DOI:
10.1039/D4SM00600C
(Review Article)
Soft Matter, 2024, Advance Article
Demulsification of Pickering emulsions: advances in understanding mechanisms to applications
Received
17th May 2024
, Accepted 20th August 2024
First published on 11th September 2024
Abstract
Pickering emulsions are ultra-stable dispersions of two immiscible fluids stabilized by solid or microgel particles rather than molecular surfactants. Although their ultra-stability is a signature performance indicator, often such high stability hinders their demulsification, i.e., prevents the droplet coalescence that is needed for phase separation on demand, or release of the active ingredients encapsulated within droplets and/or to recover the particles themselves, which may be catalysts, for example. This review aims to provide theoretical and experimental insights on demulsification of Pickering emulsions, in particular identifying the mechanisms of particle dislodgment from the interface in biological and non-biological applications. Even though the adhesion of particles to the interface can appear irreversible, it is possible to detach particles via (1) alteration of particle wettability, and/or (2) particle dissolution, affecting the particle radius by introducing a range of physical conditions: pH, temperature, heat, shear, or magnetic fields; or via treatment with chemical/biochemical additives, including surfactants, enzymes, salts, or bacteria. Many of these changes ultimately influence the interfacial rheology of the particle-laden interface, which is sometimes underestimated. There is increasing momentum to create responsive Pickering particles such that they offer switchable wettability (demulsification and re-emulsification) when these conditions are changed. Demulsification via wettability alteration seems like the modus operandi whilst particle dissolution remains only partially explored, largely dominated by food digestion-related studies where Pickering particles are digested using gastrointestinal enzymes. Overall, this review aims to stimulate new thinking about the control of demulsification of Pickering emulsions for release of active ingredients associated with these ultra-stable emulsions.
1. Introduction
Pickering emulsions, first described in the early 1900s,1,2 are extraordinarily stable dispersions of two immiscible fluids of any type; either oil-in-water (O/W), water-in-oil (W/O) or even multiple phases stabilized by solid particles instead of molecular surfactants. Although they were discovered more than a century ago, nowadays they have attracted renewed interest mainly due to both the high demand of decreasing the use of non-eco-friendly surfactants and the abundance of biocompatible, biodegradable and laboratory-synthesised tailored particles that are able to adsorb at oil–water interfaces.3–7 These emulsions are known for their ultrastability because the particles strongly adsorb to the oil–water interface with very high desorption energies of the order of several thousands of kBT (where kB is the Boltzmann constant and T is the temperature), which effectively delays the coalescence or Ostwald ripening of the droplets.8,9 Furthermore, Pickering emulsions can be stabilized by softer microgel particles,10 which can be made by the physical crosslinking of various animal and plant proteins. These microgel particles possess additional unique properties, such as softness, deformability, and porosity, enabling them to swell or contract under the influence of external stimuli.11,12 This makes them particularly fascinating materials when present at the fluid–fluid interface.5,13 There has been recent interest in Pickering emulsions stabilised by a binary (two component) mixture of particles, often termed as “hybrid” particles.7,14,15 Such hybrid particles formed via a range of associative interactions (covalent, electrostatic, van der Waals, hydrophobic and hydrogen bonds) enable the design of more complex interfaces to deliver functionalities unachieved by a single particle by varying the complementary benefits of the particles (e.g., various functional groups, sizes, modulus, opposing charges or wettability). This approach presents a promising solution to address the limitations of using single colloidal particles such as modifying wettability of a particle, where often addition of surfactants to adjust particle wettability can be costly, time-consuming, and often only partially effective.14
A concept that has not been widely explored in Pickering emulsions, particularly in biological applications such as foods and agrochemicals, is demulsification – processes that undermine the stability of the emulsion against coalescence leading to the separation of the two immiscible phases. Controlled release stands at the forefront of scientific innovation, offering a tailored approach to supply a diverse range of active ingredients—ranging from drugs and food additives to pesticides and fragrances. Whether it entails gradual, sustained release over an extended period or intermittent, targeted delivery of specific doses at predefined intervals at certain sites, on-demand demulsification of Pickering emulsions could be a necessary step. Unlike in foods, demulsification via chemical additives is a rather established field in the energy sector, such as petroleum production, where de-watering of crude oils is often a crucial part of the extraction process.16,17 Even though there has been significant research on demulsification of emulsions stabilized by synthetic particles in this sector,18,19 there are still uncertainties regarding the true mechanisms that trigger demulsification.19–21 In contrast, there has been limited research on demulsification of Pickering emulsions stabilized by biocompatible particles, which has many potential applications as controlled-release delivery systems in the food and pharma industries.
Although the adhesion of particles to oil–water interfaces can be strong and appear irreversible in many situations, it is possible to cause desorption or detachment of these particles through the application of a suitable stimuli such as pH, temperature, or mixing with secondary additives such as surfactants, enzymes, salts and bacteria.22,23 Thus, it is highly desirable to develop strategies for controlling the destabilization of Pickering emulsions, enabling design of demulsification on demand.24
Therefore, the aim of this review is to understand the mechanisms of demulsification in Pickering emulsions stabilized by particles in biological and non-biological systems. Firstly, we cover an insight into the detachment energy theories, where we highlight that the variation of the force for displacement of particles is largely governed by the particle wettability and size, plus the interfacial tension between the fluids. Therefore, we start with two key approaches of (1) changing the wettability of the particles, or (2) reducing the particle size or to achieve demulsification. Then we cover theoretical basis of how externally applied fields may dislodge a particle or a network of particles from the interface. We then discuss experimental work that has emerged in the last few years that highlights how these two approaches have been exploited with demulsification in non-biological fields − largely dominated by wettability modifications via chemical additives, pH, temperature, magnetic fields or their combined effects. Addition of chemical demulsifiers alters the interfacial rheology from an elastically dominated network to a viscous dominated network, thus enhancing mobility of the interfacial species that enables coalescence. In contrast, we pinpoint particle dissolution via enzymes as a preferred route for demulsification in biological applications. This area is largely dominated by numerous food-related gastrointestinal digestion-related studies, where proteinaceous25,26 or starch-based27 Pickering particles are degraded by physiological enzymes, such as α-amylase, pepsin and/or trypsin leading to droplet coalescence. We have limited our discussion in this domain to the principle of how enzymatic treatment may affects demulsification via altering particle wettability or reduction of particle size rather than the chemistry of digestion of proteinaceous/starch-based particles or how the droplet coalescence affects fatty acid release from the emulsion droplets. Readers may refer to such knowledge from recent reviews.27–30 Noteworthy, we also do not cover compositional ripening31,32 that may result in phase separation, and we only focus on the Pickering particles that may affect droplet breakup. Overall, this review should serve as a valuable resource for understanding demulsification, based largely on the desorption energy equation and inspire bottom-up design of particles for destabilisation on demand.
2. Particle detachment mechanism
A knowledge of the principles of demulsification requires an understanding of the factors that determine the stability of emulsions. Pickering emulsions (PEs) are stabilized by solid particles, mainly via removal of interfacial area (ΔA) or change in the interfacial area of contact, by adsorption of the particles, between the two immisicible fluids. This in turn leads to a change in the interfacial free energy i.e., by ΔAγ, where γ is the interfacial tension between the fluids. Whether the emulsion takes the form of W/O or O/W is determined by the wetting properties of the Pickering particles. This is elucidated by the Young–Dupre equation with the three-phase contact angle denoted as θ,33 involving various interfacial tensions such as γow for oil–water or γwo for water–oil, γaw for air–water or γao for air–oil, and γos for oil–solid or γws for water–solid or γas for air–solid. Eqn (1) describes the relationship between the detachment energy, ΔGd (= ΔAγ) of a spherical particle of radius r from the interface and the θ as follows:34 |
ΔGd = γπr2(1 − |cos θ|)2
| (1) |
The most stable PEs form when θ is close to 90° because the ΔAγ term is near maximal. Moderately hydrophilic particles (θ < 90°) tend to form O/W emulsions while slightly hydrophobic particles (θ > 90°) form W/O emulsions. Even for solid particles that are very small, ΔGd can be extremely large, e.g., for r = 50 nm, θ = 90° and γow = 50 mN m−1, ΔGd ≈ 105kBT. This energy difference is significantly higher compared to adsorption energy of classical surfactants, which typically have an ΔGd ≈ a few kBT, causing them to continuously transition between being attached and detached from the interface35 due to their Brownian (thermal) motion. Note that ΔGd in eqn (1) represents the energy difference of a particle that has been completely removed from the interface compared to its equilibrium position when sitting at the interface.36 This takes no account of the actual energy required to achieve this. The processes of detachment involves significant additional stored interfacial energy that is dissipated when the particle is dislodged. This will be discussed in the next section.
