DOI:
10.1039/D2QI02398A
(Research Article)
Inorg. Chem. Front., 2023,
10, 824-831
Optimizing the pore space of a robust nickel–organic framework for efficient C2H2/C2H4 separation†
Received
12th November 2022
, Accepted 12th December 2022
First published on 13th December 2022
Abstract
The separation of acetylene (C2H2) from ethylene (C2H4) is an important but challenging process in industry because of their similar physical properties and kinetic molecular sizes. Here, we presented a robust nickel–organic framework (UPC-22) with intrinsic hydrogen bonds and one-dimensional functionalized channels, offering efficient purification of C2H4. UPC-22 exhibits excellent chemical stability at various pH values from 1 to 11 and a high C2H2/C2H4 selectivity of 4.8 at 273 K. Actual breakthrough experiments further demonstrate that UPC-22 is a promising potential adsorbent for C2H2/C2H4 separation with a C2H4 productivity of 1.07 mmol g−1. Grand canonical Monte Carlo simulation and density functional theory indicate that the unsaturated NiII sites and uncoordinated carboxylate groups with an additional contribution from π⋯π packing between aromatic rings provide stronger multipoint interactions with C2H2 over C2H4. This study offers practical guidance to fabricate durable materials for C2H2/C2H4 separation in real industrial scenarios.
Introduction
Ethylene (C2H4), as one of the most important chemical feedstocks, is widely used to manufacture various chemicals and polymers. More than 170 million tons of C2H4 are produced annually through petroleum cracking and ethane dehydrogenation.1 During the C2H4 production, trace amounts of acetylene (C2H2, about 1%) are always mixed with the resultant C2H4-containing gases, which can cause catalyst poisoning and hinder further utilization of C2H4 in the chemical and polymer industries.2 Therefore, the purification of C2H4 is considered to be one of the most critical industrial processes.3 However, the similar physical properties and molecular sizes of C2H2 and C2H4 also make their separation quite challenging. Currently, industrial technologies for C2H2/C2H4 separation, including partial hydrogenation and solvent extraction, are very costly and energy-intensive.4–6 In contrast, adsorptive separation based on porous materials has attracted extensive attention in academia and industry because of its high efficiency and low-energy consumption.7–11
Metal–organic frameworks (MOFs) exhibit excellent application prospects in adsorption and separation because of their designable structures and tunable pore sizes/functionalities.12–18 Recently, MOFs have attracted increasing attention for their potential applications in C2 hydrocarbon separation (C2H2/C2H4, C2H4/C2H6, and C2H2/C2H6, etc.).19–25 To improve the separation performance of MOFs, several strategies have been proposed, including pore size customization, pore surface functionalization, open metal sites (OMSs), etc. Since the first porous MOF (M′MOF) for C2H2/C2H4 separation was reported in 2011, researchers have taken considerable effort to implement some porous MOFs to achieve their efficient separation.26–29 For example, Li et al. achieved high C2H2/C2H4 selectivity through NbU-8 with ultra-microporous building units and –OH groups, which was attributed to the supramolecular interaction between the framework and C2H2 molecules.30 The C–H⋯O/N hydrogen-bonding interaction between MOFs and gas molecules plays a significant role in the selective adsorption of gas molecules.31 A special Cu-MOF (NbU-1) synthesized by Zhou et al. showed one of the highest kinetic separation efficiencies of C2H2/C2H4 through the cooperation of Cu⋯C
C interactions and Lewis-basic sites forming the H–C
C−H⋯N (pyridyl) hydrogen bond.32 Sun et al. reported that ZNU-3 with intrinsic hydrogen bonding exhibited excellent C2H2 separation performance in the presence of C2H4, C2H6, and CO2 due to the synergistic hydrogen bonding of carboxylate groups and π⋯π packing interactions.33 Therefore, an effective method to achieve special recognition for C2H2 is to introduction of particular functional sites, such as OMSs, functional groups, and hydrogen-bonding acceptors.
Based on the above considerations, we constructed a novel nickel-MOF UPC-22 ([Ni3(μ3-OH)2(HATTCA)(H2O)4], H3ATTCA = 2-amino-[1,1:3,1-terphenyl]-4,4,5-tricarboxylic acid), featuring multiple types of pores and specific pore space forming stronger host–guest interactions. UPC-22 not only has excellent thermal and chemical stability, but also has two types of optimized functional channels with OMSs, –NH2, and uncoordinated carboxylate groups. Breakthrough experiments show that UPC-22 can efficiently separate C2H4 from the C2H2/C2H4 mixture. Grand canonical Monte Carlo (GCMC) simulation and density functional theory (DFT) reveal that the unsaturated NiII sites and hydrogen bonding from carboxylate groups play a significant role in C2H4 purification.
