DLP-based 3D printing is generally limited to material choices. For example, most of DLP-compatible materials are photocurable rigid thermoset materials.5,6,28–36 It is highly required to devise flexible devices with soft and active architectures in the new era of intelligence.
Recent advances in soft materials such as hydrogels with good biocompatibility, processibility, and adaptability have opened up a broad spectrum of opportunities in diverse areas, including drug delivery, tissue engineering, and soft actuators.37–46 Combined with emerging DLP based 3D printing technologies, hydrogels have been fabricated into complex architectures such as vascular networks, porous scaffolds, and meniscus substitutes for target applications.47–50 In response to the growing interest in this area, we aim to provide a general overview for the rapid development of DLP printing of hydrogels. In this minireview, we give a simple introduction to DLP-based printing technologies, summarize recent progress in DLP-based printing of hydrogels into various constructs, and present their applications in the fields of tissue engineering, biomedical devices, soft actuators, and flexible electronics. Finally, we conclude with the discussion of current challenges and future perspectives of DLP printing of hydrogel materials.
2. DLP printing technologies and materials requirements
2.1. Printer features and operation
A DLP printer includes a projector light and build platform, a vat of liquid resin, and a mobile Z axis.5,6 The core component is the digital micromirror device (DMD) embedded in the projector which controls the resolution of projected 2D images sliced from the target 3D object and directly determines the geometric morphology and printing accuracy of the final constructs.51 Once a layer has been printed, the platform moves vertically for a certain distance and continues to locally cure the precursor by using patterned ultraviolet light. This process is repeated layer by layer until the desired 3D architecture is constructed. According to the moving direction of the building platform, DLP-based 3D printing is generally divided into the “bottom-up” form and “top-down” form.29
2.1.1. “Bottom-up” projection approach.
In “bottom-up” DLP printing, the printed object hangs upside down from the bottom of the build platform. During the printing process, solidification occurs at the bottom of the vat, after which the retractable platform pulls upward from the liquid for a certain distance. The robotically controlled focal plane moves vertically for another illumination and photocuring process (Fig. 1a). Notably, the bottom plate of the resin tank needs to be light permeable and covered with an oxygen-permeable film or perfluorinated film to reduce the adhesion between the printed structure and the printer. For the “bottom-up” projection approach, the prominent advantages are less resin consumption, higher leveling rate of liquid resins, and more diversified adaptability of materials.52
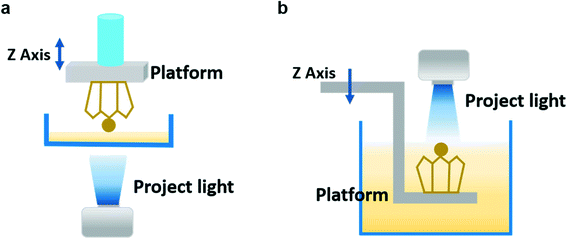 |
| Fig. 1 Schematic illustration for the “bottom-up” (a) and “top-down” (b) printing technologies based on digital light processing (DLP) of photocurable materials. | |
2.1.2. “Top-down” projection approach.
In “top-down” DLP printing, the object is printed at the air–liquid interface at the top, and the platform is moved downward into the vat vertically during the printing process (Fig. 1b). The height of the printed object, therefore, depends heavily on the vat depth. The printed object is soaked in the raw material, and the printer cures the top layer of the fluid. This type of printing requires lots of raw materials and is prone to waste.13,25 The object size obtained by this method has relatively high resolution and fidelity, because the bottom vat resin not only serves as the supply source to form a new layer for the next printing but also provides buoyant force to support the printed constructs. However, the vibration of solution at the air–liquid interface is a challenging issue that reduces the resolution in top-down projection when compared to the bottom-up approach, which can be mitigated by increasing the layer-to-layer printing interval.29
2.2. Formulation of the precursor solution
In general, processing of hydrogels by using DLP-based 3D printing involves an aqueous solution of photosensitive oligomers or reactive monomers, a photoinitiator activated above 385 nm, a photo-inhibitor, and/or a cross-linking agent.24,48,53 Many DLP precursor solutions possess a low apparent viscosity (<5 Pa s) to bring rapid and even replenishment of fresh resin into the processing area for the construction of the next layer.54 When the apparent viscosities are higher than 100 kPa s, continuous printing often fails because the partially cured resin with a high viscosity cannot be fully removed from the surface of the printed object. The repeated exposures for subsequent layers can further aggravate this issue of incomplete resin recirculation, sub-gelation polymerization, and eventual overcuring. This undesired solidification adds unwanted fillets at the edges or completely fills negative features (i.e., holes, gaps, and overhangs). Beyond the limitation of resolution, highly viscous ink also creates large adhesive or drag forces between the projection platform and printed object during bottom-up DLP. Such adhesive stresses, particularly for large cross-sectional areas, can exceed the strength of the material and cause failure of the printed constructs.55
The photoinitiator plays a crucial role in determining the rate of polymerization and, consequently, the resulting properties of the printed construct and the time required for 3D fabrication.48,56,57 According to different solubilities in water, photoinitiators are divided into water-soluble photoinitiators and oil-soluble photoinitiators. Oil-soluble photoinitiators commonly used for DLP printing are 2,4,6-trimethylbenzoyldiphenylphosphine oxide (TPO) and phenylbis(2,4,6-trimethylbenzoyl)phosphine oxide (Irgacure 819) because of high efficiency. In addition, available water-soluble photoinitiators include 2-hydroxy-1-[4-(2-hydroxyethoxy)phenyl]-2-methyl-1-propanone (I2959) and lithium phenyl-2,4,6-trimethylbenzoylphosphinate (LAP). However, it still remains a grand challenge to engineer hydrogels with high water content using DLP printing technology because of the absence of more efficient water-soluble photoinitiators to enable fast gelation and continuous printing of the precursors.58 In order to alleviate the problem, Pawar et al. successfully presented an innovative approach to enable rapid DLP printing of hydrogel precursor solutions by converting the water-insoluble photoinitiator TPO into water-dispersible nanoparticles,59 which exhibited a high efficiency and high speed of polymerization upon irradiation.60 The reaction rate also depends on the light intensity of the projector. In other words, the processing speed of the precursors is related to the intensity of light and the efficiency of photoinitiators. For practical applications, the selection of photoinitiators should also consider the stability of the whole system, which should be ensured after the addition of photoinitiators. The photoinitiators used for DLP printing, as well as the light initiation wavelength and corresponding curing speed, are summarized in Table 1.
Table 1 Photoinitiators commonly used for DLP printing of hydrogels
Name |
Molecular structure |
Solubility |
Initiation wavelength (nm) |
Ref. |
I2959 |
|
Water-soluble |
365–405 |
47
|
LAP |
|
Water-soluble |
365–405 |
61
|
Water-soluble TPO |
|
Water-soluble |
365–405 |
47
|
TPO |
|
Oil-soluble |
365–405 |
62
|
Irgacure 819 |
|
Oil-soluble |
365–405 |
63
|
Furthermore, photoinitiators and photo-absorbers are particularly important for DLP-based 3D printing. The former determines the time required to initiate the polymerization and/or crosslinking reaction and the cross-linking degree, while the latter is used to control the curing depth of the photopolymer resin and prevent ultraviolet light from penetrating the entire layer.64 Photo-inhibitors contribute to the smooth surface of printed building blocks, avoiding the occurrence of excessive cured hydrogel in preset sections. Otherwise, hydrogels normally undergo excessive curing in the absence of a light absorber and displays a poor printing ability.65 For a better comparison of the influencing factors on the DLP printing of hydrogel materials, the material composition, printing method, light initiation wavelength, curing speed, mechanical properties, resolution, and relevant applications of printed constructs are summarized in Table 2.