For demulsification, i.e., the PEs lose stability, there must be a significant lowering of ΔGd via a change in γ, θ, r or some combination of them. In other words, by manipulating the variables in the eqn (1) we can induce instability in the Pickering particles by two key approaches:
Approach (1) altering wettability (θ) by making the particle more hydrophilic/hydrophobic by reducing θ towards zero, or increasing it towards 180°, respectively. This can be also achieved by reducing γ between the phases by adding chemical/biochemical species which ultimately also affect θ as illustrated in Fig. 1.
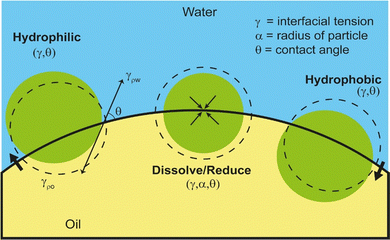 |
| Fig. 1 Different approaches to facilitate the process of destabilising Pickering emulsions where particles are shown by the green circle at the oil–water interface by changing their wettability of particles by making them more hydrophilic (left) or hydrophobic (right) via additives or dissolving the particles/reducing their size (middle). | |
Approach (2) dissolving the particle and therefore reducing its size, i.e., r.
In approach 1, adding a chemical additive into one of the fluid phases, as often practiced in the energy industry sector, one may easily be able to change γow or γwo by a factor of 10 mN m−1, or reduce θ to 15°. Once again these may cause a 10-fold reduction in ΔGd. In Approach 2 on the other hand, imagine, that you add an enzyme to dissolve a proteinaceous particle sitting at the interface with a contact angle of 30°. The radius may rapidly change say from 20 to 5 nm, with a clear impact in reducing the ΔGd from 165kBT down to 10kBT, if γ = 30 mN m−1. Now, the partially dissolved particle has a sufficiently low ΔGd so that there is a small but appreciable probability of overcoming this energy barrier under the influence of its own thermal motion, becoming detached from the surface and therefore leading to demulsification.
Also, it is noteworthy to mention that in practice most particle stabilizers are present in some aggregated configuration and may form a viscoelastic network at the fluid–fluid interface. This in itself can provide an extraordinary level of emulsion stability. In such a case, demulsification is largely dictated by reducing the propensity of the particles to stick to other particles, contributing to a elastically-dominated interfacial rheology. Simply put, this means that a large number of particles must leave the surface simultaneously for them to become removed from the interface. One may also think of such aggregates of particles as significantly larger single “effective” particle. Recall that an r value larger by 20 times implies a ΔGd that is 20 × 20 = 400 times bigger. The presence of such interfacial networks makes the challenge of demulsification much more difficult. Nonetheless, note that this consideration applies to scenarios involving spontaneous detachment of particles from the surface, due to Brownian motion. When external fields are applied to dislodge the particles from the interface, the forces applied to a cluster can also grow with the size of the cluster. Then, under suitable circumstances, it actually becomes easier to displace larger “effective” particles, as opposed to smaller ones. We briefly discuss such situations in the following section.
Another aspect to consider is the demulsification mechanism of microgel particles as they differ from those of true solid particles. The stability of these emulsions is intricately linked to the nature of the stabilizing microgel particles, whether they are more polymeric or colloidal, and the morphology they assume at the liquid–liquid interface.37,38 The colloidal properties of the microgels provide the foundation for the long-term stability of Pickering emulsions. However, the polymeric properties of the microgels allow them to spread and flatten at the liquid–liquid interface, which might influence the demulsification behaviour significantly. A key difference lies in the position of the liquid–liquid interface in defining the three-phase contact angle; for rigid particles, the interface rests on their surface, whereas for microgel particles, it is situated within them.39 We find few examples via demulsification of microgels in subsequent sections and further research is needed to have an in depth understanding of the desorption mechanisms of microgels.
3. Particle detachment due to the influence of an external field
Of all the possible ways to destabilise Pickering emulsion droplets, the most obvious might seem the direct removal of particles from the interface via the application of an external field. Depending on the nature of the particle, the fields in question can be electric, magnetic, gravitational, or even forces induced by the imposition of a shear flow. Though conceptually simple, in practice it is not so easy to entice a single isolated particle to leave the interface. Let us demonstrate this by considering a simple example of a charged particle of radius r, with a surface potential ζ. The total electric charge of the particle, assuming a low electrolyte solution (i.e. large Debye length ≫ r) will be 4πεrε0rζ, where ε0 is the permittivity of vacuum and εr ∼ 79, i.e., the relative permittivity of water. The force acting on the particle in an electric field E is thus 4πεrε0rζE. For the particle to be removed from the surface, it will need to be displaced by a distance of the order of its own size, that is a distance ∼r. We can now equate the energy decrease due to such a displacement with that typically needed for the removal of the particle, as given by eqn (2). Therefore, we arrive at 4πεrε0r2ζE ≪ πr2γ or |
 | (2) |
Note that in this case the field obtained is not size dependent, although for other types of field, e.g., gravitational, it can be. For a particle with ζ = 50 mV and γow = 30 mN m−1, the calculated electric field from the above equation is E = 214 MV m−1. This is a very high field indeed. To provide an idea of its magnitude, recall that most plastic insulating material will have a dielectric breakdown strength ∼1 to 100 MV m−1.
The above calculation thus seems to suggest that applying fields to destabilise emulsion droplets through the removal of particles from their surfaces may not prove a feasible technique for demulsification. It turns out that this conclusion is not in line with our practical experience, where application of electric fields,40–42 gravity,43 and in the cases of suitably paramagnetic particles a magnetic field,44,45 have all been reported to cause destabilisation of Pickering type emulsions. This discrepancy arises because the above calculation is based on the removal of a single isolated particle from the interface. It ignores the importance of the interparticle interactions that exist between the particles adsorbed on the surface of a droplet (or gas bubble, for that matter). In other words, forces exerted on one particle can be transmitted to neighbouring particles, and through them to particles further away. This rather important point was first recognised and verified through computer simulation work of Kim et al.46 In their work, the authors clearly demonstrated the accumulative effect of the forces exert on each individual particle. Thus, a particle not only felt the direct effect of the external field, but also that exerted by this field on other particles much further away, through the interactions with its neighbours. In a gravitation field, and for sufficiently large droplets considered by these authors, the force exerted on a particle at the bottom of droplet was found to be substantially greater than that due to the direct effect of the field on the particle. In fact, the accumulated experienced force increased with the size of droplet and hence the number of particles present on the surface. Kim et al.46 predicted that for sufficiently large droplets this will cause the detachment of particles from the bottom of the drop, a fact that was also verified experimentally later on.43
More recently Rong et al.47 considered the stability of Pickering emulsions under the influence of a flow field. These authors were interested in the colloidal stability of particle stabilised liquid marbles, used for capturing CO2 during fast affluent flow of gas. Before we discuss the results and the limit on flow for the stability (or conversely for destabilising) of particle stabilised droplets in such a flow field, it is useful first to give a somewhat more accurate picture of the detachment of a particle from a fluid–fluid interface. When a particle is slightly displaced from its equilibrium position on the interface, it initially forms a liquid bridge connecting it back to the interface Fig. 2. At some point and as the particle is moved further away, the bridge breaks and the interface relaxes back to its equilibrium position. The particle is now fully detached from the surface and resides in the bulk phase. However, the formation of the liquid bridge involves creation of additional interface between the two fluids. When the surface relaxes back, the stored interfacial energy in the liquid bridge is dissipated. The additional dissipated energy should be accounted for and added to the detachment energy as given by eqn (1) or (2) when accounting for the actual work needed to remove a particle from the surface. For large particles the effect is not large. However, for sub-micron sized particles it becomes increasingly important, to the extent that for particles below 100 nm the actual energy required for removing the particle can be several times larger than that estimated by the commonly quoted eqn (1).36,48 Over the years several researchers have attempted to calculate the force–displacement relation during the removal of a particle from the surface, prior to breakup of the liquid bridge. Most of the theoretical work had been on flat surfaces and in the presence of gravity,49–51 though more recent studies have also considered curved surfaces (such as those for droplets) in the absence of gravity both for spherical36,48,52 and spheroid shaped particles.53,54 For small displacements, the force–distance relation follows a simple linear relation f = Kx, somewhat akin to the particle being attached to the interface by a fictitious spring. The spring constant K, frequently referred to as “de Gennes–Hook” constant, was given by Ettelaie and Lishchuk36 to be:
|
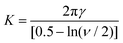 | (3) |
for small particle to droplet size ratios,
ν =
r/
R < 0.1 and for the case with a contact angle ∼90°.
