DOI:
10.1039/D4QI00549J
(Research Article)
Inorg. Chem. Front., 2024,
11, 4721-4730
Multicolor and multimodal luminescence in an Er3+ single-doped double perovskite for advanced anti-counterfeiting and encryption†
Received
29th February 2024
, Accepted 29th May 2024
First published on 30th May 2024
Abstract
Multicolor and multimodal luminescence materials play crucial roles in anti-counterfeiting and encryption technologies due to their attractive properties of colorful, visually identifiable emissions and distinct emission modes in various excitation or stimulus channels. Nevertheless, integrating multicolor and multimodal luminescence via single rare-earth doping based on a single matrix remains a significant challenge. Herein, the Er3+ single-doped NaGdTi1.8Al0.2O6 double perovskite material achieves multicolor luminescence, afterglow, and upconversion emission by responding to several types of excitation ranging from UV to NIR and thermal disturbance. Spectral analysis has revealed that the varying excitation efficiency of the two luminescence centers at various excitation wavelengths leads to a shift in the overall emission color from red to green as the excitation wavelength transitions from 250 nm to 390 nm. Additionally, the energy transfer process between defect levels and Er3+ leads to multicolor luminescence from green to orange within a temperature range of 217 K to 417 K. These findings offer a facile and unique strategy for designing highly integrated multicolor and multimodal luminescence in a single matrix and promise applications in advanced anti-counterfeiting and information encryption technology.
1. Introduction
Advanced anti-counterfeiting strategies and technologies are invaluable in all aspects of the economic and social sphere, including the security of documents, artworks, and even private data information.1–3 Multicolor and multimode luminescence materials have received growing attention in anti-counterfeiting and encryption technologies due to their adjustable photon emissions in response to diverse external stimuli, which are generally generated via multiple dopant lanthanide ions.4–6 Nevertheless, the multi-doping strategy still suffers from the complex ionic interactions in the matrix.7–9 Meanwhile, the co-doping strategy typically requires more luminous ions, which increases the expense and complexity of the synthesis and design of the materials.10,11 In fact, the single rare-earth-doped phosphors typically exhibit mono-mode luminescence or limited emitting color.12 Hence, integrating multicolor and multimodal luminescence into a single matrix with a single lanthanide dopant remains a bottleneck issue.
Considerable efforts have been made to develop single rare-earth-doped phosphors, and various pertinent studies have attracted extensive attention. For instance, Zhang et al.13 reported combining persistent luminescence (PersL), photo-stimulated luminescence, upconversion luminescence (UCL) and photochromism in the Li2CaSiO4:Pr3+ phosphor. However, the phosphor is equipped with a monochromatic emission color. Zhou et al.14 reported the color-tunable luminescence from white to yellow by exploiting the distinct behaviors of Eu2+ ions single-doped in multiple sites of Ca6BaP4O17:Eu2+. However, the material showed a limited luminescence mode. Du et al.15 proposed a Sr2YGaO5 phosphor doped with just Sm3+, exhibiting multimodal and color-changeable luminescence (from violet to rose hermosa), but the phosphor requires constant optical excitation. The current state of research necessitates further investigation to find the appropriate matrix and dopants for effectively integrating multicolor and multimodal luminescence within a single compound doped with a single rare-earth ion.16–18 The rare-earth-doped perovskite structure has been widely employed in inorganic phosphors due to its remarkable optical characteristics, including sharp emission peaks, multi-level emission, and exceptional photochemical stability.19–22
Herein, the effective integration of multicolor and multimodal luminescence in an Er3+ single-doped double perovskite NaGdTi1.8Al0.2O6:y%Er3+ (0.1 ≤ y ≤ 2.0) (NGTAO:Er3+) was designed and synthesized through high-temperature solid state reactions, free from the use of multiple lanthanide dopants or diverse host matrices. The NGTAO:Er3+ samples with various Er3+ concentrations exhibit multicolor luminescence (red, orange, yellow and green) and red afterglow when excited by 365 nm ultraviolet (UV) light. In addition, the as-prepared NGTAO:0.1%Er3+ successfully integrated excitation-wavelength-dependent color-tunable luminescence (from red to green), external temperature-dependent color variation (from green to orange), and green UCL attributed to Er3+ under 980 nm near-infrared (NIR) excitation.23 Experimental results reveal that two luminescence centers with varying excitation efficiencies at various excitation wavelengths (250–390 nm) are the origins of the tunable excitation-wavelength-dependent multicolor luminescence. Furthermore, the energy transfer process from Er3+ to defect levels enables temperature-dependent multicolor luminescence at various temperatures (217–417 K). The designed functional anti-counterfeiting and encryption patterns can be visually read out by using simple tools (cooling–heating stimuli, UV lamps, and a 980 nm NIR laser), based on their multicolor and multimodal emission features. These findings provide a unique strategy for constructing multifunctional optical materials for anti-counterfeiting and encryption applications.
2. Experimental section
Sample preparation
NaGdTi2−xAlxO6 (0.1 ≤ x ≤ 0.5) and NGTAO:y%Er3+ (0.1 ≤ y ≤ 2.0) series of samples were prepared through a high-temperature solid-state reaction process. Na2CO3 (99.99%), Gd2O3 (99.99%), TiO2 (99.9%), and Al2O3 (99.99%) were purchased from Aladdin and used as the raw materials. A 10% excess of Na2CO3 was used to compensate for losses due to volatilization.19 Typically, a stoichiometric amount of the raw material was mixed and thoroughly ground in an agate mortar for 30 minutes, with an appropriate amount of ethanol as the milling medium. The finely milled powder was placed in a corundum crucible and subjected to annealing in a muffle furnace at 1250 °C for 4 hours. The process occurred in the atmosphere at a heating rate of 5 °C per minute during the ramp stage. Then, the resulting samples were allowed to cool down to room temperature (RT) at a rate of 5 °C min−1, followed by a fine grinding for further testing and characterization. The synthesis of pure NGTAO:y%Er3+ (0.1 ≤ y ≤ 2.0) followed the same procedure, with the only change being the addition of Er2O3 (Aladdin, 99.99%).