Table 2 Various DLP printed hydrogels, including the material system, photoinitiator, photo-absorber, printing approach, initiation wavelength, curing time, Young's modulus, resolution, and relevant applications
Material system |
Photoinitiator |
Photo-absorber |
Printing method |
Initiation wavelength (nm) |
Curing speed |
Young's modulus (kPa) |
Resolution (μm) |
Applications |
Ref. |
Note: PEGDA, GelMA, AAm, ACMO, PVA, and AAc are the abbreviations of poly(ethylene glycol) diacrylate, gelatin methacrylate, acrylamide, 4-acryloylmorpholine, polyvinyl alcohol, and acrylic acid, respectively.
|
PEGDA/GelMA |
LAP |
Brilliant blue |
Bottom-up |
365 |
4.9 s/50 μm |
∼500 |
10 |
Cell culture |
66
|
AAm |
Water-soluble TPO |
Sudan I/food dye |
Top-down |
405 |
7.5–32 s/140 μm |
7–260 |
7 |
Stretchable electronics |
67
|
ACMO/PEGDA/AAc |
Oil-soluble TPO |
Sudan III |
Top-down |
405 |
8 s/100 μm |
— |
500 |
Self-weldability |
62
|
AAm/AAc |
Water-soluble TPO |
Tartrazine |
Bottom -up |
— |
2.4 s/50 μm |
42 |
100 |
Tissue engineering |
68
|
PVA/AAc/PEGDA |
Water-soluble TPO |
Methyl red and brilliant green |
Bottom-up |
385 |
5 s/200 μm |
∼60 |
1000 |
— |
53
|
AAc |
Irgacure 819 |
Sudan I |
Top-down |
385 |
3–10 s/100 μm |
— |
— |
— |
69
|
AAm/AAc |
Irgacure 819 |
Sudan I |
Bottom-up |
405 |
3 s/100 μm |
∼110 |
— |
Sensor |
48
|
3. DLP 3D printing of hydrogels and their applications
Elaborate architectures of hydrogel materials can be printed by selecting suitable raw materials and adjusting the printing parameters, which greatly broadens the application of hydrogels in diverse fields. In this section, 3D hydrogel constructs fabricated by DLP printing are reviewed with their applications in tissue engineering, biomedical devices, soft actuators/robots, and flexible electronics.
3.1. Tissue engineering and biomedical devices
Hydrogels are an ideal candidate for tissue engineering and biomedical devices due to their special characteristics such as flexibility, biocompatibility, and sensitivity to physiological environments.70–73 DLP-based 3D printing offers a fascinating manufacturing method to fabricate sophisticated constructs with self-defined microstructures, which can greatly widen the application of hydrogels or their hybrids in these areas. For example, Yang and coworkers have fabricated a heterogeneous structure with the shape of a human heart valve derived from computed tomography (CT) data.68 This hybrid hydrogel construct consisted of a hydrogel skeleton produced by DLP-based 3D printing with the “bottom-up” projection approach and another hydrogel matrix prepared by casting (Fig. 2a). The artificial heart valve exhibited excellent fatigue resistance during cyclic experiments, much better than that of comparative homogeneous hydrogel constructs. This work will inspire the creation of artificial organs with tailored mechanical performances and good biocompatibility based on the DLP printing technology. In addition, Zhang et al. printed high-resolution and high-fidelity stretchable hydrogel tissues such as the ear, nose, and blood vessels by using the DLP-based 3D printing technology.67 The raw materials used for printing included acrylamide, poly(ethylene glycol) diacrylate, and a self-made water-soluble photoinitiator, which showed excellent biocompatibility. In addition, Xue et al. printed cell-seeding hydrogel scaffolds by DLP-based 3D printing of precursors containing poly(ethylene glycol) diacrylate (PEGDA) and a home-made photoinitiator, which exhibited regionally varied stiffness by adjusting the exposure time (Fig. 2b).24 3T3 fibroblasts were evenly distributed on the printed scaffold and proliferated well during cell culture, indicating the excellent biocompatibility and physical support of the hydrogel scaffolds. The approach provides a solution to constructing heterogeneous cell-seeding hydrogel scaffolds with different regional stiffness by changing the exposure time to adjust the mechanical properties. Moreover, Wang et al. have developed complex hydrogel constructs at a high resolution by using a gradient DLP 3D printing system with a combination of a digital micromirror device-based bioprinter and a microfluidic chaotic mixer-linked vat (Fig. 2c).74 The approach can achieve functional (bio)printing by controlling the (bio)ink gradients with various cell densities, substrate stiffness, and growth factor concentration, as well as porosity and pore size, and endow the recapitulation of the gradient properties of natural tissues with complex architectures and at high resolution. Furthermore, Ge et al. printed a cardiovascular shape memory polymer stent with a self-built multi-material DLP printer by integrating a hydrogel inside it, which can be used to load drugs at the designated hydrogel sites.75 The working principle of the printed stent was demonstrated using artificial blood vessels (Fig. 2d). The stent contracted prior to use and expanded gradually after being held at the desired site accompanied by drug release. The release rate of loaded drugs can reach 90% within 3 hours. This work provides a new way to realize the fabrication of soft devices with multiple materials by bonding hydrogels with other polymers in 3D architectures.