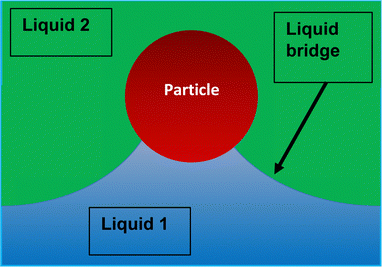 |
| Fig. 2 The removal of a particle from the interface between two immiscible fluids, showing the formation of a liquid bridge in the process. This involves additional stored interfacial energy, which is dissipated when the particle fully leaves the interface. For small particles this can greatly increase the energy needed to dislodge the particle. | |
Rong et al.47 considered the above equation in conjunction with the force experienced by the particles in a closed packed layer on the surface of a droplet, when the layer is subjected to a flow field as shown in Fig. 3a. Depending on its location on the surface, each particle receives a tangential shear force which is also transmitted to the neighbouring particles. The contact forces between the particles means that the particles are subjected to compressive stresses, which increase as one moves further upstream from one pole of the droplet to the other. The stress attains its highest value for the particle highlighted with the lighter colour in Fig. 3a.
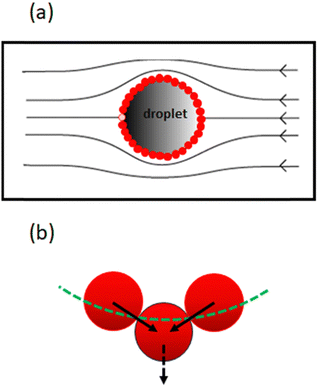 |
| Fig. 3 The flow (a) around a Pickering stabilised spherical droplet, inducing tangential shear forces on the hard-sphere particles adsorbed on its surface. Displacement of a particle (b) by a small distance away from its equilibrium position at the pole of the droplet (indicated by the more lightly shaded particle in a), where the maximum compressional stress due to shear forces is felt. Only two of the neighbours of the displaced particle are shown here for clarity. Such a displacement results in a force pushing the particle away from the interface. | |
This particle has to support the cumulative shear force acting on all the other particles downstream. If slightly displaced from its equilibrium position, the presence of the compressive stress results in a net force pushing this particle away from the surface (see Fig. 3b). The magnitude of this force was calculated as 6πηux, where u is the flow velocity around the droplet, η the viscosity of the dispersion medium and x the displacement from the equilibrium position. Rong et al.47 reasoned that when 6πηu is smaller than the de Gennes–Hook constant given by eqn (3), the restoring surface force is sufficient to pull the particle back onto the interface. However, when the reverse is true, the particle is pushed out and will be removed from the interface. Using this criterion, the critical flow velocity u* around the droplet is:
|
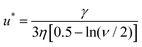 | (4) |
As an example, for oil droplets of size 10 μm, stabilised by particles of size 100 nm in an aqueous phase of viscosity η = 0.001 Pa s, a velocity greater than ∼1.72 m s−1 will be needed to destabilise the emulsion. This method for destabilising emulsions may well be more suited to W/O Pickering emulsions, where the viscosity of the continuous phase will generally be much higher.54
While the above situation only considers the contact forces between hard spheres, in reality the inter-particle forces may have a variety of other repulsive and attractive components. In the cases where such interactions cause clustering and aggregation of particles one must deal with the removal of particles as a collective process. Now one has to consider the desorption energy of the whole cluster, as well as how the net effect of the force on the cluster may scale with the size of the aggregates. This is an interesting problem that as yet does not seem to have received sufficient theoretical attention, and in particular where the aggregates may have open ramified (fractal) structures. We cover some of these aspects in the next section in reviewing the experimental papers.
4. Demulsification of Pickering emulsions in non-biological applications
Demulsification is rather an age-old process in non-biological applications, largely dominated by modification of γ and consequently alteration of θ i.e. Approach 1 as schematically presented in Fig. 4. One of the sectors exhibiting profound interest in demulsification using Approach 1 is the energy industry sector, i.e., petroleum production, with the need to rapidly dewater the crude oil prior to further downstream processing. With its complex chemistry, crude oil has several native species that can stabilize oil–water interfaces, such as resins, asphaltenes and clays. While all these species may contribute to stabilization of W/O droplets, it is often the asphaltenes that are the most difficult to desorb. Asphaltenes are complex, particulate-like materials composed of polyaromatic hydrocarbons, decorated with heteroatoms which make them interfacially active. Once adsorbed at the oil–water interface they often appear to become effectively irreversibly adsorbed and can network with neighbouring asphaltene molecules/nanoaggregates to form elastically dominated-interfaces. The resulting solid-like interfacial film prevents the coalescence of water droplets and slows down the rate of water separation.
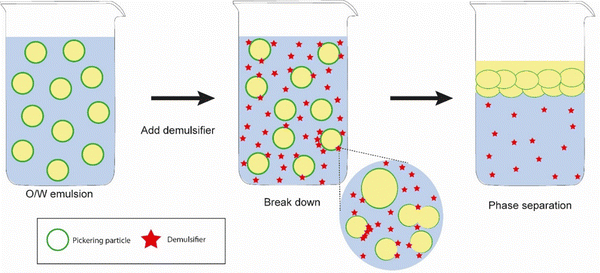 |
| Fig. 4 Schematic illustration of demulsification of Pickering emulsions stabilized by synthetic particles via competitive displacement, changing the species present at the interface using Approach (2) altering particle wettability. | |
Several studies have highlighted that the process of demulsification is closely linked to rheology of the interface.55–57 Demulsifier molecules act to decrease the interfacial elastic modulus, reducing film strength facilitating droplet coalescence.58 It is well known that the interfacial shear storage modulus (G′) and loss modulus (G′′) of the interface are indicative of emulsion stability, with G′ > G′′ correlating with stable emulsions provided the magnitude of G′ and G′′ are relatively high. Additionally, G′ is considered as a reliable indicator of attractive interfacial molecular interactions and cross-linking leading to elastic character of the particle–laden interface. As a crude rule of thumb in most practical applications, demulsifier molecules decrease the interfacial viscoelasticity to a specific threshold (G′ < G′′) leading to separation of water from the emulsions.59–61
To overcome the issues attributed to elastically dominated interfaces and to disrupt the attractive interactions between the particles at the interface, chemical demulsifiers are dosed into the W/O emulsion. These demulsifiers create purely viscous interfacial films under shear, hence when they compete at an interface and disrupt the species positioned at the interface, they lower the interfacial elasticity. The chemical demulsifiers used are typically polymer-like (e.g. ethylene oxide–propylene oxide (EO–PO)) that are strongly interfacially active62 (Table 1) so that they compete for available area at the interface, altering γ, increasing the surface pressure and so displace the asphaltenes, analogous to small molecule surfactants displacing large polymeric (protein) molecules.63 As the demulsifier molecules spread and occupy a greater interfacial area, they also lower the interfacial elasticity, transitioning the oil–water interface from being elastic to viscous dominant, as shown in Fig. 5. Then, when two water droplets come into contact, the interfacial material is more freely mobile, such that a liquid bridge can more easily form leading to coalescence.