Materials characterization
Powder X-ray diffraction (XRD) patterns of the samples were obtained on a Rigaku D/Max-2400 X-ray diffractometer at RT. Rietveld refinement was carried out using General Structure Analysis System (GSAS) software. An FEI Tecnai F30 transmission electron microscope was used to record the results of energy dispersive spectroscopy (EDS), high-resolution transmission electron microscopy (HRTEM), and microscopic morphology. The XPS tests were carried out on a PHI-5702 instrument and the binding energy was measured using the C 1s peak (284.8 eV) as a reference. The FLS-920T fluorescence spectroradiometer was used to monitor photoluminescence excitation (PLE), photoluminescence (PL), temperature-dependent PL and UCL spectra using a Xe lamp as the excitation source. Additionally, the diffuse reflectance (DR) spectrum was recorded using a PerkinElmer LAMBDA 950. The luminescence decay measurements were conducted at 544 nm and 615 nm under 379 nm and 350 nm excitation via an FLS98. The emissions at 544 nm and 615 nm were monitored. The Vienna Ab initio Simulation Package (VASP) was employed for the spin-polarized Density Functional Theory (DFT) calculations.
3. Results and discussion
A series of NaGdTi2−xAlxO6 (0.1 ≤ x ≤ 0.5) and NGTAO:y%Er3+ (0.1 ≤ y ≤ 2.0) samples were characterized by XRD, as shown in Fig. S1† and Fig. 1a. All characteristic diffraction peaks were well matched to the standard card PDF#89-3111, revealing the successful formation of the pure phase.2 As depicted in Fig. 1a, no additional impurity peaks were detected in the NGTAO:Er3+ samples due to the small amount of doping ions and the approximately equal radii of Gd3+ (CN = 8, r = 1.053 Å) and Er3+ (CN = 8, r = 1.004 Å), suggesting the occupation of Er3+ dopants in Gd3+ sites in accordance with the Hume-Rothery rule.23 Meanwhile, the diffraction peak of NGTAO shifts in the higher angle direction compared to that of the pure NGTO sample, confirming that the larger Ti4+ (CN = 6, r = 0.605 Å) was partially replaced by the smaller Al3+ (CN = 6, r = 0.53 Å) (Fig. S1a†).24 Meanwhile, the subtle replacement of Ti4+ by Al3+ results in the shrinkage of the crystal lattice.25 To obtain more information about the structure, the XRD patterns of the sample were calculated by Rietveld refinement of NGTAO:1.0%Er3+ (Fig. 1b and Table S1†). The results show good convergence (Rwp = 13.62%, Rp = 9.66%, and χ2 = 0.69) for NGTAO and indicate that the refinement results are reliable, and the phase crystallizes in an orthorhombic perovskite structure based on the Pnma (No. 62) space group. The HRTEM reveals the single-crystalline nature of the individual particles, with a d-spacing of 2.67 Å (Fig. S1b†), corresponding to the lattice spacing along the [002] direction of NGTAO. Fig. 1c displays the crystal structure of the NGTAO matrix. Eight oxygen ions coordinate with the sites occupied by Na+/Gd3+ ions, while six oxygen ions coordinate with the sites occupied by Al3+/Ti4+ ions. This exacerbates the tilting of the [TiO6]/[AlO6] octahedra.19 Additionally, the EDS images of NGTAO:Er3+ confirm the presence of Na, Gd, Ti, Al, O and Er elements, demonstrating that all of the constituent elements are uniformly distributed within the as-prepared NGTAO:Er3+ single particle (Fig. 1d and Fig. S1c†). The XPS spectra of NGTAO:0.1% Er3+ further demonstrate that the prepared compounds are composed of Na, Gd, Ti, Al, O and Er (Fig. 1e and inset).26 A representative SEM micrograph of the NGTAO:1.0%Er3+ sample reveals a relatively regular morphology with an average size of 1–5 μm (Fig. 1f and inset).
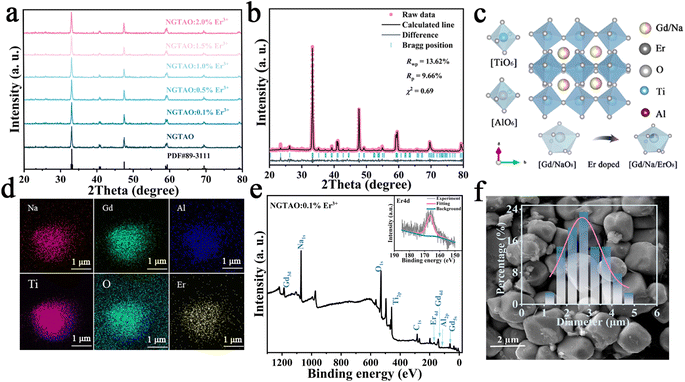 |
| Fig. 1 Morphological and structural characterization: (a) XRD patterns with different Er3+ concentrations. (b) Rietveld refinement of the powder XRD profile. Inset: calculated parameters. (c) The crystal structure of NGTAO. (d) The mapping images of the elements Na, Gd, Al, Ti, and O, respectively. (e) The XPS survey spectrum of NGTAO:0.1%Er3+. Inset: XPS spectrum of Er 4d. (f) SEM of NGTAO:yEr3+. | |
We next investigated the multicolor and multimode luminescence properties of NGTAO and NGTAO:Er3+. The normalized PLE and PL spectra of the as-obtained NGTAO:Er3+ are given in Fig. 2a. Under 350 nm excitation, the PL spectrum displays a wide emission range between 570 nm and 660 nm centered at 615 nm, which can be ascribed to the existence of oxygen-related defects, i.e. interstitial oxygen in the matrix.27–30 By monitoring the emission spectra at 615 nm, the observed PLE spectrum reveals a band peak at 350 nm, which can be attributed to the host absorption band. The shoulder band PLE spectra were monitored at 544 nm, ranging from 355 nm to 395 nm. This range corresponds to the characteristic excitation of NGTAO:Er3+, originating from the 4I15/2 → 4G11/2 and 4I15/2 → 2H9/2 transitions of Er3+.26 Importantly, the excitation regions of red and green emissions have a partial spectral overlap in the 360–395 nm range, indicating that tunable color in the red-green range of NGTAO:Er3+ can be obtained through simultaneously pumping different ratios of green/red emission by adjusting the excitation wavelength. Microstructures such as defects also play an important role in intrinsic luminescence.2 To investigate the regulating effect of Al3+ on the PL of NGT2−xAxO6, the relationship between the PL intensity of the NGT2−xAxO6 samples at different concentrations of Al3+ (x = 0, 0.1, 0.2, 0.3, 0.4, and 0.5) is shown in Fig. 2b. Interestingly, the heterovalent substitution of Al3+ for Ti4+ does not affect the positions of the red emission peaks, and the PL intensity at 615 nm in NGTAO is enhanced significantly after Al3+ is added. The addition of a small amount of Al3+ increases the asymmetry of the crystal lattice,19 leading to lattice shrinkage and ultimately enhancing the effectiveness of the energy transfer. This results in an increased luminescence intensity.25 The maximum PL intensity is observed when the Al3+ ion content is 0.2. As the content of Al3+ increases further, the distance between defects decreases (Fig. 1c), leading to the occurrence of the so-called concentration quenching effect (Fig. 2b).24,25
