DOI:
10.1039/D2QI00479H
(Research Article)
Inorg. Chem. Front., 2022,
9, 2583-2593
Strategic design of a bifunctional Ag(I)-grafted NHC-MOF for efficient chemical fixation of CO2 from a dilute gas under ambient conditions†
Received
2nd March 2022
, Accepted 8th April 2022
First published on 11th April 2022
Introduction
The CO2 concentration in the environment is increasing rapidly and has reached 412 ppm in 2020 and it is continuing to increase with time resulting in various environmental consequences.1–5 Hence, there is an urgent requirement to capture and utilize carbon dioxide as a useful C1 source for the synthesis of commodity chemicals/fuels.6–9,10,11–17 In particular, the flue gases from industries and power plants have the components of CO2 (5–15%), N2 (∼80%), nitrogen oxide (NOx; 0.06–0.4%), and sulfur oxide (SOx; 0.02–0.3%) and contribute significantly to the rise in the concentration of atmospheric CO2.18 Furthermore, the capture of carbon dioxide from these flue gases having large quantities of N2 is found to be an energy-demanding process.19–21 Hence, direct utilization of flue gases/dilute gases as sources of CO2 for fixation into value-added chemicals offers potential benefits.22–24 Despite these merits, most of the catalytic systems reported so far still use high purity CO2 as a feedstock for its conversion into high-value chemicals.25–27 Therefore, the design of highly active catalytic systems for simultaneous carbon capture and functionalization from flue gases at low concentrations of CO2 (∼13%) has attracted enormous interest from researchers worldwide.28–30 Furthermore, out of the various CO2 functionalization processes known, α-alkylidene cyclic carbonate (α-aCC) production by coupling CO2 with propargylic alcohols has gained significant interest due to the potential utility of α-aCCs as commodity chemicals for the synthesis of natural products,31 polymers,32,33 polyurethanes,34etc.35 More importantly, the production of oxazolidinones, an important raw material for antibiotics by a three-component coupling of CO2 with propargylic alcohols and primary amines under eco-friendly mild conditions is of great significance.36–38 To achieve this transformation, Ag(I) based catalysts were found to be effective owing to their alkynophilicity promoting the activation of the C
C bond of alkynes.39,40 However, most of these studies on Ag(I) catalyzed functionalization of CO2 with alkynes have been carried out using pure CO2 under high pressure and/or high-temperature conditions to achieve high yield production of α-alkylidene cyclic carbonates/oxazolidinones,41–43 whereas, the catalytic systems known for the conversion of CO2 into fine chemicals using simulated flue gas/dilute CO2 are very sparse. Hence the catalysts which are active in the capture and conversion of CO2 from dilute gases at low concentrations are of significant interest. In this regard, MOFs have attracted tremendous interest owing to their modular nature which facilitates the introduction of CO2-philic and catalytically active sites for the simultaneous capture and utilization of carbon dioxide.44–52 Among the various types of MOFs utilized for the chemical fixation of carbon dioxide, N-heterocyclic carbene (NHC)-based MOFs have gained special interest because the NHC sites can act as potential anchoring sites for catalytic metal ions and also provide CO2-philicity to the framework.53–56 Here, the covalent linkage formed between the carbene site and Ag(I) will be beneficial in preventing the possible leaching of Ag(I) during the catalysis. Therefore, herein, we report the application of an NHC-based MOF as an ideal support for grafting catalytically active Ag(I) ions at the NHC sites to obtain Ag(I)@MOF-NHC which acts as an efficient bifunctional catalyst for CO2-capture and fixation with alkynes.57,58 Indeed, Ag(I)@MOF-NHC displayed recyclable CO2 uptake and excellent catalytic activity for efficient cyclization of CO2 with propargylic alcohols/primary amines to afford α-aCCs/oxazolidinones in high yields from a dilute gas (CO2
:
N2 = 13
:
87%) at RT and 1 atm pressure. Furthermore, the catalyst was highly recyclable and quite stable for multiple cycles of usage. The present work demonstrates the rational design of MOF-based heterogeneous catalysts for the highly recyclable conversion of CO2 into value-added oxazolidinones from dilute CO2 gas under mild conditions.
Results and discussion
The MOF-NHC was prepared by adopting a reported procedure with a slight modification (see the ESI†).59 The powder X-ray diffraction (PXRD) plots of the as-synthesized MOF corroborated the phase purity of the sample (Fig. 1a). As shown in Scheme 1, the NHC sites lined in the 1D channels of the MOF were applied to graft Ag(I) ions to generate an Ag(I)@MOF-NHC hybrid.