Table 1 Demulsification by modifying the interfacial tension and/or particle wettability of Pickering particles
Particle |
Concentration |
Emulsion type |
Oil phase |
Demulsification strategy |
Ref. |
Asphaltenes (C5Pe and C5PeC11) |
0.3 wt% |
W/O |
Crude oil/bitumen |
Chemical additive: ethylene oxide and propylene oxide (EO–PO) |
62 |
Silica–benzaldehyde (SiO2–B) |
0.05 to 0.75 wt% |
O/W |
Paraffin |
pH: <3.5 |
64 |
Phosphatidylcholine-kaolinites |
0.5% (wt/v%) |
O/W |
Medium-chain triglycerides (MCT) |
pH: 7.2 |
65 |
Mesoporous silica nanoparticles (MSNPs)-cetyltrimethylammonium bromide (CTAB surfactant) |
0.3 wt% MSNPs with different concentrations of CTAB |
O/W |
n-Octane |
pH: NaOH (equimolar NaOH with respect to CTAB) |
66 |
Silica nanoparticles |
0.1 wt% with different concentrations of charge-reversible surfactant, 11-(N,N-dimethylamino) sodium undecanoate (DMUa) |
O/W |
n-Octane |
pH: 7.5 ± 0.2 |
67 |
Chitosan (CS)–poly(N-isopropylacrylamide (pNIPAM)) |
0.05 wt% |
O/W |
Corn/sunflower oil |
Multiple stimuli: pH: <2.0, temperature: >80 °C |
68 |
Poly(oligoester)–poly(ethylene glycol)methyl ether methacrylate (EG4) nanoparticles |
5, 2.5, 1 and 0.5 wt% |
O/W |
Limonene |
Temperature: 70 °C/2 hours |
69 |
Hybrid microgel particles (fumed silica, pNIPAM) |
2% v/w |
W/O |
Isooctane, cyclohexane, toluene |
Temperature: 42 °C |
15 |
CS-sodium 11-(butylselenyl)undecylsulfate (C4SeC11S) |
0.2 wt% |
O/W |
Liquid paraffin |
Multiple stimuli: redox: H2O2 (equimolar H2O2 with respect to C4SeC11S) |
70 |
|
|
|
Ion: CTAB (equimolar CTAB with respect to C4SeC11S) |
|
|
|
pH: 6.7–7.7 |
CS-sodium dodecylsulfate (SDS) |
0.2 wt% CS with 1 mM SDS |
O/W |
Isopropyl tetradecanoate |
Ion: CTAB (equimolar CTAB with respect to SDS) |
71 |
(pNIPAM-co-4-vinylpyridine) (pNIPAM-co-4VP)-pNIPAM-co-(methacrylic acid) (PNIPAM-co-MAA) |
0.1 wt% 4VP or MAA (water-swollen microgels) for O1/W emulsion |
(O1/W/O2) |
Toluene |
Temperature: 5 °C: W/O2 broken, maintain the O1/W emulsion, 50 °C: O1/W emulsion broken |
72 |
0.1 wt% octanol-swollen microgels for the W/O2 |
|
|
|
Fe3O4–SiO2–CS |
0.5 wt% at different CS molecular weight (2.5, 10 and 30 kDa) |
O/W |
Liquid paraffin |
Multiple stimuli: magnetic field: ≈0.4 T at pH 3.0–11.0 |
73 |
|
|
|
pH: 7.0 (≈pKb (CS)) |
Fe3O4–SiO2–pNIPAM |
0.01–0.5 wt% |
O/W |
Toluene |
Multiple stimuli: magnetic field: ≈33.48–63.45 emu g−1 at 45 °C |
74 |
|
|
|
Temperature: >31 °C |
Glycerol monostearate (GMS) |
4 wt% |
W/O |
Canola oil |
Chemical additive: ≥2 wt% sorbitan monooleate (SMO) |
75 |
GMS |
4 wt% |
W/O |
Canola oil |
Chemical additive: ≥0.5 wt% of SMO, sorbitan monolaurate (SML) and citric acid esters of monoglycerides (CITREM) |
76 |
CS-hydrophobic alginate nanocomposites (HSA-CS NCs) |
0.5, 1, 2 and 3 wt% |
O/W |
Corn oil |
pH: 6.8 |
77 |
CS-carrageenan (CRG) microgels |
0.9 wt% |
W/O |
Palmolein |
Multiple stimuli: temperature: >40 °C |
78 |
|
|
|
pH: >7.5 |
Cationic starch nanoparticles (CSNPs) |
0.5 wt% |
O/W |
PPG 15 |
pH: 2.0 |
79 |
Bacterial cells (M. neoaurum) |
1.33 wt% |
W/O |
Bis-(2-ethylhexyl) phthalate (BEHP) |
Chemical additive: modified silica particles with different degree of hydrophobicity |
80 |
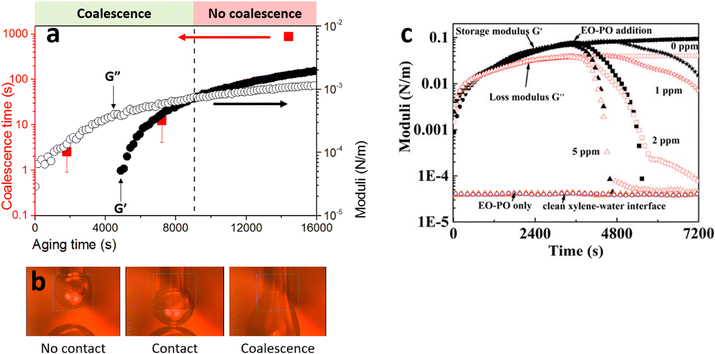 |
| Fig. 5 Critical role of interfacial shear rheology modifying the coalescence time of two contacting droplets (a). Initially, when an interface is formed the shear response is purely viscous (aging time = 0 s). Then as the chemical species (in this case asphaltenes) partition at the oil–water interface the viscous component increases and a critical concentration is reached when the chemical species interact to form a contiguous network that has shear elasticity. The interfacial film stiffens as more chemical species partition at the oil–water interface, leading to a transition from being viscous dominant to elastic dominant. When viscous dominant two contacting droplets can coalesce (b), however, when the interfacial film is solid-like (G′ > G′′), the yield stress of the film must be exceeded to coalesce droplets. The effect of adding a chemical demulsifier is to reduce the elastic contribution and transition the interfacial film from a solid-like to a liquid-like response (c). This occurs because chemical demulsifiers have no G′ contribution in shear, and so as the molecules occupy more surface area the elasticity of the interfacial film diminishes. Parts (a) and (b) are adapted57 and part (c) is reproduced62 with permission from ACS. | |
Besides asphaltenes, particles like silica (SiO2), polymer based systems including poly(N-isopropylacrylamide) (pNIPAM), poly(methyl methacrylate) (PMMA) and polystyrene (PS) have been studied as stabilisers of Pickering emulsions, showing various degrees of possible demulsification via chemical additives or adjustment of environmental conditions to alter particle contact angle θ.
From the articles compiled in Table 1, we briefly discuss various conditions that facilitate the demulsification of Pickering emulsions in a non-biological setting – where developing responsive particles with functional groups is rather straightforward compared to food applications. The most prevalent approach described in literature employs strategic manipulation of pH or temperature or a combination of stimuli, i.e. pH, ions (solution conductivity), and temperature together. Note that demulsification efficiency is often used as a quantitative term to express the extent of demulsification, which is the fraction of oil that has been phase separated from the emulsion:
|
 | (5) |
where
V0,oil is the original oil volume present in the emulsions at time (
t) = 0 and
Vt,oil is the separated oil volume at time
t. In recent literature, demulsification efficiency is also expressed indirectly as % of active ingredients released from the droplet due to the droplet breakup. Often demulsification efficiency is not reported in literature and rather microstructural and interfacial rheological data are shown to evidence the detachment of the particles and/or breakup of the interfacial barrier layer. Nevertheless, we highlight few examples of such demulsification efficiency in the following sections where it is linked to either of the two approaches of altering particle wettability or affecting particle dissolution.
4.1 pH-Induced destabilization
There is an increasing momentum to design pH-switchable Pickering particles by incorporating functional groups within the particles such that they may cause spontaneous demulsification by altering θ via change in pH of the continuous phase. Ren et al.64 recently demonstrated this approach using the so-called “dynamic covalent bond” where reversible switching on/off of the imine bond in silica–benzaldehyde nanoparticles (SiO2–B) via a pH change led to demulsification. The hydrophobic SiO2–B formed stable O/W Pickering emulsions at pH 7.8. Reducing the pH to 3.5 triggered dissociation of the covalent bond separating the amino silica (SiO2–NH2) and benzaldehyde. This reduced θ, and facilitated dislodging of the particles to the continuous phase, resulting in droplet coalescence. Study of combinations of particles and surfactants66,67 to achieve pH-induced demulsification has been an area of interest in colloid science. For instance, in one study,66 mesoporous nanosilica particles (MSNPs) were combined with the cationic surfactant cetyltrimethylammonium bromide (CTAB) to stabilize O/W Pickering emulsions that had high sensitivity to acid and base. NaOH induced rapid demulsification as the MSNPs nanoparticles partitioned into the water phase due to their increased (negative) surface charge and therefore loss of amphiphilicity via a reduction in θ. Subsequent addition of HCl enhanced the stabilizing properties of the system upon re-homogenization. The same group used MSNPs combined with dodecyltrimethylammonium bromide (DTAB) but now where the particle morphology varied between spherical, rod-like, or thread-like.81 Strikingly, although the spherical as well as rod-like nanoparticles were dislodged at high pH, as with the MSNP-CTAB system, demulsification was not observed with the thread-like particles, that were entangled at the interface, thus effectively forming a cross-linked two-dimensional network structure with substantial interfacial viscoelasticity. Consequently, although θ of the MSNPs was changed in the same way when NaOH was added, and the droplets became larger via Ostwald ripening, they remained interlocked in the particle network with the enhanced interfacial rheology of the thread-like MSNPs restricting complete droplet breakup.81 This example highlights the additional importance of particle–particle interactions and the effects of interfacial rheology that are not taken into account in the eqn (1), as well as the effective increase in r when the primary stabilizing particles tend to entangle or aggregate, increasing the magnitude of ΔGd in eqn (1).
4.2 Temperature-induced destabilization
Droplet destabilization can also proceed if the particles are thermo-responsive. Paramount amongst such systems are poly(n-isopropylacrylamide) (pNIPAM)-based particles.82 Below its low critical solution temperature (LCST), pNIPAM chains exist as coils due to hydrogen bonding of the amide groups with water: the polymer is swollen and can be used to form microgels. However, above LCST (32 °C83) the hydrogen bonding weakens, the polymer chains aggregate and the microgels collapse (r changes) and θ changes. In a molecular dynamics (MD) simulation of chitosan-pNIPAM (CS-pNIPAM68) particles it was predicted that increasing temperature would reduce r alongside increasing θ due to increased hydrophobicity of pNIPAM, leading to demulsification as strong aggregation of hydrophobic groups of pNIPAM leads to particle partitioning to the hydrophobic phase (Table 1).
