DOI:
10.1039/D4QI02015D
(Research Article)
Inorg. Chem. Front., 2024,
11, 7416-7425
Bioinspired electron carrier mediated transmembrane photocatalytic hydrogen evolution in silica colloidosomes†
Received
8th August 2024
, Accepted 18th September 2024
First published on 19th September 2024
Abstract
Inspired by the ordered processes of chloroplasts in photosynthesis involving coordinative linkages and efficient compartmentalization, a bioinspired compartmentalized photocatalytic system (BCPS) with an inorganic compartment is designed for photocatalytic hydrogen evolution with light driven electron carrier mediated cross-membrane energy transport. A typical BCPS comprises silica colloidosomes containing co-catalysts as microreactors, where positively charged N-methyl-4-cyanopyridinium (MCP+) serves as the electron carrier. The MCP+ molecules harvest energy from photoexcited g-C3N4, and transit to electrically neutral species MCP0, which can pass the colloidosome. Driven by light, the MCP0 diffuse across the membrane and transport electrons to H+ for hydrogen evolution, in which Pt particles are employed as co-catalysts. Analogous to natural photosynthesis, hydrogen evolution occurs within compartments, separating light and dark reactions, protecting the dark reaction from interference during light harvesting and minimizing potential side effects. The system enables sustained evolution of hydrogen with a higher yield than that with the control catalysis in a bulk catalyst suspension. The dramatic influence of SDS on the hydrogen evolution was revealed, and a possible mechanism of electron carrier amount buffering was proposed.
Introduction
Hydrogen, with its high combustion value and non-polluting nature, is one of the most coveted new energy sources in contemporary society. Industrial processes commonly produce hydrogen from natural gas and coal, but these methods are plagued by low raw material utilization, polluting by-products, and high costs.1,2 In the long term, renewable energy sources are considered as the ultimate solution for hydrogen production. Photocatalytic hydrogen production, which harnesses two of the most abundant resources – solar energy and water – to generate hydrogen without any pollution, is considered a potential solution to the challenges of over-utilization, limited reserves, and negative environmental impact associated with fossil fuels.3,4 Over recent years, significant efforts have been made to enhance the intrinsic activities of photocatalysts using various techniques, including morphological control,5–7 elemental doping,8,9 heterojunction creation,10,11 and defect engineering.12–14 In spite of recent progress in the development of robust photocatalysts, encompassing semiconductor biohybrids,15–17 organic semiconductors,18,19 metal–organic frameworks,20 and plasmonic nanoparticles (NPs),21,22 the exploration of photocatalytic platforms and their widespread deployment for efficient solar hydrogen production remains an understudied area.23 Beyond the inherent activity of photocatalysts, meticulous attention to diverse facets, especially the design of photocatalytic systems, is imperative for the effective enhancement of photocatalysis.
Photosynthesis, a paradigmatic light energy utilization system in nature, achieves efficient energy conversion through light reaction and dark reaction. This intricate molecular machinery of photosynthesis is dependent on the compartmentalization of chloroplasts and a complex electron transport chain. The membranes in chloroplasts serve as barriers between different environments, allowing the influx and efflux of materials and energy to be highly regulated, thereby enhancing reaction efficiency.24 The multistep electron transfer processes from Photosystem II (PS II) to Photosystem I (PS I) and from PS I to NADP+ enable the realization of high quantum yields.25 Inspired by photosynthesis, the key steps in building a bionic microreactor involve creating compartmentalization and constructing a cascade reaction. It can be achieved by constructing bionic membranes.
In the context of bionic membranes, the self-assembly of colloidal particles (e.g., SiO2 nanoparticles,26–28 Fe2O3 particles,29,30 graphene oxide,31,32 montmorillonite,33 and LAPONITE®34,35) at the oil/water interface through Pickering emulsion facilitates the formation of cell-sized colloidosomal microcompartments.36 These microcompartments can emulate biological membranes owing to their semipermeable and robust structures.37 Emulsions stabilized by colloidal particles, named colloidosomes in 2002,38 offer easily adjustable and highly controllable permeability and elasticity after crosslinking. Colloidosome bionic microreactors have found applications in constructing cascade biphasic reaction systems,2 artificial phagocytosis,39 interfacial catalysis,40,41 multicore capsules,42,43 and artificial cells.44,45 Colloidosome-built biomimetic microreactors have a multiscale spatial structure, which distributes varying functionalities among spatially distinct units, as well as regulatory networks that link the functional units and corresponding kinetic processes. It can cause non-equilibrium behaviour, which is more akin to natural systems in many ways than to artificial materials.46
In this study, inspired by photosynthesis, a bioinspired compartmentalized photocatalytic system (BCPS) with separated light harvesting and hydrogen evolution is designed by using colloidosome microcompartments to isolate the dark reaction from the light one and transporting energy across the membrane by employing electron carriers. In a typical BCPS, Pt co-catalyst-containing colloidosomes assembled from silica nanoparticles function as the microreactors for hydrogen evolution, while g-C3N4 is employed as the photocatalyst. Positively charged N-methyl-4-cyanopyridinium (MCP+) serves as the electron carrier, harvesting energy from photoexcited g-C3N4 and becoming electrically neutral (MCP0). An MCP0 transfer from the photocatalyst to Pt co-catalysts within microreactors completes the hydrogen evolution, while MCP+ can hardly pass the colloidosomes. SDS, an anionic micelle, is introduced as energy buffers and solubilizer. Similar to natural photosynthesis, the hydrogen evolution is carried out in compartments, which protect the dark reaction from interference from the light harvesting process and minimize possible side effects. Making it possible to simulate photosynthesis through inorganic membranes supports the possibility of organic life emerging within an inorganic environment.