Results and discussion
Crystal structure of UPC-22
Conventional rod-shaped UPC-22 crystallizes in an orthorhombic system with the I2b2 space group (Fig. S1 and Table S1†). The Ni2+ ions in the framework adopt an octahedral geometry with oxygen atoms from carboxylate and H2O, which are then arranged into 1D metal chains (Fig. 1c). Adjacent chains are connected by ligands into two-dimensional sheets. Only two of the three carboxylate groups of each H3ATTCA ligand are coordinated to the nickel ions, and the other are protonated and hydrogen-bonded to the adjacent ligand (Fig. 1d). These hydrogen bond arrays connect the layers into a three-dimensional framework with one-dimensional channels. The length of hydrogen bonds (1.901 Å) is largely shorter than the sum of the van der Waals radii of the H and O atoms (2.6 Å), indicating that a strong hydrogen bonding exists (Fig. 1d). There are two rhombic channels in UPC-22 (Fig. 1e): the smaller channel A (Fig. 1a) with approximate dimensions of 2.8 × 16 Å2 and the larger channel B (Fig. 1b) with the dimensions of 6.5 × 15 Å2. Furthermore, there are many open Ni2+ sites and carboxylate groups, which can be regarded as active sites around the rhombic channels and provide a stronger binding affinity for C2H2 than C2H4.
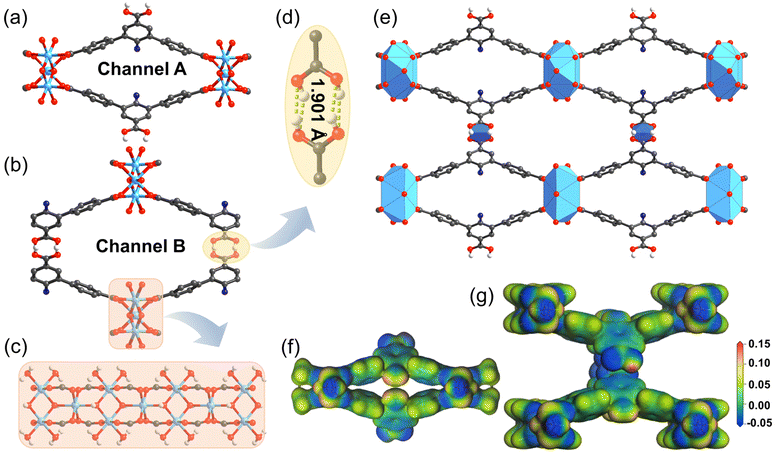 |
| Fig. 1 Crystal structure of UPC-22. (a) and (b) The different channels of the framework in UPC-22; (c) the infinite secondary building units (iSBUs) comprised of one-dimensional (1D) nickel(II) chains; (d) view of the connection of the ligand by hydrogen bonds; (e) the three-dimensional framework with one-dimensional channels along the b-axis; (f) and (g) electrostatic potential (ESP) onto the pore surface of Ni-MOF mapped onto the 0.05 e Å−3 electron density isosurfaces. Ni, O, N and C are represented by green, red, blue, and gray, respectively. | |
Stability of UPC-22
The stability of the adsorbent plays a significant role in practical application. Therefore, we evaluated the thermal and chemical stability of UPC-22. Thermogravimetric analysis (TGA) confirmed the thermal stability of the as-synthesized UPC-22 (Fig. S2†). When heated to 150 °C, the loss of guest water molecules was observed. Up to 375 °C, a substantial decline in quality was observed, and the structure began to collapse, which was consistent with the result of variable temperature powder X-ray diffraction (PXRD; Fig. S3†). The PXRD of bulk crystalline samples was conducted to examine the purity and chemical stability (Fig. 2). The chemical stability was determined by soaking UPC-22 samples in solutions of different pH values. There are no obvious peak changes in the PXRD patterns; thus, UPC-22 can retain its structural integrity over 24 h in water in different acidic/basic aqueous solutions (pH, 1–11). The high stability of UPC-22 can be attributed to the stable multicore nickel metal chain and the strong Ni–O bond with a length of 2.034–2.093 Å. In a word, thermal and chemical stability in acidic, basic, and neutral aqueous solutions makes UPC-22 an extraordinary adsorbent.
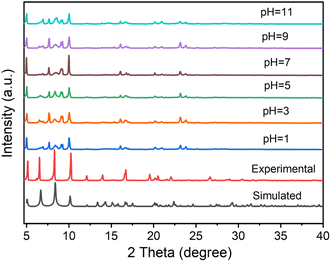 |
| Fig. 2 PXRD patterns of UPC-22 simulated from X-ray crystal diffraction data, experimental and samples sustained in a solution of a given pH, respectively. | |
Permanent porosity
The permanent porosity of activated UPC-22 was measured using the N2 sorption isotherm at 77 K. As shown in Fig. 3a, UPC-22 with a typical reversible type-I N2 adsorption isotherm shows a saturated uptake of 165.6 cm3 g−1, revealing the permanent porosity of the microporous material. The corresponding Brunauer–Emmett–Teller (BET) surface area and total pore volume are 486.3 m2 g−1 and 0.26 cm3 g−1, respectively. In addition, there are two pore systems of around 3.8 Å and 6.5 Å (Fig. 3a), which is consistent with the single crystal data.