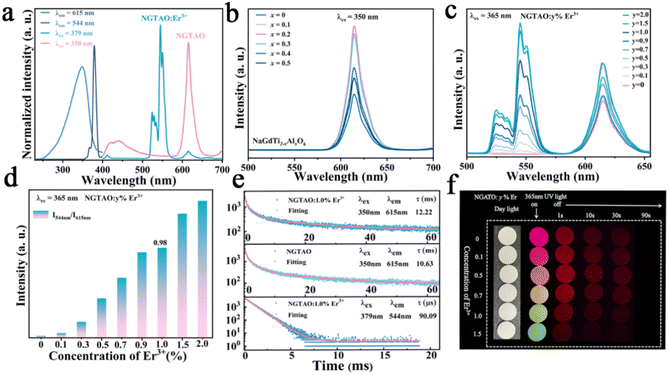 |
| Fig. 2 (a) PLE and PL spectra of NGTAO:1.0%Er3+. (b) PL spectra of NGT2−xAxO6 under 350 nm excitation. (c) PL spectra and (d) integrated intensity ratio (I544 nm/I615 nm) of NGTAO:y%Er3+ (0.1 ≤ y ≤ 2.0) under 365 nm excitation. (e) PL lifetime curves of NGTAO and NGTAO:y%Er3+ measured under 350 nm and 379 nm excitation, respectively. (f) Photos of the concentration-dependent color of NGTAO:y%Er3+ (0.1 ≤ y ≤ 2.0). | |
According to the aforementioned results, red emissions are found in NGTAO under UV light. To investigate the multicolor luminescence performance under the same excitation source, the emissions of NGTAO:y%Er3+ (0.0 ≤ y ≤ 2.0) are measured upon excitation at 365 nm and shown in Fig. 2c. As the concentration of Er3+ dopant increases, the green emission is enhanced and the red emission shows negligible change. At low concentrations of dopant, we observe that the emission at 615 nm primarily dominates the PL spectrum. The green emission at 544 nm becomes more noticeable and begins to dominate the PL as the concentration of Er3+ rises. The trend in the integrated intensity ratio (I544 nm/I615 nm) incrementally indicates that the relative contribution of emission peaks centered at 544 nm increases as the Er3+ dopant concentration increases from 0.0% to 2.0% (Fig. 2d), leading to a dopant concentration-dependent color change from red to green.
To get detailed information about the species responsible for luminescence in the NGTAO:Er3+ samples, the fluorescence decay curves corresponding to emissions from the samples were recorded. The decay curves were fitted by using the following equation:31
where
I(
t) represents the intensity at time
t,
t stands for the time,
A0 and
Ai are constants, and
τi is the characteristic lifetime. This lifetime is further used to calculate the average decay time
via the following formula:
32
Tables S2 and S3† list the derived average decay times and fitting parameters, respectively. The calculated results are presented in Fig. S2a and S2b.† As the concentration of Er3+ increases from 0.1% to 2.0%, the average decay durations for green emissions decrease (Fig. S2a†), while the red emissions exhibit an opposite trend (Fig. S2b†). This suggests enhanced non-radiative energy transfer at the higher doping levels.29 In contrast to the lifetime of green emission at 544 nm (90.09 μs), the red emission at 615 nm shows a significantly longer lifetime of 13.58 ms, as seen in Fig. 2e. This observation confirms the energy transfer from Er3+ to defect levels.26 In addition, the decay curves of red emission exhibit a long temporal tail, which correlates to the red afterglow.31,32 The photos (Fig. 2f) and CIE chromaticity coordinates (Fig. S2c†) of the NGTAO:Er3+ samples clearly show that the observed emission color shifts from red to orange, yellow and green progressively, with the concentration of Er3+ increasing from 0.0% to 2.0%. When the 365 nm irradiation is removed, the colorful luminescence disappears immediately due to the green luminescence from Er3+ vanishing, and the samples only exhibit the red afterglow (Fig. 2f). As a result, a series of multicolor-emitting phosphors were obtained through Er3+ doping concentration modulation.
Further excitation-wavelength-dependent PL and temperature-dependent PL measurements of the representative NGTAO:1.0%Er3+ sample were also carried out to obtain more insight into its luminescence properties. As expected, the emission colors of the NGTAO:1.0%Er3+ sample exhibit an obvious excitation-wavelength-dependent PL, which is obtained by integrating the green PL of Er3+ and the red emission of defect levels, as illustrated in Fig. 3a. Fig. 3b depicts the integrated luminescence intensities of I544 nm and I615 nm with the excitation wavelength range from 250 nm to 400 nm. The PL intensity of the red emission reaches a maximum at 350 nm and then decreases with the continuous increase of excitation wavelength, while the green emission is produced at 355 nm and decreases rapidly after 380 nm. The corresponding chromaticity coordinates of the wavelength-dependent PL were calculated and are shown in Fig. 3c. The PL color switched from red to green when the excitation wavelength increased from 250 nm to 390 nm, due to the shift in the relative intensity of the two different wavelengths at 615 nm and 544 nm in the PL spectrum. This shift allows for precise control over the emission color by adjusting the excitation wavelength. Fig. 3d shows the temperature-dependent luminescence behavior under 365 nm excitation of the NGTAO:1.0%Er3+ sample. The trend of temperature-dependent PL intensity in the NGTAO:1.0%Er3+ samples at various temperatures (217–417 K) is shown in Fig. 3e. It can be observed that as the temperature increased from 217 K to 377 K, the red emission intensity increased while the intensity of the green emission decreased (Fig. 3e). The energy transfer from emission centers (Er3+) to defect levels is thus confirmed again. In addition, the following slight decrease in red emission is observed as the temperature increases from 377 K to 417 K, which is attributed to the temperature quenching effect.33,34 The results indicate that NGTAO exhibits proficient intrinsic luminescence and thermal stability, making it a highly promising contender for a wide range of potential applications. The corresponding chromaticity coordinates of the temperature-dependent PL were calculated and are shown in Fig. 3f, showing that the adjustable emission colors, ranging from green to orange, were achieved at different temperatures (217–417 K). Based on the study above, the color outputs of the developed NGTAO:Er3+ samples can be easily adjusted by controlling dopant concentrations, excitation wavelengths, and external temperatures.