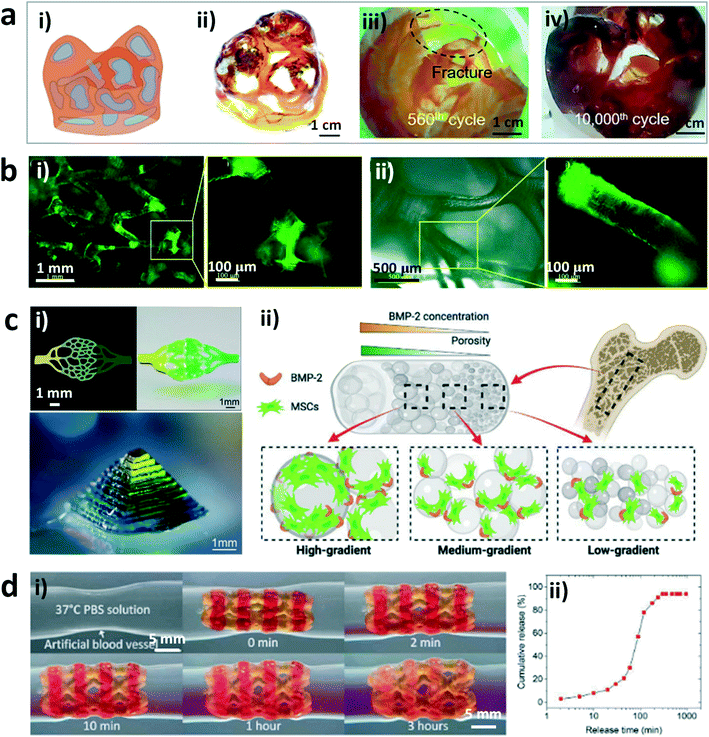 |
| Fig. 2 DLP printed hydrogels and their applications in tissue engineering and biomedical devices. (a) Schematic (i) and image (ii) of the heterogeneous hydrogel, and endoscopy images of the homogeneous (iii) and heterogeneous (iv) hydrogel heart valves after cyclic experiments.68 Reprinted from ref. 68, copyright 2021, Elsevier. (b) Fluorescence microscopy images of the 3T3 fibroblast distribution in the printed hydrogel scaffold (i) and interaction of 3T3 fibroblasts with the joint of the scaffold (ii).24 Reprinted from ref. 24, copyright 2019, American Chemical Society. (c) 2D capillary-like network structure and 3D pyramid structure printed by the gradient DLP printing technique (i) and a schematic to show the printed 3D object encapsulated with MSCs for the investigation of osteogenic effects of the dual gradients of porosity and BMP-2 (ii).74 Reprinted from ref. 74, copyright 2022, Wiley-VCH. (d) Illustrations of drug release from the printed stent in the artificial blood vessel (i) and the quantification of drug release from the printed stent (ii).47 Reprinted from ref. 47, copyright 2021, American Association for the Advancement of Science. | |
3.2. DLP 4D printing of hydrogels
4D printing is an emerging technology, in which the 3D-printed structure can self-transform in shape, properties, and functions in response to stimuli.69,76,77 Inspired by the biological deformations of natural systems, abundant bionic morphing hydrogels have been developed by creating heterogeneous network structures and/or imparting inhomogeneous stimulations.78–81 Recently, there have been several programmed shape-morphing systems developed by using DLP-based 3D printing technologies to fabricate hydrogels or hydrogel–polymer hybrids. For example, Ji et al. explored a one-step method to fabricate complex 3D architectures that can deform under stimuli from humidity and temperature.82 These hydrogel constructs were developed by using a commercial DLP printer with the “bottom-up” projection approach. The principle of shape morphing was related to the asymmetric swelling of the printed hydrogel blocks with programmed secondary microstructures on one surface. The printed architectures showed bending and twisting deformations induced by moisture, and returned to their original shapes by adjusting the environment conditions (Fig. 3a). In particular, various 3D desired morphologies including triangular pyramid, stereo windmill, “octopus”, and hydroturbine shapes can be obtained by taking advantage of the flexible design and freeform fabrication of this method (Fig. 3b). In addition, Zhao et al. printed self-folding origami constructs with environmental responsiveness by DLP printing of a PEGDA based material system.83 As shown in Fig. 3c, different cross-linking degrees existed in the as-printed architecture by controlling the light intensity during the printing, which resulted in self-folding deformation when desolvation in water occurred. Notably, the folded origami recovered its original shape after being incubated in the swelling medium. Moreover, Wu et al. achieved distinct circumferential buckling, visible during the growth of pumpkins, which was reproduced by a core–shell barrel structure developed by 4D printing of responsive hydrogels (Fig. 3d).69 The deformation process was triggered by swelling of the hydrogels. Various morphological transformations of biomimetic structures were inspired by real cabbage flowers/leaves, chrysanthemum, leaves of rose, and helix strips of seedpods. These studies have paved the way to fabricating hydrogels with heterogeneous structures, which should broaden the applications of hydrogel materials in bionics and engineering fields.
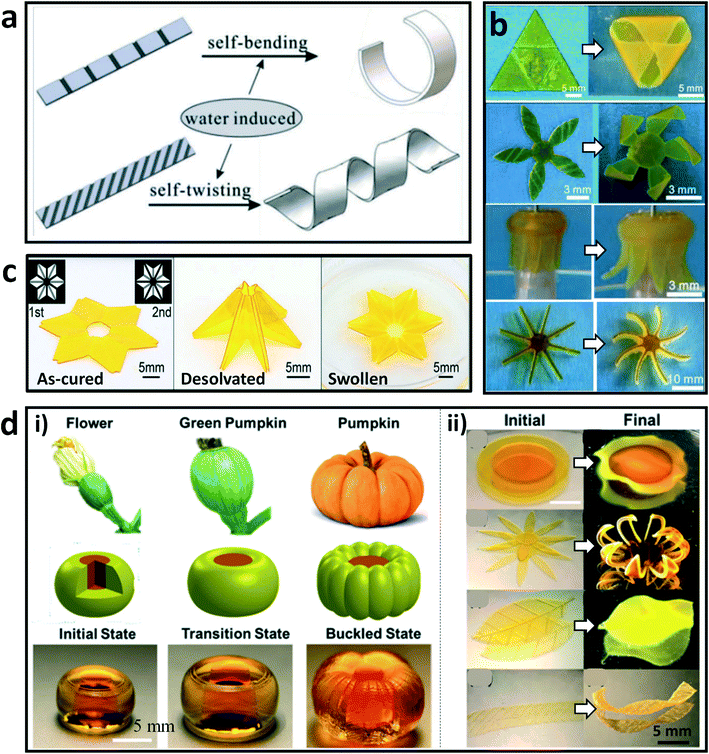 |
| Fig. 3 DLP-based 4D printing of hydrogels. (a) Illustration of the bending and twisting deformations of printed strips with programmed structures. (b) Complex shape morphing of printed constructs triggered by water.82 Reprinted from ref. 82, copyright 2019, Wiley-VCH. (c) As-cured flat pattern, desolvated origami shape, and swollen flat shape of the printed six-petal flower with the origami structure. Insets show two grayscale patterns for DLP printing.83 Reprinted from ref. 83, copyright 2017, Wiley-VCH. (d) Images, schematic diagram, and photos of the printed hydrogel (i) that deformed into a pumpkin-like morphology during the swelling process; morphological transformations (ii) of printed biomimetic structures from the initial plain states to their final states after the swelling process.69 Reprinted from ref. 69, copyright 2019, American Chemical Society. | |
3.3. DLP printed hydrogels as soft actuators
As one class of intelligent devices, soft actuators and robots can transform external stimuli into programmed deformations and mechanical motions.84–87 By using DLP-based 3D printing technologies, a variety of hydrogel actuators and robots have been developed with responsive elements and/or asymmetrical geometries in the constructed blocks.88 For example, an eight-arm gripper was printed by incorporating the responsive component with a lower critical solution temperature (LCST) to the system, and showed asymmetric swelling behavior in response to variation of temperature. The printed gripper can be used to transport objects quickly by controlling the environment temperatures (Fig. 4a).82 In addition, Hua et al. have devised a bilayer hydrogel actuator with high toughness and actuation force by DLP-based 3D printing with the raw materials N-isopropylacrylamide (NIPAm) and poly(vinyl alcohol) (PVA) or its methacrylate derivatives (PVA-MA).61 The passive layer was composed of PVA/PVA-MA, while the active layer was composed of PVA/PVA-MA-g-PNIPAm with temperature responsiveness. In a hot water bath, the actuator can grasp an object multiple times its own weight. The bilayer actuator was further coated with polypyrrole to enable remote and selective activation by using an infrared laser (Fig. 4b), which makes the actuator easier to use in practical applications.