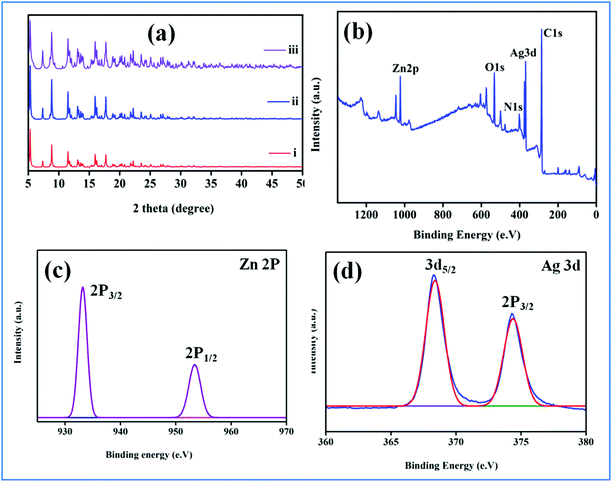 |
| Fig. 1 (a) PXRD pattern of (i) a simulated MOF, (ii) Ag(I)@MOF-NHC, and (iii) recovered Ag(I)@MOF-NHC, and (b) survey scan of Ag(I)@MOF-NHC, (c) Zn 2p, and (d) Ag 3d. | |
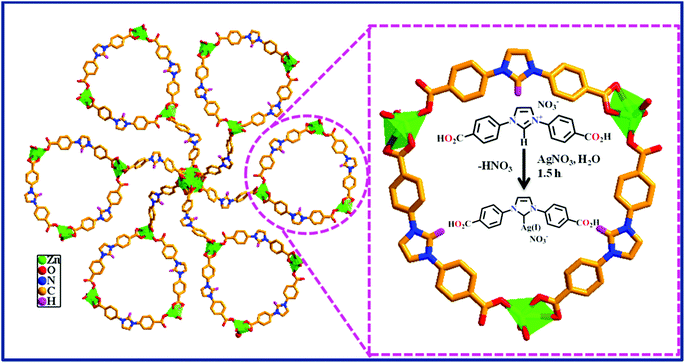 |
| Scheme 1 Schematic representation of the preparation of Ag(I)@MOF-NHC. | |
The powder X-ray diffraction (PXRD) pattern of Ag(I)@MOF-NHC was found to match well with that of the pristine MOF indicating that the framework structure is maintained even after the immobilization of Ag(I) ions at the NHC sites (Fig. 1a). Additionally, FTIR spectra showed shifting of the –C–N stretching band from 1704 cm−1 in MOF-NHC to 1179 cm−1 in Ag(I)@MOF-NHC due to the formation of –C
N+ after Ag(I) incorporation at the NHC sites (Fig. S2†). Furthermore, the UV-Vis spectra of the Ag(I) grafted sample showed absorption bands at 208 and 271 nm owing to π → π* transitions from the ligand (Fig. S3†). Furthermore, the incorporation of Ag(I) into the framework was ascertained from microwave plasma atomic emission spectroscopy (MP-AES) analysis and was found to be about 6.8% (Fig. S4†) and 22.6% NHC sites were occupied by Ag(I) ions. The XPS studies were carried out to validate the anchoring of Ag(I) at the NHC sites (Fig. 1b). The survey spectra of Ag(I)@MOF-NHC display the presence of elements Ag, Zn, C, N, and O in the sample. The Zn(II) spectra show binding energy peaks at 933.2 and 953.2 eV corresponding to 2p3/2 and 2p1/2, respectively (Fig. 1c). The Ag(I) spectra show two distinct binding energy peaks at 368.3 and 374.3 eV which are assigned to 2p3/2 and 2p1/2, respectively (Fig. 1d). It is worth noting that, these peaks were found to be slightly shifted in comparison with the corresponding peaks (368.9 and 374.9 eV) observed in AgNO3 which rule out the presence of a silver salt.31 Furthermore, the Ag(I) spectra observed here match very well with that of the Ag(I)@NHC complex (368.25 and 374.28 eV) reported in the literature60,61 which confirms the grafting of Ag(I) ions at the NHC sites. Moreover, scanning electron microscopy (SEM) images showed a rod-like morphology of MOF-NHC and notably, the morphology was maintained despite the anchoring of Ag(I) ions (Fig. S5a and S5b†). The embedding of Ag(I) in MOF-NHC was further supported by energy dispersive spectroscopy (EDS) analysis (Fig. S6a and S6b†).
Gas adsorption analysis
To ascertain the microporous nature of MOF-NHC, N2 adsorption measurements were performed for the pristine and Ag(I) doped samples at 77 K. As can be seen from Fig. 2a, the N2 adsorption isotherm showed a type-I plot with a BET surface area (SBET) of 91 m2g−1 which was found to be about 38 m2g−1 lower than that of the parent MOF-NHC (129 m2g−1). The pore size distribution measurements revealed a microporous nature with pore diameters of 10.1 and 6.6 Å for MOF-NHC and Ag(I)@MOF-NHC, respectively (Fig. S7†). This decrease in the surface area of the Ag(I) anchored sample could be ascribed to a partial loss of porosity due to the grafting of Ag(I) ions at the NHC sites. Furthermore, the CO2 sorption isotherms showed a type-1 plot with an uptake of 78/59 cm3g−1 observed at 273/298 K (Fig. 2b). By following the Freundlich–Langmuir equation (Fig. S8 and S9†) the prediction of the CO2 adsorption at saturation was determined.62 Moreover, the interaction energy (Qst) of CO2 with Ag(I)@MOF-NHC was estimated using the Clausius–Clayperon equation63 and the value was found to be 39.2 kJ mol−1 (Fig. S10†). This relatively high value of Qst represents the favorable interaction of CO2 with the basic NHC sites lined in the 1D channels of the framework.64,65 In addition, the hysteresis behavior observed in CO2 adsorption isotherms indeed supports the favorable interaction of CO2 with the NHC sites.66–68 Furthermore, a selective gas adsorption study (Fig. 2C) revealed negligible uptake of CH4 and N2 with the estimated Henry selectivity constants of 58 and 53 for CO2/CH4 and CO2/N2, respectively (Fig. S11†). Interestingly, Ag(I)@MOF-NHC demonstrated highly recyclable CO2 uptake characteristics and almost retained its adsorption capacity even after five cycles of reuse (Fig. 2d).