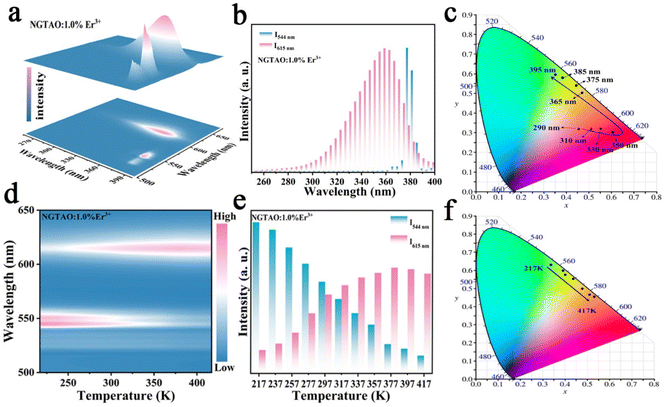 |
| Fig. 3 (a) 3D PL spectra and (b) the integrated intensity of I544 nm and I615 nm from NGTAO:1.0%Er3+ under 250–400 nm UV excitation. (c) CIE 1931 coordinates of the wavelength-dependent color of NGTAO:1.0%Er3+. (d) The temperature-dependent PL spectra and (e) the integrated luminescence intensities of I544 nm and I615 nm from NGTAO:1.0%Er3+ at different temperatures under 365 nm excitation. (f) CIE coordinates of the temperature-dependent color of NGTAO:1.0%Er3+. | |
To further investigate the UCL properties of NGTAO:Er3+, the green-emitting UCL measurements of the samples were carried out and the results are displayed in Fig. 4. The UCL spectra of NGTAO:y%Er3+ (0.1 ≤ y ≤ 2.0) present similar shapes and positions compared to those observed in the PL spectra (Fig. 4a). According to the experimental data, the 2H11/2 → 4I15/2 and 4S3/2 → 4I15/2 transitions of Er3+ are the origins of the emissions at 534 nm and 544 nm, respectively.35 The emission intensity of the two peaks rises gradually as the concentration of Er3+ increases and reaches the maximum at y = 1.5. As expected, the UCL intensity increases as the pump density increases from 0.5 to 2.0 mW cm−2, resulting in a series of progressively brighter green visible patterns (Fig. 4b). The relationship between UCL emission intensity and pump density of the NIR laser is explored according to the following equation: I ∝ Pn. Here, I refers to the UCL emission intensity, the pump density is represented by P, while n denotes the number of photons required in the upconversion process.36 The fitted slopes of the two main emissions are both close to 2, confirming the involvement of a two-photon absorption process in the UCL (Fig. 4c).35 Possible UCL mechanisms are depicted in Fig. 4d. Under 980 nm laser irradiation, the green-emitting levels 2H11/2 and 4S3/2 were populated through the 4I15/2–4I11/2 ground state absorption (GSA) and the 4I11/2–4F7/2 excited state absorption (ESA), followed by successive non-radiative relaxation from 4F7/2 to 2H11/2 and 4S3/2.36 The electrons finally return to the 4I15/2 level with intense UC emission at 534 nm and 544 nm, respectively.35
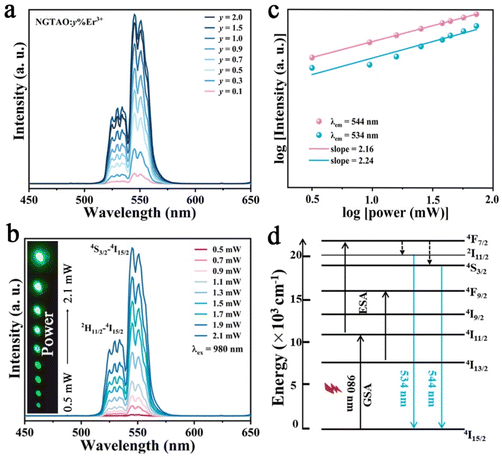 |
| Fig. 4 Dependence of the UCL spectra on the 980 nm laser power density. (a) PL spectra of NGTAO:y%Er3+ (0.1 ≤ y ≤ 2.0) under 379 nm excitation. (b) UCL spectra of NGTAO:1.0%Er3+ at different pump powers and (c) double logarithmic plots of excitation power versus UCL intensity of Er3+ at 534 nm and 544 nm for NGTAO:1.0%Er3+. (d) Energy level diagram and corresponding possible UC processes of NGTAO:Er3+. | |
Fig. 5a and the inset show the luminescence decay curves of the red emission in NGTAO:y%Er3+ (0 ≤ y ≤ 2.0) phosphors after being excited by 365 nm radiation for 5 min. The samples exhibit noticeable red afterglow for up to 200 s. It was observed that the PersL duration of the NGTAO sample was longer than that of the NGTAO:Er3+ samples. This suggests that the presence of Er3+ reduces the trapping centers compared to the NGTAO sample, resulting in a decrease in the PersL duration after doping with Er3+.36 The afterglow curve peaks corresponding to thermal energies indicate traps at different depths responsible for PersL.37 To further reveal more information about the traps, a thermoluminescence (TL) study was conducted. Generally, Urbach's empirical formula Etrap = Tm/500 can be used to calculate trap depth.37 Here, Tm represents the temperature of the peak position of the TL curve, and E denotes the trap depth. The TL curves of the NGTO, NGTAO, and NGTAO:1.0%Er3+ samples exhibit peaks at 330 K, 354 K, and 335 K after irradiation at 365 nm for 5 min, as shown in Fig. 5b. The corresponding trap depths are 0.66 eV, 0.70 eV, and 0.67 eV, respectively. For all three samples, the trap depths are smaller than 0.8, which is suitable for the PersL luminescence generation at RT.38 We observed that the presence of Al3+ caused the peak of the TL curve to shift towards higher temperatures, from 330 K to 354 K. On the other hand, the presence of Er3+ caused the peak to shift towards lower temperatures, from 