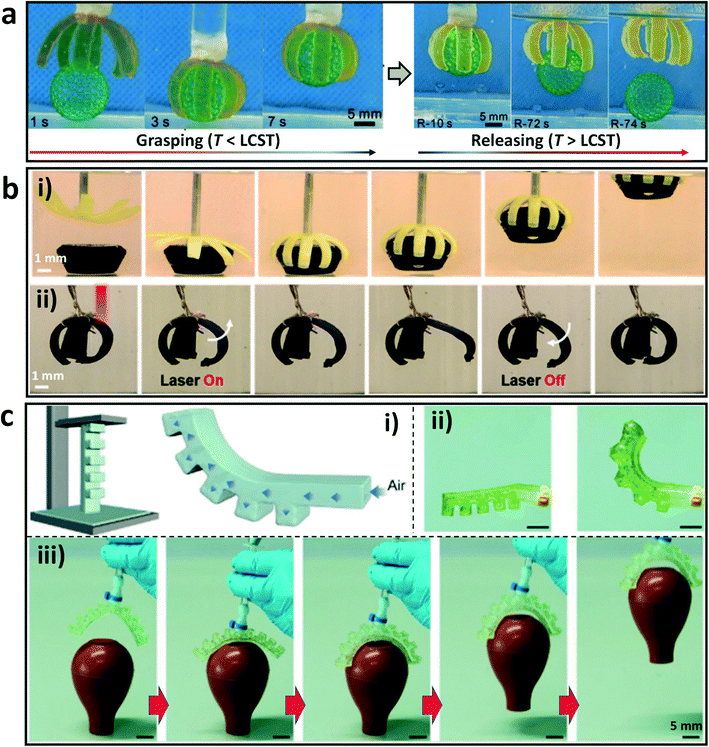 |
| Fig. 4 DLP printed hydrogels as soft actuators. (a) 3D printed thermal-responsive hydrogel actuator for object transportation.82 Reprinted from ref. 82, copyright 2019, Wiley-VCH. (b) Grasping of an object with a 3D printed bilayer hydrogel gripper through thermal actuation (i), and infrared laser-mediated remote and selective actuation of the bilayer actuator coated with polypyrrole (ii).61 Reprinted from ref. 61, copyright 2021, American Chemical Society. (c) Schematic diagram of the soft pneumatic actuator and the deformation after pressurization (i); photos of the one-arm actuator before and after pressurization (ii); photos of the printed two-arm hydrogel actuator to capture a soft rubber pipette bulb (iii).89 Reprinted from ref. 89, copyright 2022, Wiley-VCH. | |
Moreover, Wang et al. developed a special polymer gel (PEGgel) system by polymerizing hydroxyethyl methacrylate and acrylic acid in poly(ethylene glycol) (PEG) medium.89 A pneumatic soft actuator with an asymmetric hollow structure was printed by DLP 3D printing with the PEG gel precursor (Fig. 4c). When pressurized, the serrated portion expanded and the printed gel deformed toward the non-serrated side, resulting in a directional force that acted as an actuator. A two-arm actuator was further fabricated, which can realize the capture of a rubber pipette bulb successfully. This work avoids the complex and multi-step molding or casting manufacturing processes for the fabrication of soft actuators, and enables geometric complexity and functionality for the design of soft robots.
3.4. DLP printed hydrogels as soft electronics
The network structure of hydrogels is conducive to the rapid movement of ions and endows them good conductivity, which gives the application possibility of conductive hydrogels in soft electronics.90–92 However, conventional fabrication methods such as molding lead to simple structures and insufficient dimensional accuracy of constructs, which are crucial for the reliability and functionality of hydrogel devices.93,94 The DLP-based 3D printing technology can provide a solution to address this conundrum. For example, He and coworkers prepared composite hydrogels through DLP 3D printing with a synthesized microemulsion and common hydrophobic photoinitiator.48 The composite hydrogel possessed high mechanical strength (22.9 MPa) and ionic conductivity (9.64 S m−1). Pressure sensors with micrometer-scale pyramids were printed, and showed a high absolute value of pressure sensitivity of 0.103 kPa−1 below 1.25 kPa. Compared to a planar hydrogel sensor with the same material, the printed three-layer pyramid sensor had a higher sensitivity due to the larger deformation under the same pressure. On account of the excellent sensitivity, the obtained hydrogel sensors were used to monitor real-time large and subtle human motions (Fig. 5a). In addition, Wang et al. synthesized a water-soluble photoinitiator through a simple and straightforward one-pot method.56 They printed a pressure sensor with a structure of regularly arranged hollow pentagonal prisms by DLP-based 3D printing. The obtained sensor showed fast responsiveness and excellent durability under cyclic loading–unloading due to its special geometry structure (Fig. 5b). These special geometry structures can increase the sensitivities and stabilities of these strain sensors by increasing the deformation capacity under external force, which is informative for the design of high-performance sensors.
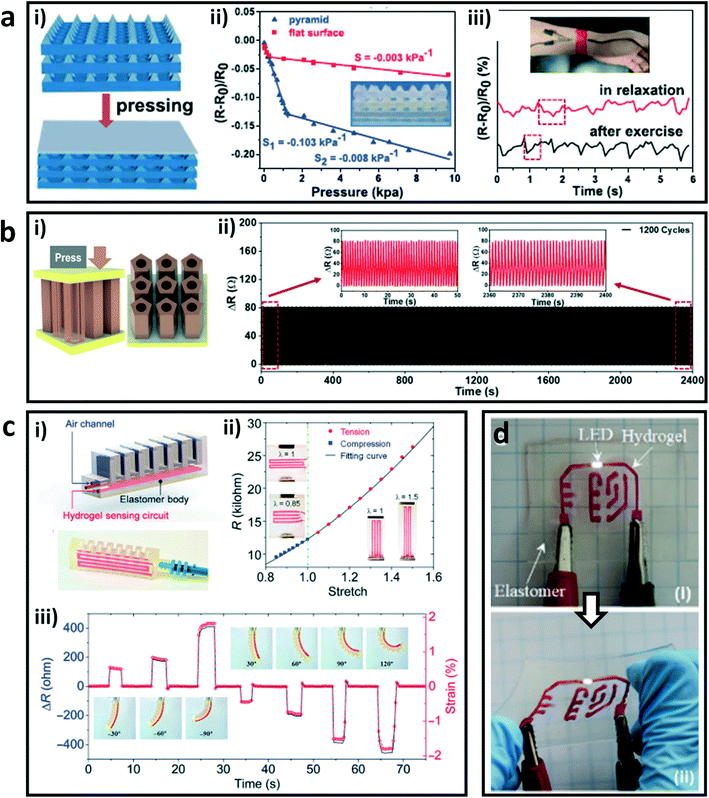 |
| Fig. 5 DLP printed hydrogels and their hybrids as soft electronics. (a) Schematic illustration of the printed three-layer pressure sensors with micrometer-scale pyramids (i); the pressure sensitivities of the printed hydrogel sensor and the planar sensor (ii); usage of the printed sensor to monitor the pulse at the wrist of a volunteer in the relaxation state and after exercise (iii).48 Reprinted from ref. 48, copyright 2021, American Chemical Society. (b) Printed pressure-sensitive sensor with the structure of regularly arranged hollow pentagonal prisms (i) and the change of its resistance in a cyclic loading process (ii).56 Reprinted from ref. 56, copyright 2021, American Chemical Society. (c) Schematic and illustration of the printed soft pneumatic actuator (SPA) (i); the relationship between the resistance and stretching (ii) and the sensing performance of the printed hydrogel SPA to bending deformations (iii).47 Reprinted from ref. 47, copyright 2021, American Association for the Advancement of Science. (d) An electronic panel achieved by printing an ionic hydrogel circuit on an elastomeric substrate in initial state (i) and stretched state (ii).67 Reprinted from ref. 67, copyright 2018, Royal Society of Chemistry. | |
Besides, Ge and coworkers printed a soft pneumatic actuator (SPA) with a strain sensor by using a self-built DLP-based 3D printer with multiple hydrogel and elastomer materials.47 The relationships between resistance and tension and compression of the composite sensor were constructed by longitudinal and transverse tensile respectively, which exhibited nonlinear resistance–deformation behaviors. The constructed strain sensor can detect the actuations of the SPA with both positive and negative bending by transforming the measured resistance variations to the strains of the printed SPA (Fig. 5c). Moreover, they also printed a flexible electronic board with an ion conductive hydrogel circuit on an elastomer sheet with high resolution and high fidelity, which maintained electrical functionality under large deformation due to the strong interface bonding between different materials (Fig. 5d).67 These studies greatly broadened the functionality and performance of hydrogel–polymer hybrid devices and machines.