Use of pNIPAM has also enabled demulsification in hybrid microgel particles, where these binary particles have been prepared using silica and pNIPAM to stabilise W/O interfaces15 (Table 1). This not only enabled demulsification as a result of temperature-induced phase inversion of pNIPAM, but the hybrid microgel particles also displayed switchable wettability between hydrophobicity and hydrophilicity, offering in situ switching between W/O or O/W Pickering emulsions via temperature change. Similarly, use of binary pNIPAM microgels (pNIPAM-co-4-vinylpyridine) (pNIPAM-co-4VP) and (pNIPAM-co-methacrylic acid) (pNIPAM-co-MAA) has also been used to prepare Pickering oil-in-water-in-oil (O/W/O) double emulsions, demulsified by exploiting the hydrophilicity/hydrophobicity transition of the microgels at different temperatures, allowing selective and rapid release of encapsulated active ingredients.72 Particularly, using perylene as a model substance, they measured demulsification efficiency with significant increase in the release of perylene molecules, from 55% to 88% within 10 min, reaching approximately 90% after 12 h of heating at 50 °C.
In another study, limonene-in-water Pickering emulsions were destabilised by increasing the temperature in order to release the fragrance within the droplets stabilised by polymeric nanoparticles69 (Table 1). The emulsions were incubated at 30 °C (Tcp, i.e., the temperature of the cloud point) for 2 h, and the amount of separated limonene was measured. As one might expect, below the Tcp the demulsification efficiency measured in terms of amount of released limonene was lower than 5%, whereas a significantly higher amount of limonene (up to 63%) was released when the temperature was above Tcp, the latter altering the θ of the nanoparticle contact angle θ. These findings demonstrate that modifying the wettability on non-biological particles can destabilize emulsions and facilitate the subsequent release of the encapsulated active ingredients by simply applying external conditions.
4.3 Multi-responsive destabilization: synergistic use of temperature, pH, ionic strength and redox agents
A more complex strategy involves the development of multi-stimuli-responsive Pickering emulsions, exploiting synergies in the responsiveness of the stabilizing particles to temperature, pH, ions and also redox conditions. For instance, emulsions stabilized by CS–C4SeC11S particles, formed by electrostatic attraction between Se-containing anionic surfactant C4SeC11S and chitosan (CS) at acidic pH, demonstrated demulsification due to the C4SeC11S responding to addition of H2O2, other ions (CTAB) as well as pH70 (Table 1). In another study, a composite Pickering emulsifier formed from Fe3O4, silica, and CS was used. The particles demonstrated paramagnetic and pH responsiveness. At 298 K, stable paraffin O/W emulsions were formed at pH 2.0, rapid demulsification could be triggered at pH 3.0 to 11.0 under a 0.4 T magnetic field dislodging the Fe3O4, whilst demulsification occurred at pH > pKb of CS (= pH 7.0), largely associated with deprotonation and precipitation of CS. These afore-mentioned studies suggest such organic–inorganic multiple responsive composite emulsifiers have great potential for on-demand destabilisation.73 However, in none of these studies has particle dissolution been targeted as a modus operandi of demulsification, which is probably the more preferred route in biological applications, largely dominated by digestion studies and through the use of enzymes; this is discussed in the next section.
5. Demulsification of Pickering emulsions in food and allied biological applications
In the field of food and pharmaceutical applications, demulsification of Pickering emulsions could facilitate the delivery of active ingredients to specific sites of interest such as the small intestine or colon. One of the classic ways to demulsify Pickering emulsions that occur in biological systems would be to employ the (2) particle dissolution approach – as would be expected to occur during digestion of food or drug delivery vehicles – depending upon the chemical nature of the particles. So, for example, proteinaceous Pickering particles like whey protein microgels are digested at the interface by gastrointestinal enzymes such as pepsin and trypsin,26,35 resulting in droplet coalescence. This particle dissolution route is schematically illustrated in Fig. 6, where it is tacitly assumed that enzyme activity is not compromised by the presence of the interface itself. Note, enzymes are themselves proteins and adsorption at bare patches of interface can sometimes cause them to unfold and lose their activity.84 On the other hand, the protein substrate itself will tend to be more unfolded when converted into microgels and therefore it might be even easier for the active site of the enzyme to access the relevant sections of the polypeptide chain and promote chain scission. Designing emulsions that have in-built controlled demulsification throughout the GI tract is vital for optimizing the delivery of numerous bioactives. Of course, the Pickering particle must also be biocompatible, biodegradable and non-toxic where food, agrochemicals and pharmaceutical systems are concerned. Examples from the literature of such materials include hydroxyapatite, calcium phosphate, tri-calcium phosphate, and calcium carbonate, cellulose nanocrystals, chitin nanocrystals, modified starch, soy protein nanoparticles, flavonoid particles, micellar casein-coated, whey protein microgels (already mentioned above), and various microgels produced from plant based materials.12
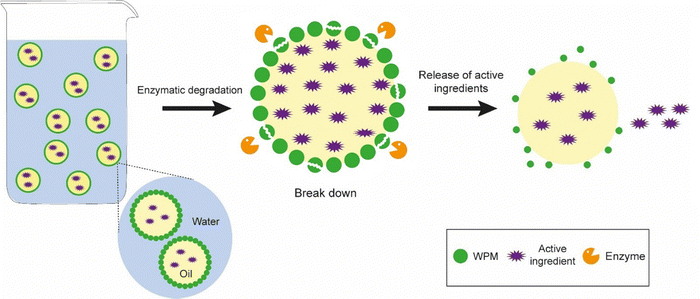 |
| Fig. 6 Mechanism of demulsification of Pickering emulsions stabilized by biocompatible particles where Approach (2) involving particle dissolution is employed, i.e., reducing the particle radius. | |
Table 2 presents a compilation of recent studies where biocompatible particles have been used as stabilizers and demulsification occurs via Approach (2), i.e., particle dissolution reducing r via pH change or application of enzymes. We have only included the studies where the primary purpose was to disintegrate the particles and eventually demulsify the emulsion rather than complete digestion, since the latter has already been well reviewed in literature extensively.25,30,85 We first discuss the studies where particle dissolution is a primary demulsification strategy followed by altered θ, an approach that is more common in the chemical industry sector.
Table 2 Demulsification by particle dissolution and size reduction of biological Pickering particles
Particle |
Concentration |
Emulsion type |
Solvent |
Demulsification strategy |
Ref. |
Calcium carbonate nanoparticle (CaCO3) |
4% (w/v) |
O/W |
MCT oil |
pH: 2.5 |
86 |
Alkylated-dextran nanoparticle |
0.6 mg mL−1 |
O/W |
Dodecane and MCT oil |
Enzyme: dextranase |
87 |
Acetalized starch-based nanoparticles (Ace-SNPs) |
2 mg mL−1 |
O/W |
Squalene |
pH: 5.4 |
88 |
Alkylated dextran-based nanoparticles |
0.16 mg mL−1 oil |
O/W |
Dodecane (model oil) and MCT (biocompatible oil) |
pH: 4.8 |
89 |
High methoxyl pectin (HMP) and whey protein isolate (WPI) complex (W-H-II) |
W-H-II nanocomplex ratio 1 : 2. WPI (0.9% w/v) : HMP (1.8% w/v) |
O/W |
Thyme oil |
Enzyme: pectin methylesterase |
90 |
Glycosylated whey protein isolate (gWPI) |
1% (w/v) |
O/W |
Algae oil |
Enzyme: pancreatic enzymes |
91 |
Media-milled black rice particles |
6% (w/w) |
O/W |
Soybean oil |
Enzyme: pancreatic enzymes |
92 |
Media-milled purple sweet potato particle |
20% (w/v) |
O/W |
Flaxseed oil |
Enzyme: pancreatic enzymes |
93 |
Soy protein hydrolysate microgel particles (SPHMs) |
3% (w/w) |
O/W |
Soybean oil |
Enzyme: pancreatic enzymes pH: 9.0 |
94 |
Sugary maize dendrimer-like glucan modified with octenyl succinic anhydride (OSA-SMDG) |
3%, 5% (w/w) |
O/W |
MCT oil |
Enzyme: α-amylase |
95 |
5.1 Dissolution and size reduction of Pickering particles
5.1.1 pH-Induced destabilization. Recent research has revealed strategies for inducing destabilization of Pickering particles by manipulating their size through pH modulation. One study used calcium carbonate nanoparticles (CaCO3 NPs) to create a stable edible oil-in-water Pickering emulsion (Table 2), suitable for delivering lipophilic drugs and providing oral calcium supplementation. The emulsion exhibited acid-triggered dissolution of the NPs to Ca2+ in a simulated gastric environment, resulting in droplet coalescence and release of encapsulated vitamin D386 from the dispersed phase in a simulated intestinal fluid. In another study, hydrophobic acetal-modified starch-based nanoparticles (Ace-SNPs), where hydrophilic hydroxyl groups in amylopectin were modified to cyclic acetal or acyclic acetal groups,88 were used as Pickering stabilizers to produce model drug (curcumin)-loaded emulsions. Although the Ace-SNPs hydrolyzed in an acidic environment and reduced the particle size, the authors only commented on changes in θ as a cause of demulsification. This may have been due to the possibility of the dissolution being erosive, i.e., proceeding from the outside inwards. Looking at demulsification efficiency,88 in vitro drug release experiments revealed that 50% of curcumin encapsulated with these Ace-SNP-stabilised Pickering droplets was released within 12 h in an acidic environment (pH 5.4) owing to the changes in θ, while only 14% was released at a higher pH (7.4) over the same period. Dextran has also been used to formulate biodegradable pH-sensitive Pickering emulsions. Modified into pH-sensitive acetylated dextran,89 nanoparticles of the acetylated dextran, obtained via precipitation, degraded under acidic conditions (Table 2). In these nanoparticle-stabilized oil-in-water Pickering emulsions, pH modulation induced droplet destabilization in less than 24 hours.