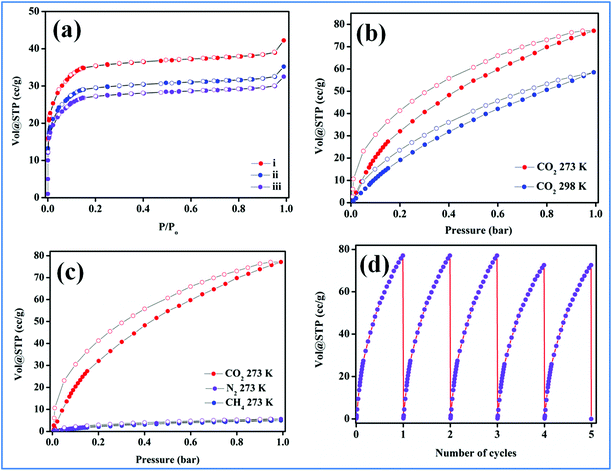 |
| Fig. 2 (a) N2 adsorption isotherm of (i) MOF-NHC, (ii) Ag(I)@MOF-NHC, and (iii) recycled Ag(I)@MOF-NHC after catalysis, (b) CO2 adsorption isotherms at 273/298 K, (c) selective CO2 adsorption plot, and (d) CO2 adsorption recyclability. | |
Catalytic utilization of CO2 at atmospheric pressure and RT
The availability of catalytically active alkynophilic Ag(I) and CO2-philic NHC sites in the 1D pores of the MOF prompted us to study the utilization of Ag(I)@MOF-NHC as a heterogeneous catalyst for the fixation of CO2 with propargylic alcohols. In this regard, 2-methyl-3-butyn-2-ol was used as a representative example for propargylic alcohols and various reaction conditions were tested by changing the catalyst and reaction time as shown in Fig. 3. As seen from Fig. 3b, the yield of the product increases with time and a slight deviation from linearity at higher reaction times could be attributed to partial masking of the catalyst surface by the substrates and products as the reaction proceeds.69–71 Under the optimized conditions, the use of 0.01 mmol of Ag(I)@MOF-NHC as a catalyst along with DBU (0.2 mmol) resulted in more than 99% conversion of 2-methyl-3-butyn-2-ol to the respective α-alkylidene cyclic carbonate with a selectivity of 100% within 6 h under atmospheric pressure (balloon) and RT conditions (Fig. S12†). Here, the role of DBU is facilitating the deprotonation of propargylic alcohol.29,72,73 Furthermore, the catalytic reaction carried out using pristine MOF-NHC as the catalyst under the optimized reaction conditions showed no formation of the product, highlighting the necessity of Ag(I) sites for catalyzing the coupling of CO2 with propargylic alcohols (Fig. 3), whereas the use of AgNO3 (homogeneous) as a catalyst rendered about 58% conversion of 2-methyl-3-butyn-2-ol under the optimized conditions (Fig. 3 and S13†). Although the homogeneous reaction resulted in the moderate conversion of the alcohol, it suffered from the limitations of catalyst recycling and product separation from the reaction mixture. The superior performance of Ag(I)@MOF-NHC over the parent samples can be ascribed to synergistic participation of alkynophilic Ag(I) and CO2-philic NHC sites projected in the 1D pores of the framework. The scope of this catalytic reaction was extended for the coupling of CO2 with various propargylic alcohols under the optimized conditions and the results obtained are summarized in Table 1 (Fig. S14–S17†). Based on the yield of α-alkylidene cyclic carbonates formed (Table 1), it can be realized that with an increase in the size of the substrates, the yield of α-aCC decreases, and this could be ascribed to the limited diffusion of the larger substrates into the pores of the framework. To further understand the effect of substrate size on the catalytic yield, the structure of the propargylic alcohols was optimized using Gaussian0974 at the B3LYP/6-311 g (d, p) level and the optimized structures are given in Table S1†. From the optimized structures, it is evident that the propargylic alcohols that are bigger underwent a lower conversion in comparison with the smaller ones. A comparison of the catalytic activity for the coupling of propargylic alcohols with CO2 revealed that Ag(I)@MOF-NHC demonstrates superior catalytic activity over various catalysts reported in the literature (Table 2).
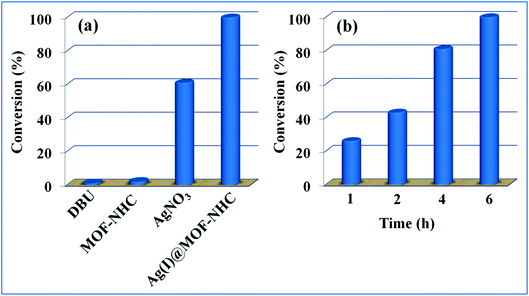 |
| Fig. 3 Catalytic optimizations by varying the catalyst (a) and reaction time (b). | |
Table 1 Catalytic utilization of CO2 under atmospheric pressure and RT conditionsa
Table 2 Comparison of the catalytic activity of Ag(I)@MOF-NHC with reported catalysts
Sl. no. |
Catalyst |
Pressure (bar) |
Time (h) |
Temperature (°C) |
Yield (%) |
Ref. |
1 |
Ag@RB-POP |
10 |
12 |
30 |
94 |
75
|
2 |
PAzo-POP-Ag |
10 |
18 |
25 |
100 |
76
|
3 |
Cu–In–MOF |
5 |
10 |
50 |
99 |
77
|
4 |
V–Cu–MOF |
4 |
10 |
25 |
99 |
78
|
5 |
AgI, IL1 |
1 |
03 |
45 |
93 |
79
|
6 |
TMOF-3-Ag |
1 |
06 |
30 |
99 |
32
|
7 |
MOF-SO3Ag |
1 |
24 |
25 |
99 |
36
|
8 |
Dy–Cu MOF |
1 |
05 |
30 |
95 |
80
|
9 |
Cu(I)@NHC-MOF |
1 |
12 |
25 |
99 |
81
|
10 |
Ag(I)@MOF-NHC |
1 |
06 |
25 |
>99 |
This work |
11 |
Ag(I)@MOF-NHC |
1 (dilute CO2) |
12 |
25 |
>99 |
This work |
Catalytic utilization of CO2 from dilute gases
The excellent performance of Ag(I)@MOF-NHC towards the utilization of CO2 (99.99%) under atmospheric pressure and RT conditions encouraged us to test its activity using dilute carbon dioxide with a composition similar to that of dry flue gas (CO2
:
N2 = 13
:
87%). To our delight, the test reaction carried out with dilute CO2 showed about 56% conversion of propargylic alcohol, 2-methyl-3-butyn-2-ol, to the respective α-alkylidene cyclic carbonate within 6 h under the optimized conditions. Furthermore, by increasing the reaction time to 12 h, the yield of the product increased to >99% with 100% selectivity (Fig. S18 and S19†). This excellent activity of Ag(I)@MOF-NHC further motivated us to extend the reaction to other propargylic alcohols under optimized conditions with dilute CO2 (13%). Interestingly, Ag(I)@MOF-NHC was able to convert a series of propargylic alcohols to the corresponding α-alkylidene cyclic carbonates with 100% selectivity (Table 3).
Table 3 Catalytic utilization of CO2 from the dilute gas (CO2
:
N2 = 13
:
87%)a
Towards exploring the potential application of our catalyst in carbon capture and utilization (CCU) from atmospheric air, the catalytic activity of Ag(I)@MOF-NHC was tested by bubbling laboratory air, and notably, about 29% of 2-methyl-3-butyn-2-ol was converted into 4,4′-dimethyl-5-methylene-1,3-dioxolan-2-one in 24 h. This observation signifies the potential application of Ag(I)@MOF-NHC toward the fixation of CO2 from direct air (Fig. S20†).