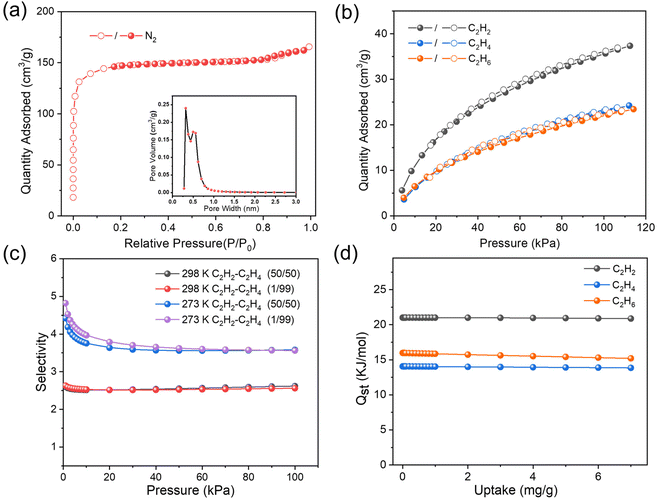 |
| Fig. 3 (a) The N2 adsorption isotherm in UPC-22 at 77 K and the pore size distribution for UPC-22. (b) The C2H2, C2H4, and C2H6 adsorption isotherms in UPC-22 at 298 K. (c) Adsorption selectivities of UPC-22 calculated by the IAST method for the mixtures of C2H2/C2H4 at 273 K and 298 K. (d) Isosteric heats of adsorption of C2H2, C2H4, and C2H6. | |
Adsorption and separation of C2 hydrocarbons
Considering the two types of rhombic channels decorated with carboxylate O atoms as hydrogen bond acceptors and dense open NiII sites, we further investigated the adsorption performance of UPC-22 for C2H2, C2H4, and C2H6. As shown in Fig. 3b and Fig. S5,† the single adsorption isotherms of C2H2, C2H4, and C2H6 at 298 and 273 K were obtained, respectively. The results show that there are significant differences in the adsorption capacity of UPC-22 for C2 hydrocarbons. Compared with C2H4 and C2H6, the type I curve of C2H2 has a steeper upward trend, indicating a stronger host–guest interaction between C2H2 and the framework. The C2H2 uptake of UPC-22 under 1 bar is 46.8 cm3 g−1 at 273 K and 37.4 cm3 g−1 at 298 K. For C2H4 and C2H6, the maximum adsorption capacities are relatively small, only 29.2 and 28.6 cm3 g−1 at 273 K and 24.2 and 23.4 cm3 g−1 at 298 K, respectively. The large adsorption capacity of C2H2 with the uptake ratio of C2H2/C2H4 is 1.55 at 298 K, which is greater than the reported values for the MOF-74 series (1.21–1.36),34 ZJNU-115 (1.26),31 ZJNU-119 (1.33),35 and ZJNU-7 (1.4).15 The great differences in the adsorption capacity between C2H2, C2H4, and C2H6 in UPC-22 revealed the large potential for one-step purification of C2H4 and C2H6. Then, the adsorption enthalpies (Qst) of the three gases were calculated based on the Clausius-Clapeyron equation (Fig. 3d and Fig. S6†). The Qst values for C2H2, C2H4, and C2H6 at zero loading are 21.1, 15.9, and 14.1 kJ mol−1, respectively. The Qst of UPC-22 for C2H2 is much higher than that for C2H4 over the entire loading range, indicating that UPC-22 has a strong affinity for C2H2. For practical application, the moderate Qst values of UPC-22 show that it can reduce the energy consumption of adsorbate recovery and adsorbent regeneration.
Inspired by the difference in the adsorption capacity and Qst for C2H2, C2H4, and C2H6, the ideal adsorption solution theory (IAST) calculations were used to evaluate the separation performance of C2H2/C2H4 and C2H2/C2H6 in UPC-22. As shown in Fig. 3c and Fig. S7,† the C2H2/C2H4 adsorption selectivity decreased first and then remained constant, while the C2H2/C2H6 selectivity showed an increasing trend. At the initial pressure, the corresponding IAST selectivity for C2H2/C2H4 (v/v, 1
:
99) was 2.7 at 298 K and 4.8 at 273 K; the C2H2/C2H4 selectivity for the molar ratio of 50
:
50 (v/v) at 298 K and 273 K was 2.6 and 4.4, respectively (Fig. 3c). Obviously, under the ambient conditions, the C2H2/C2H4 selectivity of UPC-22 is higher than that of most reported promising MOFs, such as MgMOF-74 (2.18),36 FeMOF-74 (2.08),37 BSF-1 (2.3),38 NUM-9a (1.63),4 NOTT-300 (2.17),39 and NUM-12a (1.4),40 but lower than that of some leading MOFs, such as SNNU-40 (4.5)41 and ZJU-198 (7.2).42 Furthermore, the C2H2/C2H6 selectivity is 2.6 and 2.4 at 298 K, corresponding to the ratio of 50
:
50 (v/v) and 1
:
99 (v/v), respectively (Fig. S7†). The above results indicate that UPC-22 is a potential C2H2-selective material to purify C2H4 and C2H6.