5.1.2 Enzyme triggered destabilization. In an interesting food-based study, a novel preservation strategy for fresh-cut apples using a pectin methylesterase (PME)-responsive nanocomplex (W-H-II) was used to stabilize a Pickering emulsion containing thyme essential oil (TEO).90 It was found that W-H-II, formed by heating whey protein isolate (WPI) + high methoxyl pectin (HMP), demonstrated good pH stability, whilst demonstrated droplet coalescence upon enzymatic degradation of the pectin part of the particle and eventual reduction in r supporting Approach 2. Maingret et al.87 on the other hand have developed Pickering emulsions stabilized by self-assembled alkylated-dextran nanoparticles. Using dextranase enzyme, the degradation of the nanoparticles led to emulsion destabilization. Although one might assume enzymatic modification may just alter r, in this particular case, contribution of Approach 1 cannot be ignored. The alkylated-dextran nanoparticles had a hydrophobic exterior, but when they were hydrolyzed from within (via the dextranase), the resulting fragments will have had a different θ in addition to the reduction in r. Enzymatic hydrolysis of a completely amorphous particle, with no distinction between its surface layers and its interior, might induce destabilisation solely via Approach 2 (particle size reduction and dissolution). However, in practice it is not so certain that the fragments will have the same balance of surface and bulk properties, depending the actual points of fragmentation of a hetero- or co-polymer and particularly in the case of protein-based particles, which possess functional groups of widely varying polarity. Hence, a combination of approaches 1 and 2 seem to most plausible scenario in such situations.
5.2 Modifying the wettability of biological Pickering particles
5.2.1 Surfactant-induced wettability changes. Using approach 1 i.e. modifying θ of Pickering particles by making them either more hydrophilic or hydrophobic is not uncommon in food and drug delivery applications. Water-in-oil (W/O) emulsions, formed using high-melting surfactant glycerol monostearate (GMS), developed interfacial crystalline shells that efficiently delayed salt release from aqueous droplets. The introduction of a non-ionic surfactant, sorbitan monooleate (SMO), enabled salt release by gradually displacing the interfacially-adsorbed GMS layer and changed the wettability of GMS75 (Table 1). The same group of authors76 further demonstrated that several surfactants such as SMO, sorbitan monolaurate (SML), and citric acid esters of monoglycerides (CITREM) were effective demulsifiers, modifying the θ of the GMS crystals by disrupting the interfacial film, with NaCl release from the internal phase. The alteration of θ of the GMS crystals also affected demulsification efficiency as evaluated by measuring the release of the encapsulated NaCl from the droplets after adding the afore-mentioned surfactants.76 Within two hours, SMO showed the highest NaCl release, with 30.0% at 1 wt% and 20.6% at 0.5 wt%. CITREM followed closely, releasing 27.4% NaCl at 1 wt% and 18.7% at 0.5 wt%, whilst SML released 23.9% and 14.8% NaCl at 1 wt% and 0.5 wt%, respectively.
5.2.2 pH-Induced wettability changes. Eco-friendly, pH-responsive cationic starch nanoparticles (CSNP) were synthesized via ethanol precipitation from pH-sensitive starch, copolymerized by grafting with dimethylaminoethyl methacrylate (DMAEMA). Emulsions stabilised by such nanoparticles exhibited colloidal stability through 6 pH cycles.79 The CSNP-stabilized emulsions remained stable at pH 7 but the emulsions broke at pH 2. This was due to the protonation of the amino groups on the DMAEMA at low pH, making them far more hydrophilic (hence reducing θ). A Pickering emulsion stabilised by chitosan–hydrophobic alginate nanocomposites (HSA-CS NCs) has been proposed as a drug-loading vehicle.77 This vehicle displayed pH-responsive and biocompatible features via the HSA-CS, with high loading capacity and a rigid layer surface film. No release was observed in the gastric phase whilst controlled-release behavior was observed in the intestinal phase, with 88.37% ibuprofen released over 24 h, making it a promising pH-responsive vehicle for oral drug delivery. Diffusive release of the ibuprofen was influenced by the contraction, swelling and expansion of this charge-varying bionanocomposite at the interface of the Pickering stabilised droplets,77 significantly affecting the θ of these particles.
5.2.3 Multi-responsive changes in θ. Multi-stimuli-responsive Pickering emulsions, exhibiting synergy in responsiveness to temperature and pH conditions. In an interesting study, chitosan-carrageenan (CS–CRG) composite microgels have been developed as dual stimuli-responsive Pickering emulsifiers, showing pH and thermoresponsiveness.78 The stability of the CS–CRG stabilised Pickering emulsions depends on the CS
:
CRG mass ratio and oil volume fraction, remaining stable at acidic pH and temperatures below 40 °C, but exhibiting demulsification at alkaline pH and temperatures above 40 °C. The deprotonation of chitosan within the CS–CRG composite microgels at alkaline pH presumably disrupted the equilibrium between the hydrophilic and hydrophobic properties of the CS–CRG composite microgels. Consequently, the microgels lost their ability to stabilize the droplets against coalescence. Likewise, the combined impact of heat-induced deprotonation of chitosan and the coil-to-helix transformation of CRG at temperatures exceeding 40 °C compromised the stability of CS–CRG composite microgels, also leading to demulsification. Such multi-responsive Pickering emulsions, based on food-grade biocompatible materials, hold great promise for developing controlled release in many systems. Nevertheless, it is clear that demulsification of Pickering emulsions stabilized by biocompatible particles is a relatively new area of research and much more work is necessary to enhance our understanding of the mechanisms involved for the optimization of single or multiple stimuli processes.96
6. Conclusions and future perspectives
In conclusion, we can see that in some applications the extreme stability of Pickering emulsions can pose problems, particularly in downstream processing and this review has highlighted strategies that could be adopted to dislodge the particles from the interface. Demulsification can be achieved by broadly two different approaches (1) altering wettability and/or (2) particle dissolution. Altering wettability has been the preferred strategy in the chemical sector whilst particle dissolution remains mostly associated with enzymatic digestion of biodegradable particles. In terms of practical applications any digestion process will probably also affect wettability. An innovative approach that deserves much more attention is to design in (1) or (2) into the initial Pickering particles, triggered by an external stimulus such as temperature, pH, redox reagents or magnetic fields. Most systems studied are W/O or O/W but demulsification of W/W systems also needs to be investigated. In terms of future perspectives and practical applications, the above principles could perhaps be explored more readily in the traditional de-watering of crude oil, but more innovative applications probably lie in the controlled release of active ingredients in drug, agrochemical, cosmetic, personal care and food systems. In this case the Pickering particle itself might be the active ingredient, irreversibly adsorbed at the surface of the colloid until it is needed to be released at the site of delivery. Many drugs and food components possessing potential anti-oxidative properties fall into this class, as well as components possessing colouring, flavouring or specific nutritive properties. Agrochemical actives often need to switch their wetting properties so that they adhere to specific plant surfaces or those of pests. Regarding digestion, many insoluble food ingredients can persist at interfaces, but the effects of the degree of desorption on the overall nutrition and health outcomes remain largely unknown. In this case the effects of the full range of physical97 and physiological conditions on particle desorption need to be explored much more widely.
Data availability
No primary research results, software or code have been included and no new data were generated or analysed as part of this review.
Conflicts of interest
There are no conflicts to declare.