4. Conclusions and perspectives
Among all the additive manufacturing technologies, DLP-based 3D printing exhibits many advantages such as high printing speed and high resolution, and thereby has been recognized as an ideal fabrication strategy to develop sophisticated architectures with applications in tissue engineering, soft actuators, and flexible electronics.95–100 This review summarizes recent progress in relevant fields with the focus on DLP printing of hydrogel materials.
In recent years, hydrogels have been developed rapidly and widely used in diverse fields.101–106 However, hydrogel systems suitable for DLP printing are still very limited because of the lack of high-efficiency water-soluble photoinitiators. In addition, most of photocurable hydrogels have the problems of cumbersome polymerization rate and inferior solidification capacity, leading to fundamental shortcomings such as a low Young's modulus of the printed hydrogels. It remains a grand challenge to achieve high-resolution printing of tough hydrogels.47,67 It is highly desired to expand hydrogel systems suitable for DLP printing in terms of developing high-efficiency water-soluble photoinitiators and designing tough hydrogels with unique network structures and energy dissipation mechanisms.101–104 Besides, DLP-based multi-material 3D printing is another way to expand the application of hydrogels, in which the problem of interface bonding between different materials should be well addressed. In this way, materials with different functions can be integrated to afford versatile printed structures.107–109
DLP printing has the advantages of high printing accuracy and smooth product surface, and it is mainly used for manufacturing small volume items such as jewelry casts and dental molds. This is because the light source in the printing process is flat exposure, and the exposure area is limited. Due to the limited resolution of the digital light mirror (DMD) of the printing equipment, it is still challenging to print large sized products. Therefore, future efforts should be devoted to improving the resolution of DMDs by enhancing the number of microscopes inside the DMD chips for the fabrication of large-sized products.
As described above, the DLP printing technology has enabled the engineering hydrogels with sophisticated architectures and/or heterogeneous structures in a high-efficiency way, which greatly improves the applications of gel materials in the biomedical and engineering fields. We believe that this review will broaden research interest and inspire innovative ideas for the fabrication and application of hydrogel constructs. The DLP-based 3D printing technologies will provide stronger power for people from all walks of life in the future.
Conflicts of interest
There are no conflicts to declare.
Acknowledgements
This work was supported by the National Natural Science Foundation of China (51973189, 52173012) and the Excellent Youth Foundation of Zhejiang Province of China (LR19E030002).
References
- R. L. Truby and J. A. Lewis, Printing soft matter in three dimensions, Nature, 2016, 540(7633), 371–378 CrossRef CAS PubMed.
- A. Ambrosi and M. Pumera, 3D-printing technologies for electrochemical applications, Chem. Soc. Rev., 2016, 45(10), 2740–2755 RSC.
- W. T. Nugroho, Y. Dong and A. Pramanik,
et al., Smart polyurethane composites for 3D or 4D printing: General-purpose use, sustainability and shape memory effect, Composites, Part B, 2021, 223, 109104 CrossRef CAS.
- E. MacDonald and R. Wicker, Multiprocess 3D printing for increasing component functionality, Science, 2016, 353(6307), aaf2093 CrossRef CAS PubMed.
- G. Ge, Q. Wang and Y. Z. Zhang,
et al., 3D printing of hydrogels for stretchable ionotronic devices, Adv. Funct. Mater., 2021, 31(52), 2107437 CrossRef CAS.
- T. J. Wallin, J. Pikul and R. F. Shepherd, 3D printing of soft robotic systems, Nat. Rev. Mater., 2018, 3(6), 84–100 CrossRef.
- J. Li and M. Pumera, 3D printing of functional microrobots, Chem. Soc. Rev., 2021, 50(4), 2794–2838 RSC.
- F. Zhu, J. Lin and Z. L. Wu,
et al., Tough and conductive hybrid hydrogels enabling facile patterning, ACS Appl. Mater. Interfaces, 2018, 10(16), 13685–13692 CrossRef CAS PubMed.
- S. C. Daminabo, S. Goel and S. A. Grammatikos,
et al., Fused deposition modeling-based additive manufacturing (3D printing): techniques for polymer material systems, Mater. Today Chem., 2020, 16, 100248 CrossRef CAS.
- M. A. Caminero, J. M. Chacón and I. García-Moreno,
et al., Impact damage resistance of 3D printed continuous fibre reinforced thermoplastic composites using fused deposition modelling, Composites, Part B, 2018, 148, 93–103 CrossRef CAS.
- S. Y. Zheng, Y. Shen and F. Zhu,
et al., Programmed deformations of 3D-printed tough physical hydrogels with high response speed and large output force, Adv. Funct. Mater., 2018, 28(37), 1803366 CrossRef.
- C. Du, J. Hu and X. Wu,
et al., 3D printing of a tough double-network hydrogel and its use as a scaffold to construct a tissue-like hydrogel composite, J. Mater. Chem. B, 2022, 10(3), 468–476 RSC.
- S. H. Kim, Y. K. Yeon and J. M. Lee,
et al., Precisely printable and biocompatible silk fibroin bioink for digital light processing 3D printing, Nat. Commun., 2018, 9(1), 1620 CrossRef PubMed.
- B. Li, N. Hu and Y. Su,
et al., Direct inkjet printing of aqueous inks to flexible all-solid-state graphene hybrid micro-supercapacitors, ACS Appl. Mater. Interfaces, 2019, 11(49), 46044–46053 CrossRef CAS PubMed.
- Z. Zhu, H. Ning and W. Cai,
et al., Morphology modulation of direct inkjet printing by incorporating polymers and surfactants into a sol-gel ink system, Langmuir, 2018, 34(22), 6413–6419 CrossRef CAS PubMed.