Encouraged by the above results, we further tested the actual separation performance of C2H2/C2H4 and C2H2/C2H6 under ambient conditions. The experimental breakthrough studies of C2H2/C2H4 (50
:
50, v/v) and C2H2/C2H6 (50
:
50, v/v) mixtures were performed using the fully degassed UPC-22 samples under a total flow of 3 mL min−1. The breakthrough curves of the C2H2/C2H4 and C2H2/C2H6 mixtures are shown in Fig. 4. For C2H2/C2H4 mixtures, owing to the low C2H4 affinity of UPC-22, the breakthrough of C2H4 occurred in the early stage, while C2H2 was detected after about 8 min g−1 and the productivity was 1.07 mmol g−1 (Fig. 4a). UPC-22 can purify the C2H2/C2H4 mixture into high-purity C2H4 (99.99%) at 298 K with good cycling stability (Fig. S11†). The productivity of C2H4 in the C2H2/C2H4 mixture is higher than those of some promising MOFs, such as ZJU-HOF-1 (0.98 mmol g−1)43 and ZnBAIm (0.3 mmol g−1).44 In addition, the breakthrough study was conducted with C2H2/C2H6 under the same conditions (Fig. 4b). The penetration of C2H6 was first detected, and the penetration time of C2H2 was about 7 min g−1.
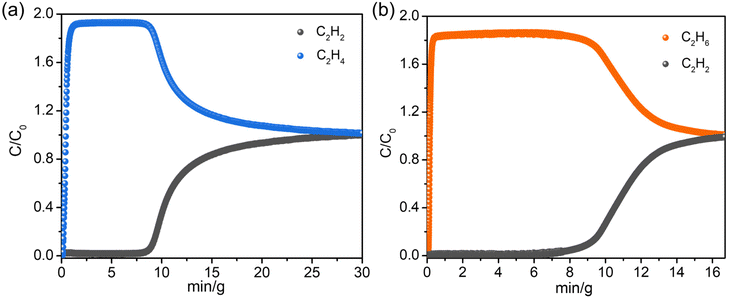 |
| Fig. 4 Column breakthrough curves of UPC-22 for (a) C2H2/C2H4 and (b) C2H2/C2H6 mixtures at 298 K and 1 bar. | |
GCMC and DFT calculations were further carried out to obtain preferential adsorption sites and gain some meaningful insights. First, the adsorption properties of UPC-22 for C2H2 and C2H4 at 298 K were investigated by GCMC simulations. There are three primary adsorption sites between C2H2 and C2H4 molecules and the channel surface (Fig. 5a, d and S9–S10†). The adsorbed gas molecules are preferentially located around the open NiII sites and uncoordinated carboxylate groups in the larger channel B, whereas few near the amine groups of ligands in the channel A with a smaller size. DFT calculations were further conducted to obtain the optimized adsorption geometrical structures and the adsorption energy between C2H2/C2H4 and the framework. The DFT calculated adsorption sites are in good agreement with the results of GCMC simulations (Fig. 5b, c, 5e, f and Fig. S11†). The adsorption energy of C2H2 molecules on open NiII sites is larger than that of C2H4, with the corresponding values of 49.94 and 48.41 kJ mol−1 (Fig. 5b and c), which are attributed to the synergistic effect of C–H⋯O hydrogen bonds and stronger Coulomb attraction between open NiII sites and C
C with π electrons. Another adsorption site of C2H2 is the non-coordinating carboxylate group and benzene ring with an adsorption energy of 47.78 kJ mol−1, forming strong and multiple O–COO⋯H–C
C–H⋯π hydrogen bonds and van der Waals interactions with close distances of 2.5/3.3 Å (Fig. 5b). In contrast to C2H2, the adsorption energy of C2H4 at this site (42.50 kJ mol−1) is significantly lower with larger distances between C2H4 and the framework (Fig. 5e). Moreover, the results of the electrostatic potential revealed that surface O atoms of non-coordinating carboxylate sites exhibited a strong negative potential, which could attractively interact with H atoms from C2H2 and C2H4 by forming strong C–H⋯O hydrogen bonds (Fig. 1f, g and S12†). Furthermore, the adsorption energies of C2H2 and C2H4 at the –NH2 site in channel A are 47.91 and 37.12 kJ mol−1 (Fig. S11†), respectively, indicating that –NH2 is a strong adsorption site, whereas only a few gas molecules are adsorbed (Fig. S9†) due to the small size of channel A (2.8 × 16 Å2) hindering the entry of gas molecules. Thus, the synergy between OMSs/carboxylate sites and appropriate rhombic channels with aromatic π-electrons can create a multi-binding environment to selectively capture C2H2 from C2H4 and C2H6.