354 K to 335 K. This implies that the addition of Al3+ increased the depth of the electron traps, while the addition of Er3+ had the opposite effect.26,35 The above results are consistent with the trend of the afterglow phenomenon of the corresponding compounds in Fig. 5a. A series of TL decay curves for various charge durations of NGTAO:1.0%Er3+ samples were obtained and are depicted in Fig. 5c and the corresponding trap depths were calculated as shown in Fig. 5d. The ideal continuous trap depth distribution from 0.66 eV to 0.69 eV ensures that NGTAO:1.0%Er3+ can produce long lifetime trapping carriers below the band-edge, and then yield afterglow emission.39 According to the wavelength-resolved TL spectra of NGTAO:1%Er3+ in Fig. 5e, the profile and maximum wavelength (∼615 nm) of the TL emission are in line with the PL spectra of the host in Fig. 2a, which further demonstrates that the PersL originated from matrix defect energy levels.39
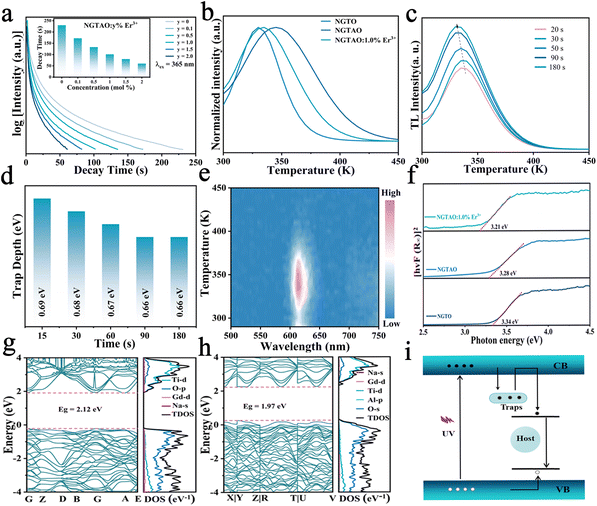 |
| Fig. 5 (a) Afterglow decay curves of NGTAO:y%Er3+ (0.1 ≤ y ≤ 2.0) after 365 nm UV irradiation. (b) TSL curve of the NGTO, NGTAO, and NGTAO:1.0%Er3+ phosphors after 365 nm excitation for 5 minutes. (c) TSL curve of the NGTAO:1.0%Er3+ phosphor at different time intervals after 5 minutes of excitation under 365 nm UV. (d) Trap depth distribution in NGTAO:1.0%Er3+. (e) Contour plot of the TL curve of the NGTAO:1.0%Er3+ after 365 nm illumination. (f) The band gap values of NGTO, NGTAO, and NGTAO:1.0%Er3+. Calculated electronic band structures and DOS of (g) NGTO and (h) NGTAO. (i) An energy diagram with a proposed plausible mechanism for the afterglow and PL mechanism. | |
The luminescence performance is directly related to the properties of the matrix. To investigate the electronic energy band structure in detail, the optical band gap (Eg) measurements and evaluation were carried out. The diffuse reflectance of the samples was measured and converted using the Kubelka–Munk function:40
and the [
F(
R∞)
hv]
2 versus
hv plots were made in accordance with the following function:
40where
R,
hv, and
A are the reflection coefficient, photon energy, and a constant, respectively, and
n = 1/2 since NGTAO is a direct semiconductor.
41 The intercept of a fitted straight line was used to calculate the band gap energy for each sample (Fig. S3a and S3b
†).
Fig. 5f shows that the fitting results of NGTO, NGTAO, and NGTAO:1.0%Er
3+ give optical band gaps of 3.34 eV, 3.28 eV, and 3.21 eV, respectively. The partial and total densities of states (DOS) of NGTO and NGTAO were calculated by DFT. The data are shown in
Fig. 5g and h, while the computational details are shown in the ESI.
† It can be observed that NGTAO possesses a direct band gap, with the maximum of the valence band (VB) and the minimum of the conduction band (CB) situated at the same location on G.
42 The predicted band gap for NGTAO was about 1.97 eV. This value was lower than the experimental value of roughly 3.34 eV, which should be attributed to the acknowledged PBE functional errors.
31 The VB is mainly dominated by the O
2p orbitals and the CB is composed mostly of the Ti
3d orbitals, as shown by the total and partial DOS of the NGTAO.
43 The above results demonstrate that the heterovalent substitution of the Al
3+ ions for Ti
4+ ions reduces the band gap and introduces trapped energy levels, increasing the conducting carriers and the absorption channel, simultaneously.
19,44 This result is in accordance with the experimental results (Fig. S3a and S3b
†). A theoretical model of the luminescence mechanism is clarified and schematically represented in
Fig. 5i based on the analysis presented above. Under UV irradiation, the electrons in the VB are promoted to the CB. The excited electrons are then captured by electron traps.
32 Some of the excited electrons relax through the nonradiative transition to the defect levels, leading to red PL emission. After the cessation of UV irradiation, the electrons stored in the traps escape into the CB by thermal vibration at RT. Subsequently, they are gradually released to the defect levels, resulting in afterglow luminescence.