Experimental
Chemicals and instruments
Ludox TM-40 colloidal silica (40 wt% in water) was purchased from Aldrich. Ethylenediaminetetraacetic acid disodium salt dihydrate (EDTA, 98%), iodomethane (99%), cyanopyridine (98%), tetraethyl orthosilicate (TEOS, 99.999%), tert-butyl methyl ether (MTBE, 99.9%), octadecyltrimethoxysilane (ODTMOS, 90%), sodium hydroxide (NaOH, 97%), melamine (99%), (3-aminopropyl)trimethoxysilane (APTMS, 99%), methyltrimethoxysilane (TMOS, 98%), tetraphenylporphyrin tetrasulfonic acid (TPPS, >85%), zinc acetate (99.99%) and dimethylformamide (DMF, 99.8%) were purchased from Aladdin Reagent (Shanghai) Co. Ltd. Three-(trihydroxysilyl)-1-propanesulfonic acid (TPSA, 30%–35% in water) was purchased from Gelest. Ethanol and sodium acetate anhydrous were purchased from Beijing Chemical Works. Sodium dodecyl sulphate (SDS, 99.5%) was purchased from Tianjin Guangfu Fine Chemical Research Institute. Acetic acid glacial was obtained from Tianjin Jindong Tianzheng Fine Chemical Reagent Factory. Ultra-purified water used in all experiments was obtained from a Milli-Q Millipore system.
An S-4800 scanning electron microscope coupled with an energy dispersive X-ray spectroscopy (EDX) system was used to take scanning electron microscopy (SEM) images. A JEM-1011 transmission electron microscope coupled with an EDX system was used to take transmission electron microscopy (TEM) images. An F20S-TWIN high resolution transmission electron microscope was used to perform high resolution transmission electron microscopy (HRTEM). A Shimadzu IR Affinity-1 spectrophotometer was used to acquire Fourier transform infrared (FTIR) spectra. A Shimadzu UV-2550PC UV-visible spectrophotometer was used to acquire UV-vis absorption spectra. Nuclear magnetic resonance (NMR) spectra were obtained using a Bruker 400 MHz spectrometer (Germany).
A China Education Au-light GC7920 gas chromatograph equipped with an MS-5 A column and a thermal conductivity detector was used to determine the yields of the products. X-ray photoelectron spectroscopy (XPS) was conducted on an X-ray photoelectron spectrometer (ESCALAB 250Xi) with Al Kα as the excitation source. The photoluminescence (PL) spectra were acquired in a 1 cm path length quartz cuvette with a Shimadzu RF-5301 spectrometer. Femtosecond transient absorption spectra (FTAS) were recorded using a HELIOS femtosecond transient absorption spectrometer. Quantification measurements for Pt were performed using an Agilent 700 ICP-OES.
Preparation of g-C3N4
G-C3N4 was fabricated following a reported method.47 Specifically, 3 g of melamine were positioned within a porcelain boat and heated up to 550 °C for 4 h at a controlled rate of 2.3 °C min−1 under a nitrogen atmosphere. The resulting yellow agglomerates were milled into powder.
Preparation of Pt nanoparticles
Platinum nanoparticles were synthesized through the reduction of H2PtCl6·6H2O. Specifically, polyvinyl alcohol (PVA) was introduced into an aqueous solution of H2PtCl6·6H2O (3.85 g L−1). The pH was adjusted to 8.0 using NaOH (1 M). Subsequently, the solution was diluted to a final volume of 100 mL by adding deionized water and stirring in an ice bath for one hour. Following this, reflux distillation was conducted in a 65 °C oil bath for four hours, allowing the solution to return to room temperature. Hydrogen was introduced into the liquid for 1.5 h using a hydrogen generator. The resulting Pt nanoparticle suspension was obtained through centrifugation at a speed of 20
800 rcf for a period of 2 h.
Preparation of MCP+
MCP+ was synthesized by refluxing 0.12 mol of cyanopyridine with 0.18 mol of iodomethane in 300 mL of ethanol for a duration of 6 h. The resulting yellow-orange precipitate was collected via filtration, rinsed with ether, air-dried, and subsequently recrystallized using ethanol.
Surface modification of the SiO2 nanoparticles
A suspension was formulated by subjecting 20 mL of LUDOX TM-40 to dispersion in 300 mL of ethanol and 0.20 mL of HCl through sonication for a duration of 30 min. In order to modify the surface polarity of the pristine SiO2 nanoparticles, 100 mL of ethanol, 0.20 mL of HCl, and 12 mL of ODTMOS were added to the suspension. The resultant mixture underwent reflux for a period of 10 h. Subsequent to this, the amphiphilic octadecyl group modified SiO2 nanoparticles (OD-SiO2NPs) were washed thrice with ethanol and then resuspended in ethanol for subsequent utilization.
To prepare positively charged OD-SiO2NPs, 2 g OD-SiO2NPs were added into a 20 mL water solution containing 12 mmol APTMS. The mixture was stirred in a 70 °C oil bath after adjusting the pH to 5 with NaOH (1 M). Negatively charged OD-SiO2NPs were prepared via a similar process with positively charged OD-SiO2NPs (by replacing APTMS with TPSA).
Preparation of colloidosomes
To form a homogeneous water-in-oil dispersion, 10 mg of OD-SiO2NPs was dispersed in 3 to 5 mL of MTBE, 200 μL of Pt nanoparticle preparation was added, and then subjected to ultrasonication. TMOS was added to couple the OD-SiO2NPs. The dispersion was then subjected to heating and oscillation in a 37 °C water bath for a duration exceeding 6 hours. Following this, the oil phase was replaced with 5 mL of acetic acid buffer solution (ABS), resulting in the formation of a water-in-water system. Positively charged colloidosomes and negatively charged colloidosomes were prepared using correspondingly charged OD-SiO2NPs, respectively.
Photocatalytic hydrogen generation experiments
In a typical process, the suspension was composed of 25 mg of g-C3N4, 16 mM SDS, 30 mM EDTA, prepared colloidosomes and 2 mM MCP+ in 50 mL of ABS. In this context, MCP+ acts as the electron carrier in the BCPS, EDTA serves as a sacrificial agent, ABS maintains the system pH at 5.0, and SDS prolongs the lifetime of photogenerated electrons, prevents energy loss of excited MCP0, and enhances the solubility of colloidosomes. The dispersion was achieved through 20 min of ultrasonication. To mitigate evaporation during light irradiation, a refrigeration cycle system installed beneath the reactor maintained a temperature of 20 °C. The evolution of H2 was monitored at 1 hour intervals. Sunlight simulation was accomplished using a 300 W xenon lamp, fitted with a cut-off filter (λ ≥ 420 nm).