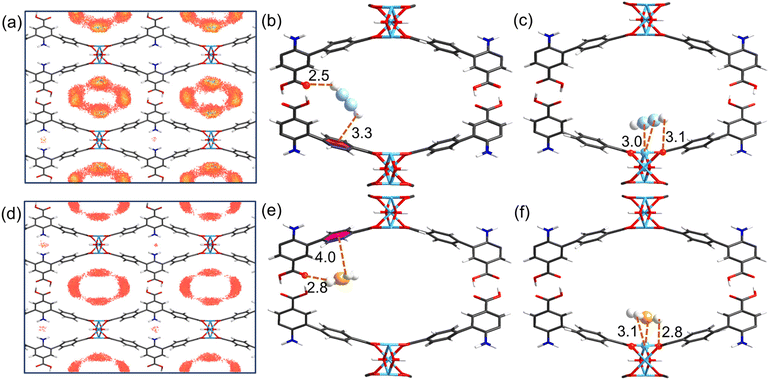 |
| Fig. 5 Results of the GCMC simulations showing preferential binding sites between the adsorbed molecule and UPC-22: (a) C2H2 and (d) C2H4 at 298 K and 30 KPa; binding sites of C2H2 and C2H4 in UPC-22 determined by DFT simulations (b–c) and (e–f). | |
Conclusions
In conclusion, a novel robust MOF (UPC-22) containing two types of rhombic channels decorated with uncoordinated –COOH groups, –NH2 groups, and OMSs was successfully constructed by the hydrothermal method. UPC-22 exhibits a high selectivity toward C2H2/C2H4 (2.7) under ambient conditions, outperforming most of the promising MOFs. Theoretical calculations reveal that the excellent separation performances are mainly attributed to the aromatic channels with abundant functional groups and OMSs that enable stronger interaction with C2H2 molecules than that with C2H4. The high framework stability and dynamic breakthrough experiments demonstrate its application prospects in ethylene purification in the petrochemical industry.
Experimental and computational section
Materials and measurements
All materials were used in the commercially available form without further purification. The ligand 2-amino-[1,1:3,1-terphenyl]-4,4,5-tricarboxylic acid (H3ATTCA) was prepared according to previously published procedures.45 The powder X-ray diffraction (XRD) data were obtained on an X-Pert PRO MPD diffractometer with Cu-Kα radiation. IR spectra were obtained on a Nexus FT-IR spectrometer using KBr pellets in the frequency range of 4000–400 cm−1. Elemental analyses (C, H, and N) were performed on an instrument EA 1110 elemental analyzer. Thermo-gravimetric analysis (TGA) experiments were conducted from 40 to 900 °C on a Mettler Toledo TGA instrument with a heating rate of 10 °C min−1 under a N2 atmosphere. Low-pressure (<800 torrs) N2, C2H2, C2H4, and C2H6 adsorption isotherms were obtained using a Micrometrics ASAP 2020 surface area and pore size analyzer. The Brunauer–Emmett–Teller (BET) surface area was calculated from N2 adsorption isotherms at 77 K. Pore size distribution data were also obtained from the N2 adsorption isotherms at 77 K based on a non-local density functional theory (NLDFT) model in the Micromeritics ASAP 2020 software package (assuming a slit pore geometry). The breakthrough experiments were performed on dynamic gas breakthrough equipment. The experiments were conducted using a stainless-steel column. The weight of UPC-22 packed in the columns was 0.6267 g. The mixed gas of C2H2/C2H4 and C2H2/C2H6 (50/50, v/v) was then introduced with a flow rate of 3 mL min−1. More details of breakthrough equipment are shown in the ESI.†
Synthesis of UPC-22
H3ATTCA (7.5 mg, 0.02 mmol) and Ni(NO3)2·6H2O (30 mg, 0.10 mmol) in 3 mL of DMF (N,N-dimethylformamide)
:
H2O (v/v = 1
:
1) were ultrasonically dissolved in a Pyrex vial. The Pyrex vial was sealed, heated to 100 °C for 3 days, and then cooled to room temperature. The resulting light-green block crystals were washed multiple times with DMF prior to single-crystal X-ray diffraction analysis. Yield: about 72% based on nickel. Elemental analysis calcd (%) for UPC-22: C, 38.24 N, 2.12 H, 3.64; found: C, 38.31 N, 2.19 H, 3.56.
Computational methods
In this work, the adsorption isotherms of pure C2H2 and C2H4 in UPC-22 were obtained using the Grand Canonical Monte Carlo (GCMC) method implemented in the Materials Studio code.46 The site-site L–J potential was described with the L–J (12, 6) model, and the electrostatic interaction was calculated via the Coulomb law. The cutoff of 8 Å was employed for the L–J interactions. Density functional theory (DFT) was performed to provide the atomic partial charges and electronic potential of the UPC-22 framework and calculate the optimized structures and energies of the C2H2 and C2H4 interaction with fragmented clusters.47 Generalized gradient approximation (GGA) with the Perdew-Burke-Ernzerhof (PBE) functional was used to do all-electron spin-unrestricted DFT calculations. DFT-D calculations were performed using the Grimme parameters for the van der Waals correction of gas adsorption. The details of all the calculations are given in the ESI.†
Conflicts of interest
There are no conflicts to declare.