The multicolored and multimodal luminescence properties of NGTAO:Er3+ phosphors offer significant potential for anti-counterfeiting and information encryption technologies. We designed a 3D printed polylactic acid device (resembling an apple tree, see Fig. 6a) based on the distinction of PL color, UCL, and different afterglow duration, filled with typical NGTAO:y%Er3+ (where y = 0.1, 0.5, 0.7, 1.0, and 1.5) samples. Under various wavelengths of irradiation in the range of 310–380 nm, the output color of the apple tree's photoluminescence pattern is extremely sensitive to the excitation wavelength. When stimulated with a 980 nm NIR laser, a bright green UCL can be achieved. Notably, under 365 nm UV lamp irradiation, the NGTAO:Er3+ phosphors exhibit excellent multicolor PL and a red afterglow. The green “tree outline” pattern is visible with four apples (red, orange, yellow, and yellowish-green) simultaneously. However, it shows a red afterglow immediately after the lamp is turned off, and their brightness gradually decreases over time. The tree outline disappeared after 10 s, and only the “red apples” pattern remains visible. When the pattern was cooled, the brightness of the “apples” decreased. While the pattern was being heated, the “apple tree” emitted a light red emission. Furthermore, multicolor and multimodal luminescence imaging of the NGTAO:Er3+ phosphors were also employed for information encryption (Fig. 6b). The NGTAO:y%Er3+ (where y = 0.1, 0.5, 0.7, and 1.0) patterns contain concealed correct information. Under the stimulation of a 980 nm NIR laser, all the interference information “green 8888” becomes visible. Under the 365 nm UV lamp, the interference information “multicolored 8888” is visible, but when the UV lamp is switched off, the correct information “red 0123” becomes visible within 20 s. As time elapses, only the correct information pattern “3” is visible and displayed after 40 s. This mode enriches the encryption dimension and improves the level of confidentiality. Consequently, the developed NGTAO:Er3+ phosphors provide exceptional potential for use in anticounterfeiting and encryption in terms of multicolor and multimodal luminescence features.
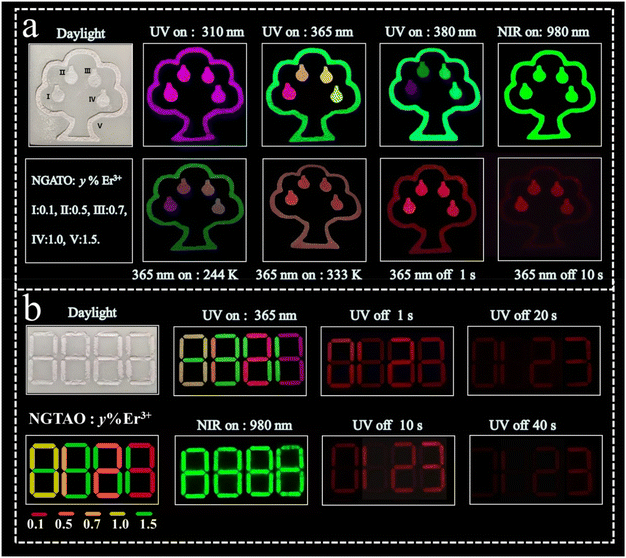 |
| Fig. 6 Applications of multicolor and multimodal luminescence features in (a) anticounterfeiting and (b) information encryption based on NGTAO:Er3+ phosphors. | |
4. Conclusion
In summary, we present a design principle for integrating multicolor and multimodal (PL, UCL, PersL) luminescence properties based on a single platform via an Er3+ single rare-earth doped double perovskite. We have demonstrated that substituting Ti4+ with Al3+ ions in NGTAO can effectively enhance the matrix emission. Parallelly, by multiplexing the emission from the host and dopant effectively, distinct emissions and multimodal luminescence properties have been achieved, avoiding the employment of multiple lanthanide ions or matrices. Thus, the tunable PL emissions can range from red to green and can be controlled simply by adjusting the doping concentration, excitation wavelength, and ambient temperature. The NGTAO:y%Er3+ (0.1 ≤ y ≤ 2.0) phosphors were successfully utilized to fabricate anti-counterfeiting and encrypted information patterns with high-level security, based on their excellent optical tunability. Finally, our study presents a facile yet efficient approach to achieve adjustable multicolor and multimode luminescence by integrating the luminescence features originating from defect levels and rare earth emission centers. Our strategy might inspire new ideas for developing optical tuning and anti-counterfeiting technology.
Conflicts of interest
The authors declare no conflict of interest.
Acknowledgements
This work was supported by the National Natural Science Foundation of China (Grant No. 21871122 and 21431002), and the Fundamental Research Funds for the Central Universities (Grant No. lzujbky-2021- kb17).
References
- J. Bai, Z. Shi and X. Jiang, “Acrostic” Encryption: Stress-Manipulation on Information Display, Adv. Funct. Mater., 2023, 33(31), 2301797 CrossRef CAS.
- X. Zhou, L. Ning, J. Qi, Y. Zha, P. Xiong and Z. Xia, Interplay of defect levels and rare earth emission centers in multimode luminescent phosphors, Nat. Commun., 2022, 13, 7589 CrossRef CAS PubMed.
- K. Huang, N. Le, J. Wang, L. Huang, L. Zeng, W. Xu, Z. Li, Y. Li and G. Han, Designing Next Generation of Persistent Luminescence: Recent Advances in Uniform Persistent Luminescence Nanoparticles, Adv. Mater., 2022, 34(14), 2107962 CrossRef CAS PubMed.
- X. Zhang, X. Hou, J. Gao, Z. Wang, X. Zhao, C. Xu and D. Gao, Colour evolution of dynamic persistent luminescence of Zn3Ga2Ge2O10:Cr3+, Mn2+phosphors for advanced anti-counterfeiting, J. Mater. Chem. C, 2023, 11, 16631–16637 RSC.
- M. Deng, Q. Liu, Y. Zhang, C. Wang, X. Guo, Z. Zhou and X. Xu, Novel Co-Doped Y2GeO5:Pr3+, Tb3+: Deep Trap Level Formation and Analog Binary Optical Storage with Submicron Information Points, Adv. Opt. Mater., 2021, 9(10), 2002090 CrossRef CAS.
- H. Sun, X. Li, Y. Zhu, X. Wang, Q. Zhang and X. Hao, Achieving multicolor emission readout and tunable photoswitching via multiplexing of dual lanthanides in ferroelectric oxides, J. Mater. Chem. C, 2019, 7, 5782–5791 RSC.
- G. Gao, D. Busko, N. Katumo, R. Joseph, E. Madirov, A. Turshatov, I. A. Howard and B. S. Richards, Ratiometric Luminescent Thermometry with Excellent Sensitivity over a Broad Temperature Range Utilizing Thermally–Assisted and Multiphoton Upconversion in Triply–Doped La2O3:Yb3+/Er3+/Nd3+, Adv. Opt. Mater., 2020, 9(5), 2001901 CrossRef.