Acknowledgements
Authors gratefully acknowledge the Engineering and Physical Sciences Research Council (EPSRC) funded Centre for Doctoral Training in Molecules to Product, Grant Ref. No. EP/S022473/1 for financial support.
References
- S. U. Pickering, J. Chem. Soc., Dalton Trans., 1907, 91, 2001–2021 RSC.
- W. Ramsden and F. Gotch, Proc. R. Soc. London, 1997, 72, 156–164 Search PubMed.
- A. Sarkar and E. Dickinson, Curr. Opin. Colloid Interface Sci., 2020, 49, 69–81 CrossRef CAS.
- B. S. Murray, Curr. Opin. Food Sci., 2019, 27, 57–63 CrossRef.
- B. S. Murray, Adv. Colloid Interface Sci., 2019, 271, 101990 CrossRef CAS PubMed.
- S. Lam, K. P. Velikov and O. D. Velev, Curr. Opin. Colloid Interface Sci., 2014, 19, 490–500 CrossRef CAS.
- N. Nimaming, A. Sadeghpour, B. S. Murray and A. Sarkar, Trends Food Sci. Technol., 2023, 138, 671–684 CrossRef CAS.
- B. P. Binks and T. S. Horozov, Colloidal Particles at Liquid Interfaces, Cambridge University Press, Cambridge, 2006 Search PubMed.
- B. P. Binks, Curr. Opin. Colloid Interface Sci., 2002, 7, 21–41 CrossRef CAS.
- D. Z. Akgonullu, B. S. Murray, S. D. Connell, Y. Fang, B. Linter and A. Sarkar, Adv. Colloid Interface Sci., 2023, 320, 102983 CrossRef CAS PubMed.
- E. Andablo-Reyes, D. Yerani, M. Fu, E. Liamas, S. Connell, O. Torres and A. Sarkar, Soft Matter, 2019, 15, 9614–9624 RSC.
- S. Zhang, M. Holmes, R. Ettelaie and A. Sarkar, Food Hydrocolloids, 2020, 102, 105583 CrossRef CAS.
- I. Navarro Arrebola, L. Billon and G. Aguirre, Adv. Colloid Interface Sci., 2021, 287, 102333 CrossRef CAS PubMed.
- L. Liu and T. Ngai, Langmuir, 2022, 38, 13322–13329 CrossRef CAS PubMed.
- H. Jiang, S. Zhang, G. Sun, Y. Li, X. Guan, C. Yang and T. Ngai, Chem. Sci., 2022, 13, 39–43 RSC.
- M. A. Saad, M. Kamil, N. H. Abdurahman, R. M. Yunus and O. I. Awad, Processes, 2019, 7, 470 CrossRef CAS.
- N. Hassanshahi, G. Hu and J. Li, Molecules, 2020, 25, 4915 CrossRef CAS PubMed.
- W. Faisal and F. Almomani, Chemosphere, 2022, 291, 133099 CrossRef CAS PubMed.
- J. Ma, M. Yao, Y. Yang and X. Zhang, J. Mol. Liq., 2022, 350, 118510 CrossRef CAS.
- S. Faizullayev, A. Adilbekova, W. Kujawski and M. Mirzaeian, J. Pet. Sci. Eng., 2022, 215, 110643 CrossRef CAS.
- S. M. Abed, N. H. Abdurahman, R. M. Yunus, H. A. Abdulbari and S. Akbari, IOP Conf. Ser.: Mater. Sci. Eng., 2019, 702, 012060 CAS.
- Z. Li, Y. Shi, A. Zhu, Y. Zhao, H. Wang, B. P. Binks and J. Wang, Angew. Chem., Int. Ed., 2021, 60, 3928–3933 CrossRef CAS PubMed.
- C. Vashisth, C. P. Whitby, D. Fornasiero and J. Ralston, J. Colloid Interface Sci., 2010, 349, 537–543 CrossRef CAS PubMed.
- J. Tang, P. J. Quinlan and K. C. Tam, Soft Matter, 2015, 11, 3512–3529 RSC.
- S. Zhang, B. S. Murray, M. Holmes, R. Ettelaie and A. Sarkar, Food Biophy., 2023, 18, 120–132 CrossRef.
- A. Sarkar, B. Murray, M. Holmes, R. Ettelaie, A. Abdalla and X. Yang, Soft Matter, 2016, 12, 3558–3569 RSC.
- M. Mahfouzi, H. Zhang, L. Haoran, D. J. McClements and M. Hadidi, Crit. Rev. Food Sci. Nutr., 2024, 1–16 CrossRef PubMed.
- A. Sarkar, S. Zhang, M. Holmes and R. Ettelaie, Adv. Colloid Interface Sci., 2019, 263, 195–211 CrossRef CAS PubMed.
- A. D. Troise, V. Fogliano and A. Madadlou, Curr. Opin. Food Sci., 2020, 31, 126–135 CrossRef.
- Z. Tai, Y. Huang, Q. Zhu, W. Wu, T. Yi, Z. Chen and Y. Lu, Drug Discovery Today, 2020, 25, 2038–2045 CrossRef CAS PubMed.
- B. P. Binks, P. D. I. Fletcher, B. L. Holt, O. Kuc, P. Beaussoubre and K. Wong, Phys. Chem. Chem. Phys., 2010, 12, 2219–2226 RSC.
- R. Tadi, B. Green, T. Curwen and P. S. Clegg, Soft Matter, 2023, 19, 9428–9434 RSC.
- C. L. G. Harman, M. A. Patel, S. Guldin and G.-L. Davies, Curr. Opin. Colloid Interface Sci., 2019, 39, 173–189 CrossRef CAS.
- A. F. Koretsky and P. M. Kruglyakov, Izv. Sib. Otd. Akad. Nauk USSR, 1971, 2, 139–140 Search PubMed.
- A. Sarkar, S. Zhang, M. Holmes and R. Ettelaie, Adv. Colloid Interface Sci., 2019, 263, 195–211 CrossRef CAS PubMed.
- R. Ettelaie and S. V. Lishchuk, Soft Matter, 2015, 11, 4251–4265 RSC.
- M. Rey, J. Kolker, J. A. Richards, I. Malhotra, T. S. Glen, N. Y. D. Li, F. H. J. Laidlaw, D. Renggli, J. Vermant, A. B. Schofield, S. Fujii, H. Löwen and P. S. Clegg, Nat. Commun., 2023, 14, 6723 CrossRef CAS PubMed.
- J. Harrer, M. Rey, S. Ciarella, H. Löwen, L. M. C. Janssen and N. Vogel, Langmuir, 2019, 35, 10512–10521 CrossRef CAS PubMed.
- B. Haney, J. G. Werner, D. A. Weitz and S. Ramakrishnan, Soft Matter, 2020, 16, 3613–3620 RSC.
- N. Aubry, P. Singh, S. Nudurupati and M. Janjua, Proceedings of the ASME Fluids Engineering Division Summer Conference, Part B, FEDSM 2008 – Jacksonville, FL, United States, 2008, pp. 1079–1088.
- K. Hwang, P. Singh and N. Aubry, Electrophoresis, 2010, 31, 850–859 CrossRef CAS PubMed.
- S. Nudurupati, M. Janjua, P. Singh and N. Aubry, Proceedings of the ASME International Mechanical Engineering Congress and Exposition 2009, IMECE 2009, American Society of Mechanical Engineers (ASME), Lake Buena Vista, FL, USA, 2009, pp. 417–426.
- J. W. Tavacoli, G. Katgert, E. G. Kim, M. E. Cates and P. S. Clegg, Phys. Rev. Lett., 2012, 108, 5 CrossRef PubMed.
- J. R. Zhang, Y. M. Li, M. T. Bao, X. L. Yang and Z. N. Wang, Environ. Sci. Technol., 2016, 50, 8809–8816 CrossRef CAS PubMed.
- S. Melle, M. Lask and G. G. Fuller, Langmuir, 2005, 21, 2158–2162 CrossRef CAS PubMed.
- E. G. Kim, K. Stratford, P. S. Clegg and M. E. Cates, Phys. Rev. E: Stat., Nonlinear, Soft Matter Phys., 2012, 85, 020403 CrossRef PubMed.
- X. Rong, R. Ettelaie, S. V. Lishchuk, H. Cheng, N. Zhao, F. Xiao, F. Cheng and H. Yang, Nat. Commun., 2019, 10, 1854 CrossRef PubMed.
- S. V. Lishchuk and R. Ettelaie, Langmuir, 2016, 32(49), 13040–13045 CrossRef CAS PubMed.
- A. Scheludko, B. V. Toshev and D. T. Bojadjiev, J. Chem. Soc., Faraday Trans. 1, 1976, 72, 2815–2828 RSC.
- A. V. Rapacchietta and A. W. Neumann, J. Colloid Interface Sci., 1977, 59, 555–567 CrossRef.