- F. Sillani, R. G. Kleijnen and M. Vetterli,
et al., Selective laser sintering and multi jet fusion: Process-induced modification of the raw materials and analyses of parts performance, Addit. Manuf., 2019, 27, 32–41 CAS.
- R. Ajdary, N. Kretzschmar and H. Baniasadi,
et al., Selective laser sintering of lignin-based composites, ACS Sustainable Chem. Eng., 2021, 9(7), 2727–2735 CrossRef CAS.
- B. Y. Yu, K. Son and K. B. Lee, Evaluation of intaglio surface trueness and margin quality of interim crowns in accordance with the build angle of stereolithography apparatus 3-dimensional printing, J. Prosthet. Dent., 2021, 126(2), 231–237 CrossRef CAS PubMed.
- D. K. Patel, A. H. Sakhaei and M. Layani,
et al., Highly stretchable and UV curable elastomers for digital light processing based 3D printing, Adv. Mater., 2017, 29(15), 1606000 CrossRef PubMed.
- J. R. Tumbleston, D. Shirvanyants and N. Ermoshkin,
et al., Continuous liquid interface production of 3D objects, Science, 2015, 347(6228), 1349–1352 CrossRef CAS PubMed.
- A. J. Capel, R. P. Rimington and M. P. Lewis,
et al., 3D printing for chemical, pharmaceutical and biological
applications, Nat. Rev. Chem., 2018, 2(12), 422–436 CrossRef.
- E. Sachyani Keneth, A. Kamyshny and M. Totaro,
et al., 3D printing materials for soft robotics, Adv. Mater., 2021, 33(19), e2003387 CrossRef PubMed.
- X. Kuang, J. Wu and K. Chen,
et al., Grayscale digital light processing 3D printing for highly functionally graded materials, Sci. Adv., 2019, 5(5), eaav5790 CrossRef CAS PubMed.
- D. Xue, J. Zhang and Y. Wang,
et al., Digital light processing-based 3D printing of cell-seeding hydrogel scaffolds with regionally varied stiffness, ACS Biomater. Sci. Eng., 2019, 5(9), 4825–4833 CrossRef CAS PubMed.
- L. Y. Zhou, J. Fu and Y. He, A review of 3D printing technologies for soft polymer materials, Adv. Funct. Mater., 2020, 30(28), 2000187 CrossRef CAS.
- X. Li, R. Yu and Y. He,
et al., Self-healing polyurethane elastomers based on a disulfide bond by digital light processing 3D printing, ACS Macro Lett., 2019, 8(11), 1511–1516 CrossRef CAS PubMed.
- M. Layani, X. Wang and S. Magdassi, Novel materials for 3D printing by photopolymerization, Adv. Mater., 2018, 30(41), e1706344 CrossRef PubMed.
- B. Zhang, K. Kowsari and A. Serjouei,
et al., Reprocessable thermosets for sustainable three-dimensional printing, Nat. Commun., 2018, 9(1), 1831 CrossRef PubMed.
- J. Wu, J. Guo and C. Linghu,
et al., Rapid digital light 3D printing enabled by a soft and deformable hydrogel separation interface, Nat. Commun., 2021, 12(1), 6070 CrossRef CAS PubMed.
- F. Kotz, K. Arnold and W. Bauer,
et al., Three-dimensional printing of transparent fused silica glass, Nature, 2017, 544(7650), 337–339 CrossRef CAS PubMed.
- B. Zhang, H. Li and J. Cheng,
et al., Mechanically robust and UV-curable shape-memory polymers for digital light processing based 4D printing, Adv. Mater., 2021, 33(27), e2101298 CrossRef PubMed.
- M. Zarek, M. Layani and I. Cooperstein,
et al., 3D printing of shape memory polymers for flexible electronic devices, Adv. Mater., 2016, 28(22), 4449–4454 CrossRef CAS PubMed.
- N. A. Traugutt, D. Mistry and C. Luo,
et al., Liquid-crystal-elastomer-based dissipative structures by digital light processing 3D printing, Adv. Mater., 2020, 32(28), e2000797 CrossRef PubMed.
- S. Deng, J. Wu and M. D. Dickey,
et al., Rapid open-air digital light 3D printing of thermoplastic polymer, Adv. Mater., 2019, 31(39), e1903970 CrossRef PubMed.
- Y. F. Zhang, N. Zhang and H. Hingorani,
et al., Fast-response, stiffness-tunable soft actuator by hybrid multimaterial 3D printing, Adv. Funct. Mater., 2019, 29(15), 1806698 CrossRef.
- S. Peng, Y. Li and L. Wu,
et al., 3D printing mechanically robust and transparent polyurethane elastomers for stretchable electronic sensors, ACS Appl. Mater. Interfaces, 2020, 12(5), 6479–6488 CrossRef CAS PubMed.
- Q. Lv, X. Hu and X. Zhang,
et al., Highly efficient removal of trace metal ions by using poly(acrylic acid) hydrogel adsorbent, Mater. Des., 2019, 181, 107934 CrossRef CAS.
- X. N. Zhang, Q. Zheng and Z. L. Wu, Recent advances in 3D printing of tough hydrogels: A review, Composites, Part B, 2022, 238, 109895 CrossRef CAS.
- X. P. Hao, Z. Xu and C. Y. Li,
et al., Kirigami-design-enabled hydrogel multimorphs with application as a multistate switch, Adv. Mater., 2020, 32(22), e2000781 CrossRef PubMed.
- F. Zhu, L. Wang and B. Demir,
et al., Accelerating solar desalination in brine through ion activated hierarchically porous polyion complex hydrogels, Mater. Horiz., 2020, 7(12), 3187–3195 RSC.
- L. X. Hou, H. Ding and X. P. Hao,
et al., Multi-level encryption of information in morphing hydrogels with patterned fluorescence, Soft Matter, 2022, 18(11), 2149–2156 RSC.
- Y. Zhao, C. Xuan and X. Qian,
et al., Soft phototactic swimmer based on self-sustained hydrogel oscillator, Sci. Robot., 2019, 4(33), eaax7112 CrossRef PubMed.
- M. Kurdtabar, S. Saif Heris and M. Dezfulian, Characterization of a multi-responsive magnetic graphene oxide nanocomposite hydrogel and its application for DOX delivery, Chin. J. Polym. Sci., 2021, 39(12), 1597–1608 CrossRef CAS.
- C. N. Zhu, T. Bai and H. Wang,
et al., Dual-encryption in a shape-memory hydrogel with tunable fluorescence and reconfigurable architecture, Adv. Mater., 2021, 33(29), e2102023 CrossRef PubMed.
- J. Ren, X. Shu and Y. Wang,
et al., Key progresses of MOE key laboratory of macromolecular synthesis and functionalization in 2020, Chin. Chem. Lett., 2022, 33, 1650–1658 CrossRef CAS.
- S. Jung, J. L. Kaar and M. P. Stoykovich, Design and functionalization of responsive hydrogels for photonic crystal biosensors, Mol. Syst. Des. Eng., 2016, 1(3), 225–241 RSC.
- Qi Ge, Z. Chen and J. Cheng,
et al., 3D printing of highly stretchable hydrogel with diverse UV curable polymers, Sci. Adv., 2021, 7(2), eaba4261 CrossRef CAS PubMed.