Preparation of zinc meso-5,10,15,20-tetrakis-(4-sulfonatophenyl) porphyrin (ZnTPPS)
One gram of TPPS was dissolved ultrasonically in 100 mL of DMF with 10 times the molar amount of zinc acetate and refluxed by heating in an oil bath at 70 °C for 1 h. The solvent was removed using a rotary evaporator to get a purple solid.
Results and discussion
Characterization of the BCPS construct
The topographic organization of the BCPS is depicted in a stylized manner in Scheme 1, with the components labelled within the diagram. For clarity, Pt@OD-SiO2NPs were defined as comprising locked OD-SiO2NPs serving as the porous membrane and Pt nanoparticles acting as the co-catalyst. G-C3N4 was chosen as the photocatalyst because of its high physicochemical stability and photocatalytic activity. MCP+ was chosen as the electron carrier whose predominant one-electron-reduced states are uncharged and therefore capable of high rates of transmembrane diffusion.48,49 As a reducing mediation, the radical of MCP+ can be used to drive water or CO2 reduction in the presence of Pt nanoparticles.50 SDS acts as an energy buffer and solubilizer. Photo-excited holes were captured by EDTA to enhance the efficiency of the photocatalytic process. The specific preparation process of Pt@OD-SiO2NPs is illustrated in Fig. S1.† This structure is assembled by emulsifying a suspension of Pt nanoparticles in MTBE containing OD-SiO2NP colloidal particles, which is followed by locking the colloidal particles together and transferring them into deionized water. Before the formation of the colloidosomes, SiO2 is soaked in ODTMOS for hydrophobic treatment to enhance its stability at the oil–water interfaces. FTIR spectroscopy, as illustrated in Fig. S2,† confirms the successful functionalization of SiO2 with octadecyl groups. Notably, the absorption bands observed at 2918 cm−1 and 2850 cm−1 are attributed to the stretching vibration of –CH2–, while the band at 2956 cm−1 is ascribed to the –CH3 group in ODTMOS. TEM images of SiO2, both before and after modification, reveal minimal changes in size (22 nm) and shape, with negligible aggregation. The particles remain uniform and monodisperse, as depicted in Fig. S3.†
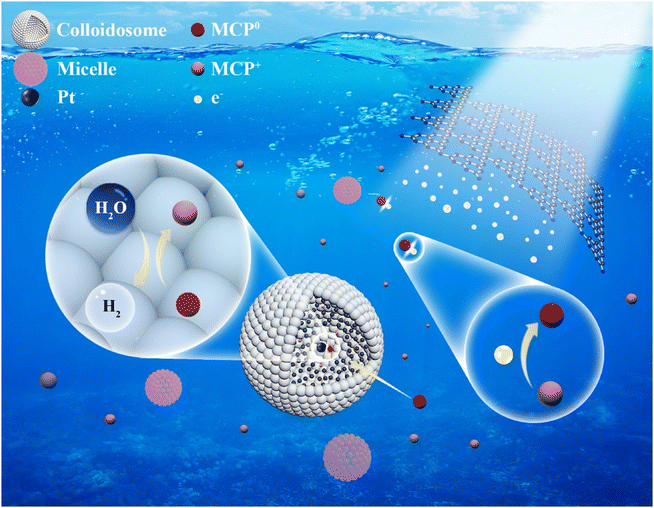 |
| Scheme 1 Reaction scheme of BCPS. | |
As a frequently utilized precious metal co-catalyst, Pt nanoparticles are encapsulated in colloidosomes. The TEM image reveals that the size of the Pt nanoparticles predominantly falls within the 3.4–6.2 nm range (Fig. S4†), which is larger than the diameter of the inner tangent sphere of the 22 nm silica particles. Furthermore, the shell of the colloidosomes, which is composed of multiple layers of colloidal particles, provides an additional barrier against the leakage of Pt nanoparticles. Any Pt leakage resulting from the rupture of colloidosomes will be removed during multiple cleaning steps. G-C3N4 was also characterized by XPS (Fig. S5†).
The surfactant-to-water ratio in emulsions stabilized by conventional surfactants plays a pivotal role in controlling droplet size and surfactant layer density. In Pickering emulsions, particles serve as the surfactant. Consequently, the ratio of OD-SiO2NP mass to liquid volume was defined as the parameter R (g mL−1) and the morphological disparities of Pt@OD-SiO2NPs with R values of 0.05 g mL−1, 0.1 g mL−1, and 0.2 g mL−1, corresponding to Fig. 1a, Fig. 1b and c, respectively, were examined. The size of the colloidosome is inversely proportional to the R-value, with a larger R-value resulting in smaller colloidosomes. With a low amount of silica particles, only a limited interfacial area can be stabilized, leading to the formation of large droplets. Conversely, a high amount of particle stabilizers facilitates the formation of very fine emulsions due to an increased interfacial area.51 As depicted in Fig. 1a–c, Pt@OD-SiO2NPs exhibited a self-supported, intact spherical structure with varying sizes at different R-values. At R = 0.05 g mL−1, the diameters of Pt@OD-SiO2NPs ranged from 5 to 6 μm, revealing significant agglomeration and rupture. At R = 0.1 g mL−1, the diameters of Pt@OD-SiO2NPs ranged from about 3 to 5 μm, shows uniformity of size and fullness of shape. At R = 0.2 g mL−1, Pt@OD-SiO2NPs also exhibit a uniform size, but with ruptures and folds 2–3 μm in size (Fig. S6†). The presence of Pt nanoparticles within the colloidosomes was characterized by SEM and HRTEM coupled with EDX (Fig. 1d and e). The Pt elemental signal observed in the SEM is a little weak due to occlusion from OD-SiO2NPs (Fig. 1e). In the Pt elemental distribution map, the strong signals around the colloidosome are attributed to background noise from the gold coating, while a strong Pt signal can be detected in the HRTEM EDX mapping (Fig. 1d), which almost completely overlaps with Si and O signals, indicating uniform distribution in Pt@OD-SiO2NPs. The concentration of Pt was found to be 115.1 mg L−1.