- L. Liang, J. Chen, K. Shao, X. Qin, Z. Pan and X. Liu, Controlling persistent luminescence in nanocrystalline phosphors, Nat. Mater., 2023, 22, 289–304 CrossRef CAS PubMed.
- Q. Wu, Q. Zhang, W. Li, L. Luo and P. Du, Tailoring of visible light driven photocatalytic activities of Bi2MoO6 flower-like microspheres via synergistic effect of doping and surface Plasmon resonance, Chem. Eng. J., 2023, 475, 146192 CrossRef CAS.
- X. Zhang, H. Suo, Y. Wang, B. Chen, W. Zheng, Q. Wang, Y. Wang, Z. Zeng, S. Tsang, D. Tu and F. Wang, Systematic Tuning of Persistent Luminescence in a Quaternary Wurtzite Crystal Through Synergistic Defect Engineering, Laser Photonics Rev., 2023, 17(9), 230013 Search PubMed.
- J. Zhang, C. Pan, Y. Zhu, L. Zhao, H. He, X. Liu and J. Qiu, Achieving Thermo–Mechano–Opto–Responsive Bitemporal Colorful Luminescence via Multiplexing of Dual Lanthanides in Piezoelectric Particles and its Multidimensional Anticounterfeiting, Adv. Mater., 2018, 30(49), 1804644 CrossRef PubMed.
- H. Lv, L. Luo, W. Li and P. Du, Manipulating Upconversion Emission and Thermochromic Properties of Ho3+/Yb3+-Codoped Al2Mo3O12 Microparticles by Negative Lattice Expansion for Multimode Visual Optical Thermometry, Inorg. Chem., 2022, 61, 11442–11453 CrossRef CAS PubMed.
- Y. Zhang, X. Shan, X. Lv, D. Chen, S. Miao, W. Wang and Y. Liang, Multimodal luminescence in Pr3+ single-doped Li2CaSiO4 phosphor for optical information storage and anti-counterfeiting applications, Chem. Eng. J., 2023, 474, 145886 CrossRef CAS.
- R. Zhou, C. Liu, L. Lin, Y. Huang and H. Liang, Multi-site occupancies of Eu2+ in Ca6BaP4O17 and their potential optical thermometric applications, Chem. Eng. J., 2019, 69, 376–385 CrossRef.
- J. Du, S. Lyu, P. Wang, T. Wang and H. Lin, Multimode-responsive luminescence smart platform by single-Sm3+-doped phosphors, Adv. Opt. Mater., 2023, 11(12), 2300359 CrossRef CAS.
- D. Gao, K. Ma, P. Wang, X. Zhang, Q. Pang, H. Xin, Z. Zhang and H. Jiao, Tuning multicolour emission of Zn2GeO4:Mn phosphors by Li+ doping for information encryption and anti-counterfeiting applications, Dalton Trans., 2022, 51(2), 553–561 RSC.
- T. Zheng, M. Runowski, J. Xue, L. Luo, U. R. Rodríguez-Mendoza, V. Lavín, I. R. Martín, P. Rodríguez-Hernández, A. Muñoz and P. Du, Giant Pressure-Induced Spectral Shift in Cyan-Emitting Eu2+-Activated Sr8Si4O12Cl8 Microspheres for Ultrasensitive Visual Manometry, Adv. Funct. Mater., 2023, 33, 2214663 CrossRef CAS.
- D. Gao, Q. Kuang, F. Gao, H. Xin, S. Yun and Y. Wang, Achieving opto-responsive multimode luminescence in Zn1+xGa2–2xGexO4: Mn persistent phosphors for advanced anti-counterfeiting and information encryption, Mater. Today Phys., 2022, 27, 100765 CrossRef CAS.
- Y. Inaguma, T. Tsuchiya and T. Katsumata, Systematic study of photoluminescence upon band gap excitation in perovskite-type titanates R1/2Na1/2TiO3:Pr (R=La, Gd, Lu, and Y), J. Solid State Chem., 2007, 180(5), 1678–1685 CrossRef CAS.
- P. Zhang, W. Xie, Z. Lin, X. Huang, Y. Yang and W. Liu, Opto–Mechano–Thermo–Sensitive Allochroic Luminescence Based on Solution–Grown Halide Double Perovskite Crystal, Laser Photonics Rev., 2023, 17(12), 230026 Search PubMed.
- W. Yin, B. Weng, J. Ge, Q. Sun, Z. Li and Y. Yan, Oxide perovskites, double perovskites and derivatives for electrocatalysis, photocatalysis, and photovoltaics, Energy Environ. Sci., 2019, 12(2), 442–462 RSC.
- T. Zheng, L. Luo, P. Du, S. Lis, U. R. Rodríguez-Mendoza, V. Lavín and M. Runowski, Highly-efficient double perovskite Mn4+-activated Gd2ZnTiO6 phosphors: A bifunctional optical sensing platform for luminescence thermometry and manometry, Chem. Eng. J., 2022, 446, 136839 CrossRef CAS.
- X. Cheng, Y. Pan, Z. Yuan, X. Wang, W. Su, L. Yin, X. Xie and L. Huang, Er3+ sensitized photon upconversion nanocrystals, Adv. Funct. Mater., 2018, 28(22), 180020 CrossRef.
- W. Hume-Rothery, Some notes on the structures of alloys of iron, Contemp. Phys., 1964, 5(5), 321–347 CrossRef CAS.
- Y. Yang, Y. Shi, J. Duan, K. Lu, G. Cheng, Y. Zhao, Z. Huang, P. Li, N. Wei, X. Zhu, J. Qi and T. Lu, Photoluminescence enhancement of Gd2Zr2O7:Eu3+ red phosphor sensitized by co-doped Al3+ ions, Ceram. Int., 2021, 47, 13071–13077 CrossRef CAS.
- H. Liu, X. Zhu, L. Nie, L. Guo, C. Jiang, G. Wang, W. Huang, L. Hou, T. Hu, A. N. Yakovlev, X. Yu and T. Wang, Multimode-Responsive Luminescence of Er3+ Single-Activated CaF2 Phosphor for Advanced Information Encryption, Inorg. Chem., 2023, 62(40), 16485–16492 CrossRef CAS PubMed.