Encouraged by the high activity of Ag(I)@MOF-NHC, the substrate scope of the reaction was extended for the synthesis of oxazolidinone, a valuable commodity chemical for antibiotics, via a three-component reaction of propargylic alcohol, CO2, and primary amines. Hence, the activity of Ag(I)@MOF-NHC was studied by using propargylic alcohol, 2-methyl-3-butyn-2-ol, and the dilute gas (CO2
:
N2 = 13
:
87%) along with n-butyl amine as an example for primary amines. To our delight, the catalytic reaction resulted in the respective oxazolidinone with > 99% yield within 12 h even when using a low concentration of CO2 at 1 atm and RT (Fig. S21†). The substrate scope of the reaction was further broadened for the coupling of different primary amines such as isobutylamine, n-hexylamine, cyclohexylamine, and benzylamine (Table 4). Interestingly all of these catalytic reactions proceeded to afford the respective oxazolidinones with >99% yield (Table 4). Furthermore, to explore the generality of this process, the catalytic reactions were carried out keeping amine constant (n-butylamine) and varying the propargylic alcohols. Interestingly, these three-component reactions of alcohol, 2-ethyl-3-butyn-2-ol/3-ethyl-1-pentyn-3-ol, with CO2 and n-butylamine resulted in the respective oxazolidinones in 89/86% yield maintaining a product selectivity of 100% (Table 4). It is worth highlighting that, to the best of our knowledge, Ag(I)@MOF-NHC represents the first example of a MOF-based catalyst known for the fixation of CO2 to value-added oxazolidinones from a dilute gas containing about 13% of CO2 under RT and 1 atm (balloon) conditions.
Table 4 Catalytic fixation of CO2 into oxazolidinones from the dilute gasa
Leaching and recyclability tests
Recyclability and chemical stability are key parameters of a heterogeneous catalyst. Notably, Ag(I)@MOF-NHC was highly recyclable for several cycles with negligible loss in catalytic performance (Fig. 4a and S22†). The catalyst was recovered by centrifugation followed by filtration and utilized for subsequent catalytic cycles after washing with methanol and activating at 120 °C for 12 h. The recovered catalyst after five cycles was characterized using various techniques to affirm its crystalline phase and structural stability. The powder X-ray diffraction pattern of the recycled sample closely resembles that of the original sample, indicating its structural rigidity (Fig. 1a). Furthermore, FTIR and UV-Vis spectra of the recovered sample were similar to those of the original sample (Fig. S2 and S3†). Additionally, the N2 adsorption isotherm revealed a BET surface area of 83 m2g−1 which is slightly reduced in comparison with that of the as-synthesized sample (91 m2g−1) supporting that the porous framework structure is maintained even after five cycles of catalysis (Fig. 2a). Furthermore, the SEM analysis revealed the retention of the rod-like morphology of the sample even after the catalysis (Fig. S5c†). Importantly, the MP-AES analysis of the reaction filtrate disclosed the nonexistence of Zn(II)/Ag(I) ions which unambiguously rules out the leaching of metal ions during the catalysis (Fig. S4†).
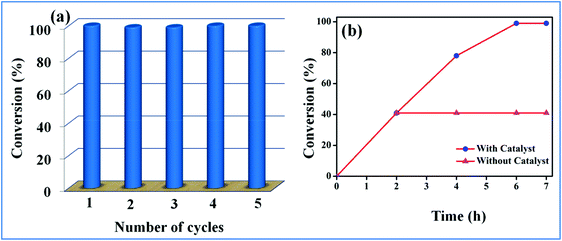 |
| Fig. 4 (a) Recyclability and (b) Catalyst leaching test. | |
Plausible mechanism
A proposed mechanism for the utilization of CO2via the carboxylation of propargylic alcohol by Ag(I)@MOF-NHC is presented in Scheme 2. The catalytic reaction proceeded with a polarization of the C
C bond of propargylic alcohol at the alkynophilic Ag(I) sites. To confirm this polarization step, both the parent and Ag(I) anchored MOFs were treated with propargylic alcohol, 2-methyl-3-butyn-2-ol, for 3 h and then the MOFs were recovered, washed with ethanol thoroughly, and dried at 80 °C for 12 h. Interestingly, the FT-IR spectra of the recovered sample of Ag(I)@MOF-NHC showed stretching bands at 2124 cm−1 corresponding to the C
C bond of 2-methyl-3-butyn-2-ol, while, no peak of the C
C bond of the alcohol was observed in the case of a pristine MOF, confirming the polarization of the π-electron of the alcohol by Ag(I) sites (Fig. S23†). This step of the alkyne bond activation is accompanied by CO2 polarization at the free NHC sites projected in the pores of the framework.82 Then the deprotonation of propargylic alcohol by DBU takes place with the concomitant CO2 insertion step. Finally, a ring-closure reaction takes place resulting in the formation of the desired product, α-alkylidene cyclic carbonate, and the subsequent elimination of the product allows the catalytic cycle to continue. Meanwhile, the addition of primary amines led to the aminolysis of α-alkylidene cyclic carbonates to generate oxazolidinones as shown in Scheme S2.†
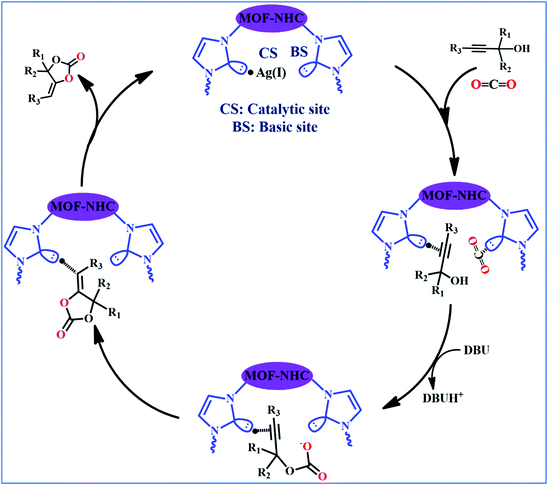 |
| Scheme 2 Proposed mechanism for the coupling of CO2 with propargylic alcohol catalyzed by Ag(1)@MOF-NHC. | |
Conclusion
A novel approach for the facile anchoring of Ag(I) ions on a basic NHC-based MOF for the rational incorporation of catalytic and CO2-philic sites suitable for simultaneous CO2 capture and conversion to high-value chemicals, oxazolidinones, from dilute CO2 gas under RT and 1 atm (balloon) conditions is presented. This study revealed that the presence of basic NHC sites enhances the framework's CO2-philicity and the local concentration of carbon dioxide at the catalytic sites for its efficient conversion into value-added chemicals. Indeed, Ag(I)@MOF-NHC exhibited excellent catalytic activity owing to the synergistic cooperation between alkynophilic Ag(I) and basic NHC sites. To the best of our knowledge, Ag(I)@MOF-NHC is the first example of an MOF-based recyclable catalyst demonstrating the conversion of CO2 from a dilute gas into value-added chemicals under the conditions of RT and 1 atm pressure. This study could pave the way for the development of efficient bifunctional catalysts for the sustainable utilization of CO2 from dilute/flue gases.