 |
| Fig. 1 The SEM images of colloidosomes of different sizes: (a) R = 0.05 g mL−1, (b) R = 0.1 g mL−1, and (c) R = 0.2 g mL−1. (d) The HRTEM images and corresponding elemental mapping images of Pt@SiO2 (scale bar: 1 μm). (e) The SEM images and corresponding EDX mapping images of Pt@SiO2 (scale bar: 2.5 μm). | |
Electron transfer behaviour and photocatalytic activity
The assessment of photocatalytic activity for all systems was conducted by measuring the rate of H2 generation under visible light irradiation (λ ≥ 420 nm). MCP+, a highly efficient oxidative quencher, was used as an electron carrier (Fig. S7†). Yields were determined based on the integral peak areas of starting materials and products in their respective gas chromatography graphs. To evaluate the efficiency of the BCPS, an experiment was performed without compartments of the colloidosomes (Fig. 2a). Both systems exhibit similar trends of hydrogen evolution for the initial two hours. After this stage, the hydrogen yield of BCPS surpasses that in the uncompartmentalized system, and reaches 1.2 times the control one by the end the of the four-hour experiments, about 68.61 μmol. When the reaction time was extended to 12 h, sustained hydrogen evolution was also observed after 2 h, with the advantages becoming even more pronounced (Fig. S8†). The results indicate that the BCPS significantly improves both hydrogen production and reaction sustainability compared to the uncompartmentalized control. Such findings underscore the role of the BCPS in enhancing catalyst stability and maintaining higher efficiency throughout the reaction duration. Additionally, the colloidosomes maintain their excellent morphology even after reactions, showing minimal fragmentation and preserving the integrity of the system (Fig. S9†). Control experiments without Pt nanoparticles or MCP+ were also performed to explore the mechanism of the system (Fig. 2a). The hydrogen production from the BCPS in the absence of Pt did not reach the detection limit of the instrument. The hydrogen detected from the BCPS without an electron carrier is likely due to a minor leakage of the Pt catalyst in the bulk suspension. These control systems exhibited much lower hydrogen production, underscoring the essential role of the BCPS integrity and compartmentalization in the overall process. In the absence of MCP+, hydrogen production is significantly reduced, indicating the involvement of MCP+ as an electron transfer mediator in the reaction.
 |
| Fig. 2 (a) Comparison of hydrogen production between the BCPS and BCPS lacking colloidosomes, Pt or MCP+. (b) Hydrogen production for systems with different colloidosome sizes. (c) UV-vis absorption spectra of MCP+ leakage. (d) UV-vis absorption spectra of MCP+ accumulation in colloidosomes over time. | |
Colloidosomes with different R values were prepared to explore the effect of the colloidosomes’ size on hydrogen evolution. As shown in Fig. 2b, the R = 0.1 g mL−1-sized colloidosomes demonstrate the highest hydrogen production efficiency, whereas R = 0.05 g mL−1 and R = 0.2 g ml−1-sized colloidosomes show comparatively lower efficiencies. This variation in hydrogen production efficiency can be analysed from the perspective of spatial confinement within the colloidosomes. In a confined environment, MCP0 can indeed diffuse and distribute more efficiently due to the increased likelihood of molecular collisions and interactions within a smaller space. This compact setting can enhance the frequency of contact between MCP0 and Pt nanoparticles, thereby promoting the oxidation of water and subsequently increasing hydrogen production efficiency. Additionally, the confinement effect can reduce unnecessary diffusion and loss of MCP0 within the colloidosomes, making it more effective in participating in the photocatalytic reaction. In contrast, a more spacious environment may lead to excessive dispersion and dilution of MCP0 molecules, reducing the collision frequency with Pt nanoparticles. Although this environment favors diffusion, it might lower the overall reaction efficiency due to fewer interaction opportunities, thereby decreasing the rate of water oxidation and hydrogen production efficiency. Therefore, for the distribution and efficiency of MCP0 within colloidosomes, a moderate spatial restriction typically provides the optimal balance. It minimizes diffusion limitations while maintaining a high frequency of molecular interactions, resulting in the most efficient photocatalytic hydrogen production process. To evaluate the cross-membrane capability of MCP+, it was encapsulated within colloidosomes and the supernatant was monitored hourly using UV-vis absorption spectroscopy (Fig. 2c). The results indicated minimal or no leakage over 4 hours. Only after breaking the colloidosomes by ultrasound, a significant amount of MCP+ was released. The concentration lower than that at the beginning is likely due to incomplete colloidosome disruption and MCP+ hydrophobic adsorption on colloidosomes. This suggests that MCP+ has virtually no capacity to leak out of the colloidosomes, which is consistent with previous research.52 It is because the transmembrane redox can only be achieved if the electron carriers are electroneutral. Meanwhile, UV-vis absorption spectroscopy revealed the gradual accumulation of MCP+ within Pt@SiO2 in the BCPS under irradiation (Fig. 2d), indicating that MCP0 is oxidized to MCP+ in Pt@SiO2. Given the above results, the electron acquisition and transfer process of MCP+ in the BCPS can be described as follows: initially, MCP+ receives electrons from the excited g-C3N4, transforming to MCP0. As the concentration of MCP0 increases, the concentration gradient drives the diffusion of MCP0 into Pt@SiO2, facilitating further electron transfer from MCP0 to H+ in the presence of Pt co-catalysts.