- Y. He, R. Yu and X. Li,
et al., Digital light processing 4D printing of transparent, strong, highly conductive hydrogels, ACS Appl. Mater. Interfaces, 2021, 13(30), 36286–36294 CrossRef CAS PubMed.
- Y. He, F. Wang and X. Wang,
et al., A photocurable hybrid chitosan/acrylamide bioink for DLP based 3D bioprinting, Mater. Des., 2021, 202, 109588 CrossRef CAS.
- B. Grigoryan, S. J. Paulsen and D. C. Corbett,
et al., Multivascular networks and functional intravascular topologies within biocompatible hydrogels, Science, 2019, 364(6439), 458–464 CrossRef CAS PubMed.
- H. Quan, T. Zhang and H. Xu,
et al., Photo-curing 3D printing technique and its challenges, Bioact. Mater., 2020, 5(1), 110–115 CrossRef PubMed.
- O. Santoliquido, P. Colombo and A. Ortona, Additive Manufacturing of ceramic components by Digital Light Processing: A comparison between the “bottom-up” and the “top-down” approaches, J. Eur. Ceram. Soc., 2019, 39(6), 2140–2148 CrossRef CAS.
- M. Caprioli, I. Roppolo and A. Chiappone,
et al., 3D-printed self-healing hydrogels via Digital Light Processing, Nat. Commun., 2021, 12(1), 2462 CrossRef CAS PubMed.
- Y. Luo, C. K. Dolder and J. M. Walker,
et al., Synthesis and biological evaluation of well-defined poly(propylene fumarate) oligomers and their use in 3D printed scaffolds, Biomacromolecules, 2016, 17(2), 690–697 CrossRef CAS PubMed.
- Z. Liu, W. Pan and K. Wang,
et al., Acoustophoretic liquefaction for 3D printing ultrahigh-viscosity nanoparticle suspensions, Adv. Mater., 2022, 34(7), e2106183 CrossRef PubMed.
- H. Wang, B. Zhang and J. Zhang,
et al., General one-pot method for preparing highly water-soluble and biocompatible photoinitiators for digital light processing-based 3D printing of hydrogels, ACS Appl. Mater. Interfaces, 2021, 13(46), 55507–55516 CrossRef CAS PubMed.
- Y. Shen, H. Tang and X. Huang,
et al., DLP printing photocurable chitosan to build bio-constructs for tissue engineering, Carbohydr. Polym., 2020, 235, 115970 CrossRef CAS PubMed.
- B. D. Fairbanks, M. P. Schwartz and C. N. Bowman,
et al., Photoinitiated polymerization of PEG-diacrylate with lithium phenyl-2,4,6-trimethylbenzoylphosphinate: polymerization rate and cytocompatibility, Biomaterials, 2009, 30(35), 6702–6707 CrossRef CAS PubMed.
- A. A. Pawar, G. Saada and I. Cooperstein,
et al., High-performance 3D printing of hydrogels by water-dispersible photoinitiator nanoparticles, Sci. Adv., 2016, 2(4), e1501381 CrossRef PubMed.
- R. Cao, X. Pu and X. Du,
et al., Screen-printed washable electronic textiles as self-powered touch/gesture tribo-sensors for intelligent human-machine interaction, ACS Nano, 2018, 12(6), 5190–5196 CrossRef CAS PubMed.
- M. Hua, D. Wu and S. Wu,
et al., 4D printable tough and thermoresponsive hydrogels, ACS Appl. Mater. Interfaces, 2021, 13(11), 12689–12697 CrossRef CAS PubMed.
- Z. Sun, Y. Lu and Q. Zhao,
et al., A new stereolithographic 3D printing strategy for hydrogels with a large mechanical tunability and self-weldability, Addit. Manuf., 2022, 50, 102563 CAS.
- L. Huang, R. Jiang and J. Wu,
et al., Ultrafast digital printing toward 4D shape changing materials, Adv. Mater., 2017, 29(7), 1605390 CrossRef PubMed.
- M. P. de Beer, H. L. van der Laan and M. A. Cole,
et al., Rapid, continuous additive manufacturing by volumetric polymerization inhibition patterning, Sci. Adv., 2019, 5(1), eaau8723 CrossRef PubMed.
- C. Zhang, H. Zheng and J. Sun,
et al., 3D printed, solid-state conductive ionoelastomer as a generic building block for tactile applications, Adv. Mater., 2022, 34(2), e2105996 CrossRef PubMed.
- Y. Li, Q. Mao and X. Li,
et al., High-fidelity and high-efficiency additive manufacturing using tunable pre-curing digital light processing, Addit. Manuf., 2019, 30, 100889 CAS.
- B. Zhang, S. Li and H. Hingorani,
et al., Highly stretchable hydrogels for UV curing based high-resolution multimaterial 3D printing, J. Mater. Chem. B, 2018, 6(20), 3246–3253 RSC.
- H. Yang, M. Ji and M. Yang,
et al., Fabricating hydrogels to mimic biological tissues of complex shapes and high fatigue resistance, Matter, 2021, 4(6), 1935–1946 CrossRef CAS.
- D. Wu, J. Song and Z. Zhai,
et al., Visualizing morphogenesis through instability formation in 4D printing, ACS Appl. Mater. Interfaces, 2019, 11(50), 47468–47475 CrossRef CAS PubMed.
- J. L. Drury and D. J. Mooney, Hydrogels for tissue engineering: scaffold design variables and applications, Biomaterials, 2003, 24(24), 4337–4351 CrossRef CAS PubMed.
- X. Xue, Y. Hu and Y. Deng,
et al., Recent advances in design of functional biocompatible hydrogels for bone tissue engineering, Adv. Funct. Mater., 2021, 31(19), 2009432 CrossRef CAS.
- Y. J. Wang, X. N. Zhang and Y. Song,
et al., Ultrastiff and tough supramolecular hydrogels with a dense and robust hydrogen bond network, Chem. Mater., 2019, 31, 1430–1440 CrossRef CAS.
- H. Ding, X. N. Zhang and S. Y. Zheng,
et al., Hydrogen bond reinforced poly(1-vinylimidazole-co-acrylic acid) hydrogels with high toughness, fast self-recovery, and dual pH-responsiveness, Polymer, 2017, 131, 95–103 CrossRef CAS.
- M. Wang, W. Li and L. S. Mille,
et al., Digital light processing based bioprinting with composable gradients, Adv. Mater., 2022, 34(1), e2107038 CrossRef PubMed.
- Q. Ge, Z. Chen and J. Cheng,
et al., 3D printing of highly stretchable hydrogel with diverse UV curable polymers, Sci. Adv., 2021, 7(2), eaba4261 CrossRef CAS PubMed.
- A. S. Gladman, E. A. Matsumoto and R. G. Nuzzo,
et al., Biomimetic 4D printing, Nat. Mater., 2016, 15(4), 413–418 CrossRef PubMed.
- X. Xin, L. Liu and Y. Liu,
et al., 4D printing auxetic metamaterials with tunable, programmable, and reconfigurable mechanical properties, Adv. Funct. Mater., 2020, 30(43), 2004226 CrossRef CAS.
- Z. J. Wang, C. N. Zhu and W. Hong,
et al., Cooperative deformations of periodically patterned hydrogels, Sci. Adv., 2017, 3, e1700348 CrossRef PubMed.