- J. Jia, X. Gao and G. Zou, Alkaline-Earth-Metal-Ions Blending Enhanced Self-Activated and Bi3+-Activated Mechanoluminescence from Ca1-xBaxZnOS, Adv. Funct. Mater., 2022, 32, 2207881 CrossRef CAS.
- N. Zhang, B. Tian, Z. Wang, A. T. Smith, Z. Ma, Z. Xue and L. Sun, Intense Mechanoluminescence in Undoped LiGa5O8 with Persistent and Recoverable Behaviors, Adv. Opt. Mater., 2021, 9, 2100137 CrossRef CAS.
- J. Zhang, T. Zhang, Z. Qiu, S. Liu, J. Zhang, W. Zhou, L. Yu and S. Lian, Fine-Tunable Self-Activated Luminescence in Apatite-Type (Ba,Sr)5(PO4)3Br and the Defect Process, Inorg. Chem., 2018, 57(19), 12354–12363 CrossRef CAS PubMed.
- W. Lü, X. Kang, Z. Zhu, Q. Pan, F. Zhou and H. Wang, A novel self-activated near-infrared luminescence of BaLaMgTaO6 phosphor, J. Mol. Struct., 2024, 1298, 137082 CrossRef.
- Y. Yang, P. Zhan, W. Xie, Z. Lin, X. Miao, S. Li, H. Wang and W. Liu, Time-dependent multicolor evolution of photoluminescence and afterglow in lanthanide-doped gallate, Chem. Eng. J., 2023, 476, 14648 Search PubMed.
- L. Li, T. Li, Y. Hu, C. Cai, Y. Li, X. Zhang, B. Liang, Y. Yang and J. Qiu, Mechanism of the trivalent lanthanides’ persistent luminescence in wide bandgap materials, Light: Sci. Appl., 2022, 11, 51 CrossRef CAS PubMed.
- J. Wang, B. Liu, W. Chen, Q. Qiang, L. Peng, T. Han, T. Zeng, Z. Zhou, Z. Yang and Y. A. Nikolaevich, Variable temperature persistent luminescence properties of phosphors with continuous traps, J. Lumin., 2022, 243, 118644 CrossRef CAS.
- Y. Wei, Y. Pan, E. Zhou, Z. Yuan, H. Song, Y. Wang, J. Zhou, J. Rui, M. Xu, L. Ning, Z. Liu, H. Wang, X. Xie, X. Tang, H. Su, X. Xing and L. Huang, Frenkel Defect-modulated Anti-thermal Quenching Luminescence in Lanthanide-doped Sc2(WO4)3, Angew. Chem., Int. Ed., 2023, 62(27), e202303482 CrossRef CAS PubMed.
- W. Liu, Y. Zhang, X. Kong, E. Y. B. Pun and H. Lin, Excitation Mechanism Rearrangement in Yb3+-Introduced La2O2S: Er3+/Polyacrylonitrile Photon-Upconverted Nanofibers for Optical Temperature Sensors, ACS Appl. Nano Mater., 2023, 6, 13570–13581 CrossRef.
- Y. Wang, P. Dang, L. Qiu, G. Zhang, D. Liu, Y. Wei, H. Lian, G. Li, Z. Cheng and J. Lin, Multimode Luminescence Tailoring and Improvement of Cs2NaHoCl6 Cryolite Crystals via Sb3+/Yb3+ Alloying for Versatile Photoelectric Applications, Angew. Chem., Int. Ed., 2023, 62(45), e20231169 Search PubMed.
- R. Chen, On Calculation of Activation Energies and Frequency Factors from Glow Curves, J. Appl. Phys., 1969, 40, 570–585 CrossRef CAS.
- K. Eeckhout, A. Bos, D. Poelman and P. Smet, Revealing trap depth distributions in persistent phosphors, Phys. Rev., 2013, 87(4), 45126.36 Search PubMed.
- Z. Li, N. Yu, J. Zhou, Y. Li, Y. Zhang, L. Huang, K. Huang, Y. Zhao, S. Kelmar, J. Yang and G. Han, Coloring Afterglow Nanoparticles for High-Contrast Time-Gating Free Multiplex Luminescence Imaging, Adv. Mater., 2020, 32(49), 2003881 CrossRef CAS PubMed.
- S. Wu, Q. Liu, P. Xiong, W. Chen, Q. Dong, D. Chen, D. Wang, G. Zhang, Y. Chen and G. Li, Single Bi3+ Ultrabroadband White Luminescence in Double Perovskite
via Crystal Lattice Engineering toward Light-Emitting Diode Applications, Adv. Opt. Mater., 2022, 10(11), 2102842 CrossRef CAS.
- Q. Zeng, M. Runowski, J. Xue, L. Luo, L. Marciniak, V. Lavín and P. Du, Pressure-Induced Remarkable Spectral Red-Shift in Mn2+-Activated NaY9(SiO4)6O2 Red-Emitting Phosphors for High-Sensitive Optical Manometry, Adv. Sci., 2024, 11, 2308221 CrossRef CAS PubMed.
- H. Lv, P. Du, W. Li and L. Luo, Tailoring of upconversion emission in Tm3+/Yb3+-codoped Y2Mo3O12 submicron particles via thermal stimulation engineering for noninvasive thermometry, ACS Sustainable Chem. Eng., 2022, 10(7), 2450–2460 CrossRef CAS.
- G. Kresse and J. Furthmüller, Efficiency of ab-initio total energy calculations for metals and semiconductors using a plane-wave basis set, Comput. Mater. Sci., 1996, 6(1), 15–50 CrossRef CAS.
- S. Kurra, N. K. Veldurthi, J. R. Reddy, C. S. Reddy and M. Vithal, A series of novel double perovskite oxides NaMTi2O6 (M = Eu, Sm, and Gd): preparation, characterization and photocatalytic studies under visible and solarlight irradiation, J. Mater. Sci.: Mater. Electron., 2016, 27, 4194–4200 CrossRef CAS.
|
This journal is © the Partner Organisations 2024 |
Click here to see how this site uses Cookies. View our privacy policy here.