Acknowledgements
This work was supported by the National Natural Science Foundation of China (NSFC, Grant No. 22108113, 22201305), the Natural Science Foundation of Shandong Province (ZR2020QB032), the Fundamental Research Funds for the Central Universities (22CX06024A), and the Outstanding Youth Science Fund Projects of Shandong Province (2022HWYQ-070).
References
- L. Li, R.-B. Lin, R. Krishna, H. Li, S. Xiang, H. Wu, J. Li, W. Zhou and B. Chen, Ethane/ethylene separation in a metal-organic framework with iron-peroxo sites, Science, 2018, 362, 443–446 CrossRef PubMed.
- Z. L. Ma, P. X. Liu, Z. Y. Liu, J. J. Wang, L. B. Li and L. Tian, Thermally and chemically stable copper(II) metal-organic framework with high performance for gas adsorption and separation, Inorg. Chem., 2021, 60, 6550–6558 CrossRef PubMed.
- G.-D. Wang, Y.-Z. Li, W.-J. Shi, L. Hou, Y.-Y. Wang and Z. Zhu, One-step C2H4 purification from ternary C2H6/C2H4/C2H2 mixtures by a robust metal-organic framework with customized pore environment, Angew. Chem., Int. Ed., 2022, e202205427 Search PubMed.
- S.-Q. Yang, F.-Z. Sun, P. Liu, L. Li, R. Krishna, Y.-H. Zhang, Q. Li, L. Zhou and T.-L. Hu, Efficient purification of ethylene from C2 hydrocarbons with an C2H6/C2H2-selective metal−organic framework, ACS Appl. Mater. Interfaces, 2021, 13, 962–969 CrossRef PubMed.
- Q. Hong, W. Wang, S. Chen, K. Chen, M. Liu, H.-X. Zhang and J. Zhang, Host−guest pore space partition in a boron imidazolate framework for ethylene separation, Chem. Mater., 2022, 34, 307–313 CrossRef CAS.
- C. Gu, J. Liu, J. Hu and D. Wu, Highly selective separations of C2H2/C2H4 and C2H2/C2H6 in metal–organic frameworks via pore environment design, Ind. Eng. Chem. Res., 2019, 58, 19946–19957 CrossRef CAS.
- B.-Y. Zhu, T. Zhang, C.-H. Li, J.-W. Cao, Z.-Q. Zhang, W. Qi, G.-Y. Wang, Z.-H. Rong, Y. Wang and K.-J. Chen, A (3,8)-connected metal−organic framework with bending dicarboxylate linkers for C2H2/CO2 separation, Inorg. Chem., 2022, 61, 4555–4560 CrossRef CAS PubMed.
- Y.-L. Peng, T. Pham, P. Li, T. Wang, Y. Chen, K.-J. Chen, K. A. Forrest, B. Space, P. Cheng, M. J. Zaworotko and Z. Zhang, Robust ultramicroporous metal–organic frameworks with benchmark affinity for acetylene, Angew. Chem., Int. Ed., 2018, 57, 10971–10975 CrossRef CAS PubMed.
- K. Su, W. Wang, S. Du, C. Ji and D. Yuan, Efficient ethylene purification by a robust ethane-trapping porous organic cage, Nat. Commun., 2021, 12, 3703 CrossRef CAS.
- C. Ji, K. Su, W. Wang, J. Chang, E.-S. M. El-Sayed, L. Zhang and D. Yuan, Tunable cage-based three-dimensional covalent organic frameworks, CCS Chem., 2022, 4, 3095–3105 CrossRef CAS.
- L. Li, C. Ji, W. Wang, F. Wu, Y.-X. Tan and D. Yuan, The effect of pore sizes on D2/H2 separation conducted by MOF-74 analogues, Inorg. Chem. Front., 2022, 9, 1674–1680 RSC.
- L.-N. Ma, L. Zhang, W.-F. Zhang, Z.-H. Wang, L. Hou and Y.-Y. Wang, Amide-functionalized In-MOF for effective hydrocarbon separation and CO2 catalytic fixation, Inorg. Chem., 2022, 61, 2679–2685 CrossRef CAS.
- X.-W. Gu, J.-X. Wang, E. Wu, H. Wu, W. Zhou, G. Qian, B. Chen and B. Li, Immobilization of lewis basic sites into a stable ethane-selective MOF enabling one-step separation of ethylene from a ternary mixture, J. Am. Chem. Soc., 2022, 144, 2614–2623 CrossRef CAS.