- Z. J. Wang, W. Hong and Z. L. Wu,
et al., Site-specific pre-swelling-directed morphing structures of patterned hydrogels, Angew. Chem., Int. Ed., 2017, 56(50), 15974–15978 CrossRef CAS PubMed.
- B. P. Lee and S. Konst, Novel hydrogel actuator inspired by reversible mussel adhesive protein chemistry, Adv. Mater., 2014, 26(21), 3415–3419 CrossRef CAS PubMed.
- Z. J. Wang, C. Y. Li and X. Y. Zhao,
et al., Thermo- and photo-responsive composite hydrogels with programmed deformations, J. Mater. Chem. B, 2019, 7(10), 1674–1678 RSC.
- Z. Ji, C. Yan and B. Yu,
et al., 3D printing of hydrogel architectures with complex and controllable shape deformation, Adv. Mater. Technol., 2019, 4(4), 1800713 CrossRef.
- Z. Zhao, J. Wu and X. Mu,
et al., Desolvation induced origami of photocurable polymers by digit light processing, Macromol. Rapid Commun., 2017, 38(13), 1600625 CrossRef PubMed.
- Q. L. Zhu, C. Du and Y. Dai,
et al., Light-steered locomotion of muscle-like hydrogel by self-coordinated shape change and friction modulation, Nat. Commun., 2020, 11(1), 5166 CrossRef CAS PubMed.
- S. Y. Zheng, Y. Tian and X. N. Zhang,
et al., Spin-coating-assisted fabrication of ultrathin physical hydrogel films with high toughness and fast response, Soft Matter, 2018, 14(28), 5888–5897 RSC.
- S. Y. Zheng, C. Y. Li and M. Du,
et al., Programmable deformations of biomimetic composite hydrogels embedded with printed fibers, ACS Appl. Mater. Interfaces, 2020, 12(51), 57497–57504 CrossRef CAS PubMed.
- C. Y. Li, S. Y. Zheng and X. P. Hao,
et al., Spontaneous and rapid electro-actuated snapping of constrained polyelectrolyte hydrogels, Sci. Adv., 2022, 8(15), eabm9608 CrossRef CAS PubMed.
- Y. Dong, S. Wang and Y. Ke,
et al., 4D printed hydrogels: fabrication, materials, and applications, Adv. Mater. Technol., 2020, 5(6), 2000034 CrossRef CAS.
- Z. Wang, H. Cui and M. Liu,
et al., Tough, transparent, 3D-printable, and self-healing poly(ethylene glycol)-gel (PEGgel), Adv. Mater., 2022, 34(11), e2107791 CrossRef PubMed.
- C.-C. Kim, H.-H. Lee and K. H. Oh,
et al., Highly stretchable, transparent ionic touch panel, Science, 2016, 353(6300), 682–687 CrossRef CAS PubMed.
- M. Kaltenbrunner, T. Sekitani and J. Reeder,
et al., An ultra-lightweight design for imperceptible plastic electronics, Nature, 2013, 499(7459), 458–463 CrossRef CAS PubMed.
- X. P. Hao, C. Y. Li and C. W. Zhang,
et al., Self-shaping soft electronics based on patterned hydrogel with stencil-printed liquid metal, Adv. Funct. Mater., 2021, 31(47), 2105481 CrossRef CAS.
- Q. Peng, J. Chen and T. Wang,
et al., Recent advances in designing conductive hydrogels for flexible electronics, InfoMat, 2020, 2(5), 843–865 CrossRef CAS.
- H. Yuk, B. Lu and S. Lin,
et al., 3D printing of conducting polymers, Nat. Commun., 2020, 11(1), 1604 CrossRef CAS PubMed.
- D. Jiao, Q. L. Zhu and C. Y. Li,
et al., Programmable morphing hydrogels for soft actuators and robots: from structure designs to active functions, Acc. Chem. Res., 2022, 55(11), 1533–1545 CrossRef CAS PubMed.
- J. Nie, Q. Gao and Y. Wang,
et al., Vessel-on-a-chip with hydrogel-based microfludics, Small, 2018, 14(45), 1802368 CrossRef PubMed.
- Z. Jin, Y. Li and K. Yu,
et al., 3D printing of physical organ models: recent developments and challenges, Adv. Sci., 2021, 8(17), 2101394 CrossRef PubMed.
- H.-Y. Wu, L. Yang and J.-S. Tu,
et al., Hydrogels with dynamically controllable mechanics and biochemistry for 3D cell culture platforms, Chin. J. Polym. Sci., 2022, 40, 38–46 CrossRef CAS.
- G. Chen, X. Liang and P. Zhang,
et al., Bioinspired 3D printing of functional materials by harnessing enzyme-induced biomineralization, Adv. Funct. Mater., 2022, 2113262 CrossRef.
- Y. Li, Q. Mao and J. Yin,
et al., Theoretical prediction and experimental validation of the digital light processing (DLP) working curve for photocurable materials, Addit. Manuf., 2021, 37, 101716 CAS.
- J. P. Gong, Materials both tough and soft, Science, 2014, 344(6180), 161–162 CrossRef CAS PubMed.
- T. L. Sun, T. Kurokawa and S. Kuroda,
et al., Physical hydrogels composed of polyampholytes demonstrate high toughness and viscoelasticity, Nat. Mater., 2013, 12(10), 932–937 CrossRef CAS PubMed.
- S. Y. Zheng, H. Ding and J. Qian,
et al., Metal-coordination complexes mediated physical hydrogels with high toughness, stick–slip tearing behavior, and good processability, Macromolecules, 2016, 49(24), 9637–9646 CrossRef CAS.
- X. Dai, Y. Zhang and L. Gao,
et al., A mechanically strong, highly stable, thermoplastic, and self-healable supramolecular polymer hydrogel, Adv. Mater., 2015, 27(23), 3566–3571 CrossRef CAS PubMed.
- M. Wu, Q.-Y. Peng, L.-B. Han and H.-B. Zeng, Self-healing hydrogels and underlying reversible intermolecular interactions, Chin. J. Polym. Sci., 2021, 39, 1246–1261 CrossRef CAS.
- J. Kim, G. Zhang and M. Shi,
et al., Fracture, fatigue, and friction of polymers in which entanglements greatly outnumber cross-links, Science, 2021, 374(6564), 212–216 CrossRef CAS PubMed.
- H. Wei, M. Lei, P. Zhang, J. Leng, Z. Zheng and Y. Yu, Orthogonal photochemistry-assisted printing of 3D tough and stretchable conductive hydrogels, Nat. Commun., 2021, 12, 2082 CrossRef CAS PubMed.
- G. Guo, Q. Wu and F. Liu,
et al., Solvent-cast-assisted printing of biomimetic morphing hydrogel structures with solvent evaporation-induced swelling mismatch, Adv. Funct. Mater., 2022, 32, 2108548 CrossRef CAS.
- S. Hou, D. Sycks and H. F. Chan,
et al., 3D printing of highly stretchable and tough hydrogels into complex, cellularized structures, Adv. Mater., 2015, 27(27), 4035–4040 CrossRef PubMed.
Footnote |
† These authors contributed equally to this work. |
|
This journal is © The Royal Society of Chemistry 2022 |