Conflicts of interest
The authors declare no conflict of interest.
Acknowledgements
C. M. N. acknowledges DST-SERB for the financial support (grant no. CRG/2018/001176). R.D. thanks IIT Ropar for the SRF and V.P. acknowledges DST-SERB for the JRF.
References
- N. M. Dowell, P. S. Fennell, N. Shah and G. C. Maitland, The role of CO2 capture and utilization in mitigating climate change, Nat. Clim. Change, 2017, 7, 243–249 CrossRef
.
- S. Basu, D. F. Baker, F. Chevallier, P. K. Patra, J. Liu and J. B. Miller, The impact of transport model differences on CO2 surface flux estimates from OCO-2 retrievals of column average CO2, Atmos. Chem. Phys., 2018, 18, 7189–7215 CrossRef CAS
.
- M. Z. Jacobson, Review of solutions to global warming, air pollution, and energy security, Energy Environ. Sci., 2009, 2, 148–173 RSC
.
- R. S. Haszeldine, Carbon Capture and Storage: How Green Can Black Be?, Science, 2009, 325, 1647–1652 CrossRef CAS PubMed
.
- R. Das, S. S. Dhankhar and C. M. Nagaraja, Construction of a bifunctional Zn(II)–organic framework containing a basic amine functionality for selective capture and room temperature fixation of CO2, Inorg. Chem. Front., 2020, 7, 72–81 RSC
.
- S. Karmakar, S. Barman, F. A. Rahimi and T. K. Maji, Covalent grafting of molecular photosensitizer and catalyst on MOF-808: effect of pore confinement toward visible light-driven CO2 reduction in water, Energy Environ. Sci., 2021, 14, 2429–2440 RSC
.
- P. Markewitz, W. Kuckshinrichs, W. Leitner, J. Linssen, P. Zapp, R. Bongartz, A. Schreibera and T. E. Müller, Worldwide innovations in the development of carbon capture technologies and the utilization of CO2, Energy Environ. Sci., 2012, 5, 7281–7305 RSC
.
- N. Sharma, S. S. Dhankhar, S. Kumar, T. J. D. Kumar and C. M. Nagaraja, Rational Design of a 3D MnII-Metal–Organic Framework Based on a Nonmetallated Porphyrin Linker for Selective Capture of CO2 and One-Pot Synthesis of Styrene Carbonates, Chem. – Eur. J., 2018, 24, 16662–16669 CrossRef CAS PubMed
.
- T. Sakakura, J. C. Choi and H. Yasuda, Transformation of Carbon Dioxide, Chem. Rev., 2007, 107, 2365–2387 CrossRef CAS PubMed
.
- H. He, J. A. Perman, G. Zhu and S. Ma, Metal-Organic Frameworks for CO2 Chemical Transformations, Small, 2016, 12, 6309–6324 CrossRef CAS PubMed
.
- S. Tiba and A. Omri, Literature survey on the relationships between energy, environment and economic growth, Renewable Sustainable Energy Rev., 2017, 69, 1129–1146 CrossRef
.
- Q. Liu, L. Wu, R. Jackstell and M. Beller, Using carbon dioxide as a building block in organic synthesis, Nat. Commun., 2015, 6, 5933 CrossRef PubMed
.
- Q.-W. Song, Z.-H. Zhou and L.-N. He, Efficient, selective and sustainable catalysis of carbon dioxide, Green Chem., 2017, 19, 3707–3728 RSC
.
- R. Das, T. Ezhil, A. S. Palakkal, D. Muthukumar, R. S. Pillai and C. M. Nagaraja, Efficient chemical fixation of CO2 from direct air under environment-friendly co-catalyst and solvent-free ambient conditions, J. Mater. Chem. A, 2021, 9, 23127–23139 RSC
.
- N. Seal, K. Karthick, M. Singh, S. Kundu and S. Neogi, Mixed-ligand-devised anionic MOF with divergent open Co(II)-nodes as chemo-resistant, bi-functional material for electrochemical water oxidation and mild-condition tandem CO2 fixation, Chem. Eng. J., 2022, 429, 132301 CrossRef CAS
.
- S. A. Rawool, R. Belgamwar, R. Jana, A. Maity, A. Bhumla, N. Yigit, A. Datta, G. Rupprechter and V. Polshettiwar, Direct CO2 capture and conversion to fuels on magnesium nanoparticles under ambient conditions simply using water, Chem. Sci., 2021, 12, 5774–5786 RSC
.
- X.-R. Tian, Y. Shi, S.-L. Hou, Y. Ma and B. Zhao, Efficient Cycloaddition of CO2 and Aziridines Activated by a Quadruple-Interpenetrated Indium–Organic Framework as a Recyclable Catalyst, Inorg. Chem., 2021, 60, 15383–15389 CrossRef CAS PubMed
.
- B. Ugale, S. S. Dhankhar and C. M. Nagaraja, Construction of 3-Fold-Interpenetrated Three-Dimensional Metal−Organic Frameworks of Nickel(II) for Highly Efficient Capture and Conversion of Carbon Dioxide, Inorg. Chem., 2016, 55, 9757–9766 CrossRef CAS PubMed
.
- R. Khalilpour, K. Mumford, H. Zhai, A. Abbas, G. Stevens and E. S. Rubin, Membrane-based carbon capture from flue gas: a review, J. Cleaner Prod., 2015, 103, 286–300 CrossRef CAS
.
- W. Lu, J. P. Sculley, D. Yuan, R. Krishna, Z. Wei and H.-C. Zhou, Polyamine-Tethered Porous Polymer Networks for Carbon Dioxide Capture from Flue Gas, Angew. Chem., Int. Ed., 2012, 51, 7480–7484 CrossRef CAS PubMed
.
- M. Wilson, S. N. Barrientos-Palomo, P. C. Stevens, N. L. Mitchell, G. Oswald, C. M. Nagaraja and J. P. S. Badyal, Substrate-Independent Epitaxial Growth of the Metal−Organic Framework MOF-508a, ACS Appl. Mater. Interfaces, 2018, 10, 4057–4065 CrossRef CAS PubMed
.