The effects of SDS and MCP+ concentrations on the BCPS
As an electron carrier, MCP+ plays a crucial role in connecting the light and dark reactions in the process. To regulate the amount of free MCP+ in solution, SDS was employed. SDS is an anionic surfactant that forms micelles above its critical micelle concentration (CMC) in solution. This study explored the effect of the molar ratio of MCP+ to SDS on hydrogen evolution, with the concentration of SDS exceeding that of CMC. As shown in Fig. 3a and c, the photocatalytic activity varies with different molar ratios of MCP+ to SDS (M/S). Compared to the SDS-free BCPS, the SDS-containing BCPS exhibits varying degrees of enhancement in hydrogen evolution. Additionally, the amount and rate of hydrogen production showed a trend of increasing and then decreasing with the rise in SDS content. A similar trend was observed in the control group with varying MCP+ contents (Fig. 3b and d), indicating that both excessively high and low concentrations of SDS or MCP+ are detrimental to catalytic efficiency. This phenomenon may be attributed to electrostatic interactions between SDS and MCP+, where the attraction of SDS to MCP+ maintains the concentration of free MCP+ in dynamic equilibrium, preventing energy dissipation due to collisions between MCP+ and MCP0. The roles of SDS and MCP+ were further investigated using FTAS and PL spectra.
 |
| Fig. 3 (a and b) Hydrogen production from the BCPS with different MCP+ and SDS ratios. (c and d) Hydrogen production rate from the BCPS with different MCP+ and SDS ratios. | |
Due to the difficulty of observing emission peaks in the FTAS of g-C3N4 aqueous dispersions, ZnTPPS was selected as the photocatalyst to elucidate the interaction between MCP+ and SDS, owing to its high energy and long lifetime.53 The structure of ZnTPPS was confirmed by 1H NMR, UV-vis absorption spectral and FTIR spectral characterization (Fig. S10†). The 1(π, π*) and 3(π, π*) excited states are distinctly observable in the time-resolved spectrum of ZnTPPS (Fig. S11†). Fig. 4a presents the transient decay curves of ZnTPPS with different components. The addition of MCP+ results in a rapid decay of ZnTPPS transient kinetics, indicating oxidative quenching of the photoexcited triplet by MCP+. In contrast, the presence of SDS prolongs the lifetime of the photogenerated electrons in ZnTPPS. Under these conditions, SDS acts as an energy buffer, stabilizing the electron transfer from ZnTPPS to MCP+. Similar results were observed in the PL spectra (Fig. 4b).
 |
| Fig. 4 (a) Transient fluorescence spectra of ZnTPPS with other components. (b) PL spectra of ZnTPPS with other components. (c) Hydrogen production in the BCPS with charged colloidosomes. (d) Mechanisms of interaction between MCP+ and SDS. (e) UV spectra of SDS when bound to colloidosomes and g-C3N4, respectively. (f) Cycling stability of BPS. | |
To further investigate the interaction between MCP+ and SDS, the surface of Pt@SiO2 was modified with amino and sulfonic acid groups to make it positively or negatively charged, respectively. Fig. 4c shows that charged Pt@SiO2 reduces hydrogen production in the BCPS, suggesting that the introduction of another charged entity interferes with the interaction between SDS and MCP+. When Pt@SiO2 is positively charged, it attracts some of the SDS, weakening the attraction between SDS micelles and MCP+, thereby diminishing the energy buffering effect of SDS. Conversely, when Pt@SiO2 is negatively charged, the concentration of free MCP+ was reduced due to the attraction of the two negatively charged entities.
Based on these results, the interaction between MCP+ and SDS can be depicted as follows (Fig. 4d). At an optimal ratio of SDS to MCP+, SDS dynamically balances the concentration of free MCP+, inhibits energy dissipation from collisions between MCP+ and MCP0, and stabilizes electron transfer, thus acting as an effective energy buffer. However, excessive MCP+ or insufficient SDS disrupts this buffering effect.
In the BCPS, SDS also plays a facilitating role in the dispersion of Pt@OD-SiO2NPs. Upon addition, SDS is partially adsorbed onto the hydrophobic chains of colloidosomes, while the remainder forms micelles in the solution. Fig. S12† visually compares the dispersion stability of SiO2 colloidosomes in deionized water, both with and without SDS micelles. The images illustrate the general dispersion of SiO2 colloidosomes in water. With the presence of SDS micelles, a notably improved stability can be observed. Importantly, the order of SDS addition does not significantly impact the dispersibility of SiO2 colloidosomes. The enhanced stability is attributed to the interactions between the surfactant and the hydrophobic end groups on the surface of OD-SiO2NPs. The interaction between anionic surfactants and hydrophobic surfaces has been documented in various studies. The introduction of SDS leads to its aggregation on the OD-SiO2NP surface due to interactions between hydrophobic ends. When concentrations exceed the critical micelle concentration (CMC), surfactant molecules cease to aggregate on the polymer and initiate micelle formation in the solution. SDS was dissolved in g-C3N4 and colloidosomes, respectively, and through sonication to ensure complete dissolution and interaction before centrifugation was employed to obtain the supernatant. As illustrated in Fig. 4e, the interaction with g-C3N4 did not significantly reduce the amount of free SDS. However, the supernatant of the colloidosomes exhibited considerably less free SDS, indicating that some SDS interacted with the hydrophobic end of the OD-SiO2NPs and was adsorbed by the colloidosomes.
Moreover, the recyclability of the BCPS was explored. The BCPS was collected by centrifugation at the end of the reaction and then washed with ABS. Then MCP+, EDTA and SDS were added to the BCPS for the next reaction cycle. As illustrated in Fig. 4f, the BCPS showed good recyclability after three reaction cycles.
Recent developments in photocatalytic microreactor systems were reviewed, revealing that the majority of these systems have been employed for photocatalytic degradation, such as dye degradation, wastewater treatment, and the breakdown of organic pollutants (Table S1†).54 In contrast, relatively few studies have utilized these systems for hydrogen production. A comparison between the limited studies on hydrogen production and our own system indicates that our system achieves significantly higher hydrogen production efficiency (Fig. S13†). These results underscore the distinctiveness of our approach and its potential to advance hydrogen production through photocatalysis.
Conclusions
In conclusion, we successfully constructed a bioinspired compartmentalized photocatalytic system (BCPS) utilizing SiO2-based colloidosome microreactors, wherein MCP+ served as the electron carrier between the active centers of the photocatalyst and co-catalyst within the microreactor. The BCPS constructs compartmentalized the cascade catalytic process. The electric carrier MCP+ transfers electrons from the photocatalyst across the membrane to H+, completing the hydrogen evolution while MCP+ can hardly pass through the colloidosomes. The presence of SDS plays a crucial role in extending the photogenerated electron lifetime and regulating the concentration of free MCP+ as energy buffer. The BCPS demonstrates a higher overall rate of hydrogen evolution and increased hydrogen production after two hours, culminating in a final yield approximately 1.2 times that of the carbon nitride uncompartmentalized system. The enhanced performance suggests the potential for simulating photosynthesis using inorganic membranes. Successfully simulating photosynthesis through inorganic membranes demonstrates the possibility of organic life emerging in an inorganic environment. Serving as an analogue of a live cell, it offers data support for devices that use directed energy transmission. This research introduces a novel approach for assembling diverse building components into a hierarchical compartment with enhanced functionality. The potential applications span various domains, including synthetic biology, biomimetics, and nanomedicine.