- A. D. Scheludko and D. Nikolov, Colloid Polym. Sci., 1975, 253, 396–403 CrossRef.
- J. Guzowski, M. Tasinkevych and S. Dietrich, Eur. Phys. J. E: Soft Matter Biol. Phys., 2010, 33, 219–242 CrossRef CAS PubMed.
- S. V. Lishchuk and R. Ettelaie, Soft Matter, 2020, 16, 4049–4056 RSC.
- G. B. Davies, T. Krüger, P. V. Coveney and J. Harting, J. Chem. Phys., 2014, 141, 154902 CrossRef PubMed.
- D. Daniel-David, I. Pezron, C. Dalmazzone, C. Noïk, D. Clausse and L. Komunjer, Colloids Surf., A, 2005, 270–271, 257–262 CrossRef CAS.
- W. Kang, G. Jing, H. Zhang, M. Li and Z. Wu, Colloids Surf., A, 2006, 272, 27–31 CrossRef CAS.
- D. Harbottle, Q. Chen, K. Moorthy, L. Wang, S. Xu, Q. Liu, J. Sjoblom and Z. Xu, Langmuir, 2014, 30, 6730–6738 CrossRef CAS PubMed.
- B.-Y. Kim, J. H. Moon, T.-H. Sung, S.-M. Yang and J.-D. Kim, Sep. Sci. Technol., 2002, 37, 1307–1320 CrossRef CAS.
- E. A. Elsharaky, A. S. El-Tabei and A. E. El-Tabey, ACS Omega, 2022, 7, 32471–32480 CrossRef CAS PubMed.
- Z. Li, S. An, Y. Liu, Z. Hua, F. Li, X. Wang, B. Jing and Y. Tan, ACS Omega, 2019, 4, 20697–20707 CrossRef CAS PubMed.
- W. Kang, X. Yin, H. Yang, Y. Zhao, Z. Huang, X. Hou, B. Sarsenbekuly, Z. Zhu, P. Wang, X. Zhang, J. Geng and S. Aidarova, Colloids Surf., A, 2018, 545, 197–204 CrossRef CAS.
- Z. Niu, T. Yue, X. He and R. Manica, Energy Fuels, 2019, 33, 5035–5042 CrossRef CAS.
- L. A. Pugnaloni, E. Dickinson, R. Ettelaie, A. R. Mackie and P. J. Wilde, Adv. Colloid Interface Sci., 2004, 107, 27–49 CrossRef CAS PubMed.
- G. Ren, M. Wang, L. Wang, Z. Wang, Q. Chen, Z. Xu and D. Sun, Langmuir, 2018, 34, 5798–5806 CrossRef CAS PubMed.
- Q. Tang, X. Xie, C. Li, B. Zhen, X. Cai, G. Zhang, C. Zhou and L. Wang, Colloids Surf., B, 2019, 183, 110414 CrossRef CAS PubMed.
- D. Xie, Y. Jiang, Y. Zhang and B. Song, Langmuir, 2021, 37, 5846–5853 CrossRef CAS PubMed.
- J. Jiang, S. Yu, W. Zhang, H. Zhang, Z. Cui, W. Xia and B. P. Binks, Angew. Chem., Int. Ed., 2021, 60, 11793–11798 CrossRef CAS PubMed.
- T. Zhang, Z. Wu, H. Zhu, Z. Wang, S. Sun and S. Hu, Colloids Surf., A, 2023, 657, 130548 CrossRef CAS.
- N. Manfredini, M. Merigo, J. Ilare, M. Sponchioni and D. Moscatelli, Nanoscale, 2021, 13, 8543–8554 RSC.
- X. Ren, S. He, D. Liu and Y. Zhang, J. Agric. Food Chem., 2020, 68, 3986–3994 CrossRef CAS PubMed.
- X. Ren and Y. Zhang, Colloids Surf., A, 2020, 587, 124316 CrossRef CAS.
- X. Guan, Y. Li, H. Jiang, Y.-L. S. Tse and T. Ngai, Chem. – Asian J., 2023, 18, e202300587 CrossRef CAS PubMed.
- R. He, S. Sun, J. Cui, M. Chi, Z. Wang and S. Hu, Phys. Chem. Chem. Phys., 2023, 25, 25780–25788 RSC.
- M. Lv, L. Wang, R. Huang, Y. Sun, X. Liu, W. Zhou and J. Wang, Eur. Polym. J., 2023, 183, 111697 CrossRef CAS.
- X. Zhao, B. Huang, M. El-Aooiti and D. Rousseau, J. Colloid Interface Sci., 2018, 509, 360–368 CrossRef CAS PubMed.
- M. El-Aooiti, A. de Vries and D. Rousseau, J. Colloid Interface Sci., 2023, 636, 637–645 CrossRef CAS PubMed.
- Q. Mao, M. Li, S. Zhang, X. Zhang, G. He and W. Zhang, Int. J. Biol. Macromol., 2020, 162, 1888–1896 CrossRef CAS PubMed.
- H.-P. Lim, S.-S. D. Ng, D. B. Dasa, S. A. Adnan, B.-T. Tey, E.-S. Chan, K.-W. Ho and C.-W. Ooi, Int. J. Biol. Macromol., 2023, 232, 123461 CrossRef CAS PubMed.
- W. Zhou, R. Zhang, Z. Cai, F. Wu, Y. Hu, C. Huang, K. Hu and Y. Chen, Food Chem., 2024, 437, 137916 CrossRef CAS PubMed.
- H. Xie, W. Zhao, X. Zhang and Z. Wang, ACS Appl. Mater. Interfaces, 2022, 14, 24102–24112 CrossRef CAS PubMed.
- D. Xie, Y. Jiang, K. Li, X. Yang and Y. Zhang, ACS Omega, 2022, 7, 29153–29160 CrossRef CAS PubMed.
- S. A. Deshmukh, S. K. R. S. Sankaranarayanan, K. Suthar and D. C. Mancini, J. Phys. Chem. B, 2012, 116, 2651–2663 CrossRef CAS PubMed.
- M. Heskins and J. E. Guillet, J. Macromol. Sci., Part A: Pure Appl. Chem., 1968, 2, 1441–1455 CrossRef CAS.
- S. Colombié, A. Gaunand and B. Lindet, Enzyme Microb. Technol., 2001, 28, 820–826 CrossRef PubMed.
- T. P. Santos, P. K. Okuro and R. L. Cunha, Food Hydrocolloids, 2021, 116, 106645 CrossRef CAS.
- X. Guo, X. Li, L. Chan, W. Huang and T. Chen, J. Nanobiotechnol., 2021, 19, 67 CrossRef CAS PubMed.
- V. Maingret, C. Chartier, J.-L. Six, V. Schmitt and V. Héroguez, Carbohydr. Polym., 2022, 284, 119146 CrossRef CAS PubMed.
- Q. M. Zhang, Q. F. Zhao, B. B. Zhu, R. Chen, Y. T. Zhou, X. P. Pei, H. Zhou, H. Y. An, Y. Tan and C. S. Chen, Int. J. Biol. Macromol., 2023, 244, 125393 CrossRef CAS PubMed.
- V. Maingret, C. Courrégelongue, V. Schmitt and V. Héroguez, Biomacromolecules, 2020, 21, 5358–5368 CrossRef CAS PubMed.
- Y. Xin, Z. Liu, C. Yang, C. Dong, F. Chen and K. Liu, Int. J. Biol. Macromol., 2023, 253, 127064 CrossRef CAS PubMed.
- L. Zhou, Y. Liu, Y. Li, W. Zhou, S. Peng, X. Qin, G. Liu and H. Zhang, Food Hydrocolloids, 2024, 151, 109858 CrossRef CAS.
- H. Hu, Y. Wang and X. Lu, Food Chem., 2024, 432, 137174 CrossRef CAS PubMed.
- L. Ye, H. Hu, Y. Wang, Z. Cai, W. Yu and X. Lu, J. Sci. Food Agric., 2024, 104, 5064–5076 CrossRef CAS PubMed.
- J. Yang, B. Zhu, K. Lu, J. Dou, Y. Ning, H. Wang, Y. Li, B. Qi and L. Jiang, Food Res. Int., 2023, 169, 112844 CrossRef CAS PubMed.
- Y. Shi, F. Ye, Y. Zhu and M. Miao, Food Chem., 2022, 385, 132626 CrossRef CAS PubMed.
- R. Zolfaghari, A. Fakhru’l-Razi, L. C. Abdullah, S. S. E. H. Elnashaie and A. Pendashteh, Sep. Purif. Technol., 2016, 170, 377–407 CrossRef CAS.
- A. Duconseille, T. Astruc, N. Quintana, F. Meersman and V. Sante-Lhoutellier, Food Hydrocolloids, 2015, 43, 360–376 CrossRef CAS.
|
This journal is © The Royal Society of Chemistry 2024 |