- J. Chen, H. Li, M. Zhong and Q. Yang, Hierarchical mesoporous organic polymer with an intercalated metal complex for the efficient synthesis of cyclic carbonates from flue gas, Green Chem., 2016, 18, 6493 RSC
.
- M. A. Arellano-Treviño, N. Kanani, C. W. Jeong-Potter and R. J. Farrauto, Bimetallic catalysts for CO2 capture and hydrogenation at simulated flue gas Conditions, Chem. Eng. J., 2019, 375, 121953 CrossRef
.
- T. Islamoglu, Z. Chen, M. C. Wasson, C. T. Buru, K. O. Kirlikovali, U. Afrin, M. R. Mian and O. K. Farha, Metal−Organic Frameworks against Toxic Chemicals, Chem. Rev., 2020, 120, 8130–8160 CrossRef CAS PubMed
.
- N. Sharma, S. S. Dhankhar and C. M. Nagaraja, Environment-friendly, co-catalyst- and solventfree fixation of CO2 using an ionic zinc(II)–porphyrin complex immobilized in porous metal – organic frameworks, Sustainable Energy Fuels, 2019, 3, 2977–2982 RSC
.
- B. Ugale, S. S. Dhankhar and C. M. Nagaraja, Exceptionally Stable and 20-Connected Lanthanide Metal−Organic Frameworks for Selective CO2 Capture and Conversion at Atmospheric Pressure, Cryst. Growth Des., 2018, 18, 2432–2440 CrossRef CAS
.
- B. Ugale, S. S. Dhankhar and C. M. Nagaraja, Interpenetrated Metal−Organic Frameworks of Cobalt(II): Structural Diversity, Selective Capture, and Conversion of CO2, Cryst. Growth Des., 2017, 17, 3295–3305 CrossRef CAS
.
- S.-H. Guo, X.-J. Qi, H.-M. Zhou, J. Zhou, X.-H. Wang, M. Dong, X. Zhao, C.-Y. Sun, X.-L. Wang and Z.-M. Su, A bimetallic-MOF catalyst for efficient CO2 photoreduction from simulated flue gas to valueadded formate, J. Mater. Chem. A, 2020, 8, 11712 RSC
.
- R. Ping, P. Zhao, Q. Zhang, G. Zhang, F. Liu and M. Liu, Catalytic Conversion of CO2 from Simulated Flue Gases with Aminophenol-Based Protic Ionic Liquids to Produce Quinazoline-2,4(1H,3H)-diones under Mild Conditions, ACS Sustainable Chem. Eng., 2021, 9, 5240–5249 CrossRef CAS
.
- W. Zhang, F. Ma, L. Ma, Y. Zhou and J. Wang, Imidazolium-Functionalized Ionic Hypercrosslinked Porous Polymers for Efficient Synthesis of Cyclic Carbonates from Simulated Flue Gas, ChemSusChem, 2020, 13, 341–350 CrossRef CAS PubMed
.
- R. Ninokata, T. Yamahira, G. Onodera and M. Kimura, Nickel-Catalyzed CO2 Rearrangement of Enol Metal Carbonates for the Efficient Synthesis of β-Ketocarboxylic Acids, Angew. Chem., Int. Ed., 2017, 56, 208–211 CrossRef CAS PubMed
.
- S. Fukuoka, M. Kawamura, K. Komiya, M. Tojo, H. Hachiya, K. Hasegawa, M. Aminaka, H. Okamoto, I. Fukawa and S. Konno, A novel non-phosgene polycarbonate production process using by-product CO2 as starting material, Green Chem., 2003, 5, 497–507 RSC
.
- X. B. Ding, D. P. Furkert and M. A. Brimble, General
Synthesis of the Nitropyrrolin Family of Natural Products via Regioselective CO2-Mediated Alkyne Hydration, Org. Lett., 2017, 19, 5418–5421 CrossRef CAS PubMed
.
- S. Gennen, B. Grignard, T. Tassaing, C. Jerome and C. Detrembleur, CO2-Sourced α-Alkylidene Cyclic Carbonates: A Step Forward in the Quest for Functional Regioregular Poly(urethane)s and Poly(carbonate)s, Angew. Chem., Int. Ed., 2017, 56, 10394–10398 CrossRef CAS PubMed
.
- K. Komatsuki, Y. Sadamitsu, K. Sekine, K. Saito and T. Yamada, Stereospecific Decarboxylative Nazarov Cyclization Mediated by Carbon Dioxide for the Preparation of Highly Substituted 2-Cyclopentenones, Angew. Chem., Int. Ed., 2017, 56, 11594–11598 CrossRef CAS PubMed
.
- G. Zhang, H. Yang and H. Fei, Unusual Missing Linkers in an Organosulfonate-Based Primitive- Cubic (pcu)-Type Metal-Organic Framework for CO2 Capture and Conversion under Ambient Conditions, ACS Catal., 2018, 8, 2519–2525 CrossRef CAS
.
- R. Karmakar and D. Lee, Reactions of arynes promoted by silver ions, Chem. Soc. Rev., 2016, 45, 4459–4470 RSC
.
- S. Arshadi, E. Vessally, A. Hosseinian, S. Soleimani-Amiri and L. Edjlali, Three-component coupling of CO2, propargyl alcohols, and amines: An environmentally benign access to cyclic and acyclic carbamates (A Review), J. CO2 Util., 2017, 21, 108–118 CrossRef CAS
.
- U. H. Letinois, J. M. Weibel and P. Pale, The organic chemistry of silver acetylides, Chem. Soc. Rev., 2007, 36, 759–769 RSC
.
- R. Das and C. M. Nagaraja, Highly Efficient Fixation of Carbon Dioxide at RT and Atmospheric Pressure Conditions: Influence of Polar Functionality on Selective Capture and Conversion of CO2, Inorg. Chem., 2020, 59, 9765–9773 CrossRef CAS PubMed
.
- S. Kikuchi, K. Sekine, T. Ishida and T. Yamada, C-C Bond Formation with Carbon Dioxide Promoted by a Silver Catalyst, Angew. Chem., Int. Ed., 2012, 51, 6989–6992 CrossRef CAS PubMed
.