Author contributions
Chengkun Bai: data curation, investigation, visualization, writing – original draft and review & editing. Bingdi Wang: methodology and conceptualization and review & editing. Chunying Lv: writing – review & editing and validation. Zhengshun Jiang: writing – review & editing and validation. Zhenning Liu: conceptualization; methodology, formal analysis, writing – original draft, review & editing, and supervision. Shiyu Wang: experimental assistance. Song Liang: conceptualization; methodology, formal analysis, writing – original draft, review & editing, and supervision. Hongying Zang: conceptualization; methodology, formal analysis, writing – original draft, review & editing, and supervision.
Data availability
The data supporting this article have been included as part of the ESI.†
Conflicts of interest
There are no conflicts to declare.
Acknowledgements
The authors gratefully acknowledge the financial support from the National Key Research and Development Program of China (No. 2022YFE0138500), the National Natural Science Foundation of China (No. 22322102, 21975097, and 51975245), and the Science and Technology Development Project of Jilin Province (20240602074RC).
References
- S. Chai, G. Zhang, G. Li and Y. Zhang, Industrial hydrogen production technology and development status in China: a review, Clean Technol. Environ. Policy, 2021, 23, 1931–1946 CrossRef.
- Z. Liu, B. Wang, S. Jin, Z. Wang, L. Wang and S. Liang, Bioinspired Dual-Enzyme Colloidosome Reactors for High-Performance Biphasic Catalysis, ACS Appl. Mater. Interfaces, 2018, 10, 41504–41511 CrossRef CAS PubMed.
- D. Bahnemann, P. Robertson, C. Y. Wang, W. Choi, H. Daly, M. Danish, H. de Lasa, S. Escobedo, C. Hardacre, T. H. Jeon, B. Kim, H. Kisch, W. Li, M. C. Long, M. Muneer, N. Skillen and J. Z. Zhang, 2023 roadmap on photocatalytic water splitting, J. Phys.: Energy, 2023, 5, 28 Search PubMed.
- D. Ma, J. Chen, J. Li, X. Ji and J.-W. Shi, A review on passivation engineering for improving photocatalytic hydrogen evolution performance, J. Mater. Chem. A, 2024, 12, 12293–12324 RSC.
- Z. Tong, D. Yang, Z. Li, Y. Nan, F. Ding, Y. Shen and Z. Jiang, Thylakoid-Inspired Multishell g-C3N4 Nanocapsules with Enhanced Visible-Light Harvesting and Electron Transfer Properties for High-Efficiency Photocatalysis, ACS Nano, 2017, 11, 1103–1112 CrossRef CAS PubMed.
- M. Cargnello, T. Montini, S. Y. Smolin, J. B. Priebe, J. J. Delgado Jaén, V. V. T. Doan-Nguyen, I. S. McKay, J. A. Schwalbe, M.-M. Pohl, T. R. Gordon, Y. Lu, J. B. Baxter, A. Brückner, P. Fornasiero and C. B. Murray, Engineering titania nanostructure to tune and improve its photocatalytic activity, Proc. Natl. Acad. Sci. U. S. A., 2016, 113, 3966–3971 CrossRef CAS PubMed.
- D. Zheng, Q. Wang, Z. Pan, S. Wang, Y. Hou and G. Zhang, Poly(triazine imide) nanospheres with spatially exposed prismatic facets for photocatalytic overall water splitting, Sci. China Mater., 2024, 67, 1900–1906 CrossRef CAS.
- B. Wang, C. Bai, Z. Wang, P. She, H. Sun, G. Lu, S. Liang and Z. Liu, Phosphorus–Oxygen-Codoped Graphitic Carbon Nitride for Enhanced Hydrogen Evolution and Photocatalytic Degradation under Visible Light Irradiation, ACS Appl. Energy Mater., 2022, 5, 5774–5784 CrossRef CAS.
- Y. Q. Zhu, T. Wang, T. Xu, Y. X. Li and C. Y. Wang, Size effect of Pt co-catalyst on photocatalytic efficiency of g-C3N4 for hydrogen evolution, Appl. Surf. Sci., 2019, 464, 36–42 CrossRef CAS.
- D. Zhao, Y. Wang, C.-L. Dong, Y.-C. Huang, J. Chen, F. Xue, S. Shen and L. Guo, Boron-doped nitrogen-deficient carbon nitride-based Z-scheme heterostructures for photocatalytic overall water splitting, Nat. Energy, 2021, 6, 388–397 CrossRef CAS.
- C. Gao, T. Wei, Y. Zhang, X. Song, Y. Huan, H. Liu, M. Zhao, J. Yu and X. Chen, A Photoresponsive Rutile TiO2 Heterojunction with Enhanced Electron–Hole Separation for High-Performance Hydrogen Evolution, Adv. Mater., 2019, 31, 1806596 CrossRef PubMed.
- D. Ruan, S. Kim, M. Fujitsuka and T. Majima, Defects rich g-C3N4 with mesoporous structure for efficient photocatalytic H-2 production under visible light irradiation, Appl. Catal., B, 2018, 238, 638–646 CrossRef CAS.
- T. R. Gordon, M. Cargnello, T. Paik, F. Mangolini, R. T. Weber, P. Fornasiero and C. B. Murray, Nonaqueous Synthesis of TiO2 Nanocrystals Using TiF4 to Engineer Morphology, Oxygen Vacancy Concentration, and Photocatalytic Activity, J. Am. Chem. Soc., 2012, 134, 6751–6761 CrossRef CAS PubMed.