- G.-D. Wang, Y.-Z. Li, W.-F. Zhang, L. Hou, Y.-Y. Wang and Z. Zhu, Acetylene separation by a Ca-MOF containing accessible sites of open metal centers and organic groups, ACS Appl. Mater. Interfaces, 2021, 13, 58862–58870 CrossRef CAS.
- Z. Jiang, L. Fan, P. Zhou, T. Xu, S. Hu, J. Chen, D.-L. Chen and Y. He, An aromatic-rich cage-based MOF with inorganic chloride ions decorating the pore surface displaying the preferential adsorption of C2H2 and C2H6 over C2H4, Inorg. Chem. Front., 2021, 8, 1243–1252 RSC.
- S. Zou, Z. Di, H. Li, Y. Liu, Z. Ji, H. Li, C. Chen, M. Wu and M. Hong, Stable fluorinated hybrid microporous material for the efficient separation of C2–C3 alkyne/alkene mixtures, Inorg. Chem., 2022, 61, 7530–7536 CrossRef CAS.
- Z. Jiang, Y. Zou, T. Xu, L. Fan, P. Zhou and Y. He, An aromatic-rich cage-based MOF with inorganic chloride ions decorating the pore surface displaying the preferential adsorption of C2H2 and C2H6 over C2H4, Dalton Trans., 2020, 49, 3553–3561 RSC.
- Z. Jiang, L. Fan, P. Zhou, T. Xu, J. Chen, S. Hu, D.-L. Chen and Y. He, A hydrostable cage-based MOF with open metal sites and Lewis basic sites immobilized in the pore surface for efficient separation and purification of natural gas and C2H2, Dalton Trans., 2020, 49, 15672–15681 RSC.
- H. Cheng, Q. Wang, M. Ding, Y. Gao, D. Xue and J. Bai, Modifying a partial corn-sql layer-based (3,3,3,3,4,4)-c topological MOF by substitution of OH(-) with Cl(-) and its highly selective adsorption of C2 hydrocarbons over CH4, Dalton Trans., 2021, 50, 4840–4847 RSC.
- S. R. Acharya, A. Elias, K. Tan, S. Jensen, R.-B. Lin, B. Chen, M. D. Gross and T. Thonhauser, Identifying the gate-opening mechanism in the flexible metal−organic framework UTSA-300, Inorg. Chem., 2022, 61, 5025–5032 CrossRef CAS PubMed.
- Q.-L. Qian, X.-W. Gu, J. Pei, H.-M. Wen, H. Wu, W. Zhou, B. Li and G. Qian, A novel anion-pillared metal–organic framework for highly efficient separation of acetylene from ethylene and carbon dioxide, J. Mater. Chem. A, 2021, 9, 9248–9255 RSC.
- F.-Z. Sun, S.-Q. Yang, R. Krishna, Y.-H. Zhang, Y.-P. Xia and T.-L. Hu, Microporous metal−organic framework with a completely reversed adsorption relationship for C2 hydrocarbons at room temperature, ACS Appl. Mater. Interfaces, 2020, 12, 6105–6111 CrossRef CAS PubMed.
- S. Geng, E. Lin, X. Li, W. Liu, T. Wang, Z. Wang, D. Sensharma, S. Darwish, Y. H. Andaloussi, T. Pham, P. Cheng, M. J. Zaworotko, Y. Chen and Z. Zhang, Scalable room-temperature synthesis of highly robust ethane-selective metal−organic frameworks for efficient ethylene purification, J. Am. Chem. Soc., 2021, 143, 8654–8660 CrossRef CAS.
- H.-J. Lv, J.-W. Zhang, Y.-C. Jiang, S.-N. Li, M.-C. Hu and Q.-G. Zhai, Micropore regulation in ultrastable [Sc3O]-organic frameworks for acetylene storage and purification, Inorg. Chem., 2022, 61, 3553–3562 CrossRef CAS PubMed.
- Y.-Z. Li, H.-H. Wang, G.-D. Wang, L. Hou, Y.-Y. Wang and Z. Zhu, A Dy6-cluster-based fcu-MOF with efficient separation of C2H2/C2H4 and selective adsorption of benzene, Inorg. Chem. Front., 2021, 8, 376–382 RSC.
- W. Fan, S. B. Peh, Z. Zhang, H. Yuan, Z. Yang, Y. Wang, K. Chai, D. Sun and D. Zhao, Tetrazole-functionalized zirconium metal-organic cages for efficient C2H2/C2H4 and C2H2/CO2 separations, Angew. Chem., Int. Ed., 2021, 60, 17338–17343 CrossRef CAS.
- X. Zhang, M. Fu, H. Liu, Y. Wang, Y. Zou, L. Wang, C. Li, Y. Lu, L. Zhou and X. Cui, A copper-based metal–organic framework with a suitable pore environment for effective ethylene purification, Inorg. Chem. Front., 2022, 9, 2104–2108 RSC.