- Q.-W. Song, B. Yu, X.-D. Li, R. Ma, Z.-F. Diao, R.-G. Li, W. Li and L.-N. He, Efficient chemical fixation of CO2 promoted by a bifunctional Ag2WO4/Ph3P system, Green Chem., 2014, 16, 1633–1638 RSC
.
- Q.-W. Song and L.-N. He, Robust Silver(I) Catalyst for the Carboxylative Cyclization of Propargylic Alcohols with Carbon Dioxide under Ambient Conditions, Adv. Synth. Catal., 2016, 358, 1251–1258 CrossRef CAS
.
- H.-C. Zhou, J. R. Long and O. M. Yaghi, Introduction to Metal–Organic Frameworks, Chem. Rev., 2012, 112, 673–674 CrossRef CAS PubMed
.
- S. S. Dhankhar, B. Ugale and C. M. Nagaraja, Co-Catalyst-Free Chemical Fixation of CO2 into Cyclic Carbonates by using Metal-Organic Frameworks as Efficient Heterogeneous Catalysts, Chem. – Asian J., 2020, 15, 2403–2427 CrossRef PubMed
.
- H.-C. Zhou and S. Kitagawa, Metal–Organic Frameworks (MOFs), Chem. Soc. Rev., 2014, 43, 5415–5418 RSC
.
- Y. Zhao, Emerging Applications of Metal-Organic Frameworks and Covalent Organic Frameworks, Chem. Mater., 2016, 28, 8079–8081 CrossRef CAS
.
- C. M. Nagaraja, R. Haldar, T. K. Maji and C. N. R. Rao, Chiral Porous Metal−Organic Frameworks of Co(II) and Ni(II): Synthesis, Structure, Magnetic Properties, and CO2 Uptake, Cryst. Growth Des., 2012, 12, 975–981 CrossRef CAS
.
- J. Liu, L. Chen, H. Cui, J. Zhang, L. Zhang and C. Y. Su, Applications of metal–organic frameworks in heterogeneous supramolecular catalysis, Chem. Soc. Rev., 2014, 43, 6011–6061 RSC
.
- A. Corma, H. García and F. X. L. Xamena, Engineering Metal Organic Frameworks for Heterogeneous Catalysis, Chem. Rev., 2010, 110, 4606–4655 CrossRef CAS PubMed
.
- R. Das, D. Muthukumar, R. S. Pillai and C. M. Nagaraja, Rational Design of a ZnII MOF with Multiple Functional Sites for Highly Efficient Fixation of CO2 under Mild Conditions: Combined Experimental and Theoretical Investigation, Chem. – Eur. J., 2020, 26, 17445–17454 CrossRef CAS PubMed
.
- X. Zhang, Z. Chen, X. Liu, S. L. Hanna, X. Wang, R. Taheri-Ledari, A. Maleki, P. Li and O. K. Farha, A historical overview of the activation and porosity of metal–organic frameworks, Chem. Soc. Rev., 2020, 49, 7406–7427 RSC
.
- X. Zhang, J. Sun, G. Wei, Z. Liu, H. Yang, K. Wang and H. Fei, In Situ Generation of an N-Heterocyclic Carbene Functionalized Metal–Organic Framework by Postsynthetic Ligand Exchange: Efficient and Selective Hydrosilylation of CO2, Angew. Chem., Int. Ed., 2019, 58, 2844–2849 CrossRef CAS PubMed
.
- S. Chen, W. Li, W. Jiang, J. Yang, J. Zhu, L. Wang, H. Ou, Z. Zhuang, M. Chen, X. Sun, D. Wang and Y. Li, MOF Encapsulating N-Heterocyclic Carbene-Ligated Copper SingleAtom Site Catalyst towards Efficient Methane Electrosynthesis, Angew. Chem., 2022, 61, e20211445 Search PubMed
.
- C. Wu, F. Irshad, M. Luo, Y. Zhao, X. Ma and S. Wang, Ruthenium Complexes Immobilized on an Azolium Based Metal Organic Framework for Highly Efficient Conversion of CO2 into Formic Acid, ChemCatChem, 2019, 11, 1256–1263 CrossRef CAS
.
- Y. Jiang, X. Zhang and H. Fei, N-heterocyclic carbene-functionalized metal–organic frameworks for the chemical fixation of CO2, Dalton Trans., 2020, 49, 6548–6552 RSC
.
- M. Du, Y. Gong, C. Bu, J. Hu, Y. Zhang, C. Chen, S. Chaemchuen, Y. Yuan and F. Verpoort, An efficient and recyclable AgNO3/ionic liquid system catalyzed atmospheric CO2 utilization: Simultaneous synthesis of 2-oxazolidinones and a-hydroxyl ketones, J. Catal., 2021, 393, 70–82 CrossRef CAS
.
- C. Weetman, S. Notman and P. L. Arnold, Destruction of chemical warfare agent simulants by air and moisture stable metal NHC complexes, Dalton Trans., 2018, 47, 2568–2574 RSC
.
- S. Sen, N. N. Nair, T. Yamada, H. Kitagawa and P. K. Bharadwaj, High Proton Conductivity by a Metal-Organic Framework Incorporating Zn8O Clusters with Aligned Imidazolium Groups Decorating the Channels, J. Am. Chem. Soc., 2012, 134, 19432–19437 CrossRef CAS PubMed
.
- J. Cao, G. Xu, P. Li, M. Tao and W. Zhang, Polyacrylonitrile Fiber Supported N-Heterocyclic Carbene Ag(I) As Efficient Catalysts for Three-Component Coupling and Intramolecular 1,3-Dipolar Cycloaddition Reactions under Flow Conditions, ACS Sustainable Chem. Eng., 2017, 5, 3438–3447 CrossRef CAS
.
- N. Boysen, T. Hasselmann, S. Karle, D. Rogalla, D. Theirich, M. Winter, T. Riedl and A. Devi, An N-Heterocyclic Carbene Based Silver Precursor for Plasma-Enhanced Spatial Atomic Layer Deposition of Silver Thin Films at Atmospheric Pressure, Angew. Chem., Int. Ed., 2018, 57, 16224–16227 CrossRef CAS PubMed
.
-
R. T. Yang, Gas Separation by Adsorption Processes, Butterworth, Boston, 1997 Search PubMed
.