- Z. Zhou, W. Guo, T. Yang, D. Zheng, Y. Fang, X. Lin, Y. Hou, G. Zhang and S. Wang, Defect and nanostructure engineering of polymeric carbon nitride for visible-light-driven CO2 reduction, Chin. J. Struct. Chem., 2024, 43, 100245 CrossRef CAS.
- Y. Honda, M. Watanabe, H. Hagiwara, S. Ida and T. Ishihara, Inorganic/whole-cell biohybrid photocatalyst for highly efficient hydrogen production from water, Appl. Catal., B, 2017, 210, 400–406 CrossRef CAS.
- S. Cestellos-Blanco, H. Zhang, J. M. Kim, Y.-x. Shen and P. Yang, Photosynthetic semiconductor biohybrids for solar-driven biocatalysis, Nat. Catal., 2020, 3, 245–255 CrossRef CAS.
- J. Qin, Y. Dong, X. Lai, B. Su, B. Pan, C. Wang and S. Wang, Oxygen vacancy-rich CoMoO4/Carbon nitride S-scheme heterojunction for boosted photocatalytic H2 production: Microstructure regulation and charge transfer mechanism, J. Mater. Sci. Technol., 2024, 198, 176–185 CrossRef.
- Z. Wu, X. Wang, Y. Li, H. Zhao, J. Wang, H. Huang, Y. Liu and Z. Kang, Converting water impurity in organic solvent into hydrogen and hydrogen peroxide by organic semiconductor photocatalyst, Appl. Catal., B, 2022, 305, 121047 CrossRef CAS.
- Y. Wang, F. Silveri, M. K. Bayazit, Q. Ruan, Y. Li, J. Xie, C. R. A. Catlow and J. Tang, Bandgap Engineering of Organic Semiconductors for Highly Efficient Photocatalytic Water Splitting, Adv. Energy Mater., 2018, 8, 1801084 CrossRef.
- Y. Zhang, D. Ma, J. Li, C. Zhi, Y. Zhang, L. Liang, S. Mao and J.-W. Shi, Recent research advances of metal organic frameworks (MOFs) based composites for photocatalytic H2 evolution, Coord. Chem. Rev., 2024, 517, 215995 CrossRef CAS.
- D. Liu and C. Xue, Plasmonic Coupling Architectures for Enhanced Photocatalysis, Adv. Mater., 2021, 33, 2005738 CrossRef CAS PubMed.
- M. Lou, J. L. Bao, L. Zhou, G. N. Naidu, H. Robatjazi, A. I. Bayles, H. O. Everitt, P. Nordlander, E. A. Carter and N. J. Halas, Direct H2S Decomposition by Plasmonic Photocatalysis: Efficient Remediation plus Sustainable Hydrogen Production, ACS Energy Lett., 2022, 7, 3666–3674 CrossRef CAS.
- W. H. Lee, C. W. Lee, G. D. Cha, B.-H. Lee, J. H. Jeong, H. Park, J. Heo, M. S. Bootharaju, S.-H. Sunwoo, J. H. Kim, K. H. Ahn, D.-H. Kim and T. Hyeon, Floatable photocatalytic hydrogel nanocomposites for large-scale solar hydrogen production, Nat. Nanotechnol., 2023, 18, 754–762 CrossRef CAS PubMed.
- S. Mann, Systems of Creation: The Emergence of Life from Nonliving Matter, Acc. Chem. Res., 2012, 45, 2131–2141 CrossRef CAS PubMed.
- W. Yamori and T. Shikanai, Physiological Functions of Cyclic Electron Transport Around Photosystem I in Sustaining Photosynthesis and Plant Growth, Annu. Rev. Plant Biol., 2016, 67, 81–106 CrossRef CAS PubMed.
- H. Wu, X. Du, X. Meng, D. Qiu and Y. Qiao, A three-tiered colloidosomal microreactor for continuous flow catalysis, Nat. Commun., 2021, 12, 6113 CrossRef CAS PubMed.
- M. Kosari, U. Anjum, S. Xi, A. M. H. Lim, A. M. Seayad, E. A. J. Raj, S. M. Kozlov, A. Borgna and H. C. Zeng, Revamping SiO2 Spheres by Core–Shell Porosity Endowment to Construct a Mazelike Nanoreactor for Enhanced Catalysis in CO2 Hydrogenation to Methanol, Adv. Funct. Mater., 2021, 31, 2102896 CrossRef CAS.
- L. Rodríguez-Arco, M. Li and S. Mann, Phagocytosis-inspired behaviour in synthetic protocell communities of compartmentalized colloidal objects, Nat. Mater., 2017, 16, 857–863 CrossRef PubMed.
- A. Nicolas-Boluda, Z. Yang, T. Guilbert, L. Fouassier, F. Carn, F. Gazeau and M. P. Pileni, Self-Assemblies of Fe3O4 Nanocrystals: Toward Nanoscale Precision of Photothermal Effects in the Tumor Microenvironment, Adv. Funct. Mater., 2021, 31, 2006824 CrossRef CAS.
- H. Wang, K. Nyein Ei San, Y. Fang, Z. Xinyu and Y. Fan, Self crosslinked admicelle-Fe3O4 Janus nanoparticle with high detachment energy to creat low-energy emulsified and ultra-stable Pickering emulsion, J. Mol. Liq., 2022, 368, 120778 CrossRef CAS.
- L. Zhao, M. Shu, K. Shi, S. Tang and Z. Li, Novel use of graphene oxide quantum dots in a pickering emulsion as a Chlamydia trachomatis vaccine adjuvant, Int. Immunopharmacol., 2023, 118, 110035 CrossRef CAS PubMed.
- Q. P. Ngo, W. Yuan, Y.-C. M. Wu and T. M. Swager, Emulsion Assembly of Graphene Oxide/Polymer Composite Membranes, ACS Appl. Mater. Interfaces, 2023, 15, 21384–21393 CrossRef CAS PubMed.
- H. Chen, X. Guo, J. Li, Z. Liu, Y. Hu, X. Tao, S. Song and B. Zhu, Pickering emulsions synergistically stabilized by sugar beet pectin and montmorillonite exhibit enhanced storage stability and viscoelasticity, Int. J. Biol. Macromol., 2023, 242, 124788 CrossRef CAS PubMed.
- W. Song and A. R. Kovscek, Spontaneous clay Pickering emulsification, Colloids Surf., A, 2019, 577, 158–166 CrossRef CAS.
- D. Yu, G. Li, W. Liu, Y. Li, Z. Song, H. Wang, F. Guan and X. Chen, A fluorescent pickering-emulsion stabilizer prepared using carbon nitride quantum dots and laponite nanoparticles, Colloids Surf., A, 2019, 563, 310–317 CrossRef CAS.
- J. H. Park, A. Galanti, I. Ayling, S. Rochat, M. S. Workentin and P. Gobbo, Colloidosomes as a Protocell Model: Engineering Life-Like Behaviour through Organic Chemistry, Eur. J. Org. Chem., 2022, 2022(43), 59 CrossRef.
- Y. Han, L. Zhu, E. Karrar, X. Qi, H. Zhang and G. Wu, Pickering foams stabilized by protein-based particles: A review of characterization, stabilization, and application, Trends Food Sci. Technol., 2023, 133, 148–159 CrossRef CAS.
- A. D. Dinsmore, M. F. Hsu, M. G. Nikolaides, M. Marquez, A. R. Bausch and D. A. Weitz, Colloidosomes: Selectively Permeable Capsules Composed of Colloidal Particles, Science, 2002, 298, 1006–1009 CrossRef CAS PubMed.
- L. Rodríguez-Arco, B. V. V. S. P. Kumar, M. Li, A. J. Patil and S. Mann, Modulation of Higher-order Behaviour in Model Protocell Communities by Artificial Phagocytosis, Angew. Chem., Int. Ed., 2019, 58, 6333–6337 CrossRef PubMed.
- Z. Sun, U. Glebe, H. Charan, A. Böker and C. Wu, Enzyme–Polymer Conjugates as Robust Pickering Interfacial Biocatalysts for Efficient Biotransformations and One-Pot Cascade Reactions, Angew. Chem., Int. Ed., 2018, 57, 13810–13814 CrossRef CAS PubMed.
- F. Chang, C. M. Vis, M. Bergmeijer, S. C. Howes and P. C. A. Bruijnincx, Bifunctional Janus Silica Spheres for Pickering Interfacial Tandem Catalysis, ChemSusChem, 2021, 14, 5328–5335 CrossRef CAS PubMed.
- D. F. F. Brossault, T. M. McCoy and A. F. Routh, Preparation of Multicore Colloidosomes: Nanoparticle-Assembled Capsules with Adjustable Size, Internal Structure, and Functionalities for Oil Encapsulation, ACS Appl. Mater. Interfaces, 2021, 13, 51495–51503 CrossRef CAS PubMed.
- X. Guan, Y. Liu, Z. Wan, Y.-L. Steve Tse and T. Ngai, Non-covalent reconfigurable microgel colloidosomes with a well-defined bilayer shell, Chem. Sci., 2022, 13, 6205–6216 RSC.
- Z. Wang, M. Zhang, Y. Zhou, Y. Zhang, K. Wang and J. Liu, Coacervate Microdroplets as Synthetic Protocells for Cell Mimicking and Signaling Communications, Small Methods, 2023, 2300042 CrossRef CAS PubMed.
- W. Jiang, Z. Wu, Z. Gao, M. Wan, M. Zhou, C. Mao and J. Shen, Artificial Cells: Past, Present and Future, ACS Nano, 2022, 16, 15705–15733 CrossRef CAS PubMed.
- O. E. Shklyaev and A. C. Balazs, Interlinking spatial dimensions and kinetic processes in dissipative materials to create synthetic systems with lifelike functionality, Nat. Nanotechnol., 2024, 19, 146–159 CrossRef CAS PubMed.
- B. Wang, C. Bai, Z. Wang, P. She, H. Sun, G. Lu, S. Liang and Z. Liu, Phosphorus–Oxygen-Codoped Graphitic Carbon Nitride for Enhanced Hydrogen Evolution and Photocatalytic Degradation under Visible Light Irradiation, ACS Appl. Energy Mater., 2022, 5, 5774–5784 CrossRef CAS.
- A. Harriman, G. R. Millward, P. Neta, M. C. Richoux and J. M. Thomas, Interfacial electron-transfer reactions between platinum colloids and reducing radicals in aqueous solution, J. Phys. Chem., 1988, 92, 1286–1290 CrossRef CAS.
- R. F. Khairutdinov and J. K. Hurst, Cyclic Transmembrane Charge Transport Mediated by Pyrylium and Thiopyrylium Ions, J. Am. Chem. Soc., 2001, 123, 7352–7359 CrossRef CAS PubMed.
- H. Zhu, N. Song, H. Lv, C. L. Hill and T. Lian, Near Unity Quantum Yield of Light-Driven Redox Mediator Reduction and Efficient H2 Generation Using Colloidal Nanorod Heterostructures, J. Am. Chem. Soc., 2012, 134, 11701–11708 CrossRef CAS PubMed.
- Y. Chevalier and M.-A. Bolzinger, Emulsions stabilized with solid nanoparticles: Pickering emulsions, Colloids Surf., A, 2013, 439, 23–34 CrossRef CAS.
- S. V. Lymar, R. F. Khairutdinov, V. A. Soldatenkova and J. K. Hurst, N-Alkylcyanopyridinium-Mediated Photoinduced Charge Separation across Dihexadecyl Phosphate Vesicle Membranes, J. Phys. Chem. B, 1998, 102, 2811–2819 CrossRef CAS.
- B. C. Patterson and J. K. Hurst, Dynamics of intervesicle transfer of dihexadecyl phosphate-bound viologens, Langmuir, 1993, 9, 16–18 CrossRef CAS.
- Y. Qu, D. Sun and Y. Yu, Interfacial engineering in Pickering emulsion photocatalytic microreactors: From mechanisms to prospects, Chem. Eng. J., 2022, 438, 135655 CrossRef CAS.
|
This journal is © the Partner Organisations 2024 |
Click here to see how this site uses Cookies. View our privacy policy here.