- H. Pan, J. A. Ritter and P. B. Balbuena, Examination of the Approximations Used in Determining the Isosteric Heat of Adsorption from the Clausius-Clapeyron Equation, Langmuir, 1998, 14, 6323–6327 CrossRef CAS
.
- M. N. Hopkinson, C. Richter, M. Schedler and F. Glorius, An overview of N-heterocyclic carbenes, Nature, 2014, 510, 485–496 CrossRef CAS PubMed
.
- L. Yang and H. Wang, Recent Advances in Carbon Dioxide Capture, Fixation, and Activation by using N-Heterocyclic Carbenes, ChemSusChem, 2014, 7, 962–998 CrossRef CAS PubMed
.
- B. Ugale, S. Kumar, T. J. D. Kumar and C. M. Nagaraja, Environmentally Friendly, Co-catalyst-Free Chemical Fixation of CO2 at Mild Conditions Using Dual-Walled Nitrogen-Rich Three Dimensional Porous Metal-Organic Frameworks, Inorg. Chem., 2019, 58, 3925–3936 CrossRef CAS PubMed
.
- C. Yue, W. Wang and F. Li, Building N-Heterocyclic Carbene into Triazine-Linked Polymer for Multiple CO2 Utilization, ChemSusChem, 2020, 13, 5996–6004 CrossRef CAS PubMed
.
- B. Ugale, S. S. Dhankhar and C. M. Nagaraja, Construction of 3D homochiral metal–organic frameworks (MOFs) of Cd(II): selective CO2 adsorption and catalytic properties for the Knoevenagel and Henry reaction, Inorg. Chem. Front., 2017, 4, 348–359 RSC
.
- R. Das, T. Ezhil and C. M. Nagaraja, Design of Bifunctional Zinc(II)−Organic Framework for Efficient Coupling of CO2 with Terminal/Internal Epoxides under Mild Conditions, Cryst. Growth Des., 2022, 22, 598–607 CrossRef CAS
.
- Y. Ye, B. Ge, X. Meng, Y. Liu, S. Wang, X. Song and Z. Liang, An yttrium-organic framework based on a hexagonal prism second building unit for luminescent sensing of antibiotics and highly effective CO2 fixation, Inorg. Chem. Front., 2022, 9, 391–400 RSC
.
- S. S. Dhankhar, R. Das, B. Ugale, R. S. Pillai and C. M. Nagaraja, Chemical Fixation of CO2 Under Solvent and Co-Catalyst-free Conditions Using a Highly Porous Two-fold Interpenetrated Cu(II)-Metal−Organic Framework, Cryst. Growth Des., 2021, 21, 1233–1241 CrossRef CAS
.
- Z.-Z. Yang, L.-N. He, Y.-N. Zhao, B. Li and B. Yu, CO2 capture and activation by superbase/polyethylene glycol and its subsequent conversion, Energy Environ. Sci., 2011, 4, 3971–3975 RSC
.
- S. Kar, A. Goeppert and G. K. Surya Prakash, Integrated CO2 Capture and Conversion to Formate and Methanol: Connecting Two Threads, Acc. Chem. Res., 2019, 52, 2892–2903 CrossRef CAS PubMed
.
-
M. J. Frisch, G. W. Trucks, H. B. Schlegel, G. E. Scuseria, M. A. Robb, J. R. Cheeseman, G. Scalmani, V. Barone, B. Mennucci and G. A. Petersson, Gaussian 09, Revision D.01, Revision B.01, Gaussian, Inc., Wallingford, CT, 2010 Search PubMed
.
- X. Yu, Z. Yang, F. Zhang, Z. Liu, P. Yang, H. Zhang, B. Yu, Y. Zhao and Z. Liu, A rose bengal-functionalized porous organic polymer for carboxylative cyclization of propargyl alcohols with CO2, Chem. Commun., 2019, 55, 12475–12478 RSC
.
- Z. Yang, B. Yu, H. Zhang, Y. Zhao, Y. Chen, Z. Ma, G. Ji, X. Gao, B. Han and Z. Liu, Metalated Mesoporous Poly(triphenylphosphine) with Azo Functionality: Efficient Catalysts for CO2 Conversion, ACS Catal., 2016, 6, 1268–1273 CrossRef CAS
.
- S.-L. Hou, J. Dong, X.-L. Jiang, Z.-H. Jiao and B. Zhao, A Noble-Metal-Free Metal-Organic Framework (MOF) Catalyst for the Highly Efficient Conversion of CO2 with Propargylic Alcohols, Angew. Chem., Int. Ed., 2019, 58, 577–581 CrossRef CAS PubMed
.
- H.-R. Tian, Z. Zhang, S.-M. Liu, T.-Y. Dang, Z. Li, Y. Lu and S.-X. Liu, A highly stable polyoxovanadate-based Cu(I)–MOF for the carboxylative cyclization of CO2 with propargylic alcohols at room temperature, Green Chem., 2020, 22, 7513–7520 RSC
.
- Y. Yuan, Y. Xie, C. Zeng, D. Song, S. Chaemchuen, C. Chen and F. Verpoort, A recyclable AgI/OAc− catalytic system for the efficient synthesis of α-alkylidene cyclic carbonates: carbon dioxide conversion at atmospheric pressure, Green Chem., 2017, 19, 2936–2940 RSC
.
- Z. Wu, X. Lan, Y. Zhang, M. Lia and G. Bai, Copper(I) iodide cluster-based lanthanide organic frameworks: synthesis and application as efficient catalysts for carboxylative cyclization of propargyl alcohols with CO2 under mild conditions, Dalton Trans., 2019, 48, 11063–11069 RSC
.
- R. Das and C. M. Nagaraja, Noble metal-free Cu(I)-anchored NHC-based MOF for highly recyclable fixation of CO2 under RT and atmospheric pressure conditions, Green Chem., 2021, 23, 5195–5204 RSC
.
- D. Yu and Y. Zhang, Copper- and copper–N-heterocyclic carbene-catalyzed C-H activating carboxylation of terminal alkynes with CO2 at ambient conditions, Proc. Natl. Acad. Sci. U. S. A., 2010, 107, 20184–20189 CrossRef CAS PubMed
.
Footnote |
† Electronic supplementary information (ESI) available: Gas adsorption–desorption isotherms, UV-Vis and FTIR spectra, FESEM images, and 1H NMR spectra of the catalytic conversions. See DOI: https://doi.org/10.1039/d2qi00479h |
|
This journal is © the Partner Organisations 2022 |