- J. Zhao, Q. Li, X.-C. Zhu, J. Li and D. Wu, Highly robust tetranuclear cobalt-based 3D framework for efficient C2H2/CO2 and C2H2/C2H4 separations, Inorg. Chem., 2020, 59, 14424–14431 CrossRef PubMed.
- J. Wang, Y. Zhang, P. Zhang, J. Hu, R.-B. Lin, Q. Deng, Z. Zeng, H. Xing, S. Deng and B. Chen, Optimizing pore space for flexible-robust metal−organic framework to boost trace acetylene removal, J. Am. Chem. Soc., 2020, 142, 9744–9751 CrossRef PubMed.
- Q. Li, N. Wu, J. Li and D. Wu, A highly connected trinuclear cluster based metal−organic framework for efficient separation of C2H2/C2H4 and C2H2/CO2, Inorg. Chem., 2020, 59, 13005–13008 CrossRef.
- L. Fan, P. Zhou, X. Wang, L. Yue, L. Li and Y. He, Rational construction and performance regulation of an In(III)-tetraisophthalate framework for one-step adsorption-phase purification of C2H4 from C2 Hydrocarbons, Inorg. Chem., 2021, 60, 10819–10829 CrossRef PubMed.
- J. Li, L. Jiang, S. Chen, A. Kirchon, B. Li, Y. Li and H.-C. Zhou, Rational construction and performance regulation of an In(III)-tetraisophthalate framework for one-step adsorption-phase purification of C2H4 from C2 Hydrocarbons, J. Am. Chem. Soc., 2019, 141, 3807–3811 CrossRef.
- W.-Q. Sun, J.-B. Hu, Y.-J. Jiang, N. Xu, L.-Y. Wang, J.-H. Li, Y.-Q. Hu, S. Duttwyler and Y.-B. Zhang, Flexible molecular sieving of C2H2 from CO2 by a new cost-effective metal organic framework with intrinsic hydrogen bonds, Chem. Eng. J., 2022, 439, 135745 CrossRef.
- X. Cui, K. Chen, H. Xing, Q. Yang, R. Krishna, Z. Bao, H. Wu, W. Zhou, X. Dong, Y. Han, B. Li, Q. Ren, M. J. Zaworotko and B. Chen, Pore chemistry and size control in hybrid porous materials for acetylene capture from ethylene, Science, 2016, 353, 141–144 CrossRef.
- P. Zhou, X. Wang, L. Yue, L. Fan and Y. He, A microporous MOF constructed by cross-linking helical chains for efficient purification of natural gas and ethylene, Inorg. Chem., 2021, 60, 14969–14977 CrossRef PubMed.
- S. Xiang, W. Zhou, Z. Zhang, M. A. Green, Y. Liu and B. Chen, Open metal sites within isostructural metal–organic frameworks for differential recognition of acetylene and extraordinarily high acetylene storage capacity at room temperature, Angew. Chem., Int. Ed., 2010, 49, 4615–4618 CrossRef PubMed.
- E. D. Bloch, W. L. Queen, R. Krishna, J. M. Zadrozny, C. M. Brown and J. R. Long, Hydrocarbon separations in a metal-organic framework with open iron(II) coordination sites, Science, 2012, 335, 1606–1610 CrossRef PubMed.
- Y. Zhang, L. Yang, L. Wang, S. Duttwyler and H. Xing, A microporous metal-organic framework supramolecularly assembled from a CuII dodecaborate cluster complex for selective gas separation, Angew. Chem., Int. Ed., 2019, 58, 8145–8150 CrossRef.
- S. Yang, A. J. Ramirez-Cuesta, R. Newby, V. Garcia-Sakai, P. Manuel, S. K. Callear, S. I. Campbell, C. C. Tang and M. Schröder, Supramolecular binding and separation of hydrocarbons within a functionalized porous metal–organic framework, Nat. Chem., 2015, 7, 121–129 CrossRef PubMed.
- Q. Zhang, S.-Q. Yang, L. Zhou, L. Yu, Z.-F. Li, Y.-J. Zhai and T.-L. Hu, Pore-space partition through an embedding metal-carboxylate chain-induced topology upgrade strategy for the separation of acetylene/ethylene, Inorg. Chem., 2021, 60, 19328–19335 CrossRef.
- Y. P. Li, Y. N. Zhao, S. N. Li, D. Q. Yuan, Y. C. Jiang, X. Bu, M. C. Hu and Q. G. Zhai, Ultrahigh-uptake capacity-enabled gas separation and fruit preservation by a new single-walled nickel-organic framework, Adv. Sci., 2021, 8, 2003141 CrossRef.
- L. Zhang, K. Jiang, M. Jiang, D. Yue, Y. Wan, H. Xing, Y. Yang, Y. Cui, B. Chen and G. Qian, A highly stable amino-coordinated MOF for unprecedented block off N2 adsorption and extraordinary CO2/N2 separation, Chem. Commun., 2016, 52, 13568–13571 RSC.
- X. Zhang, J.-X. Wang, L. Li, J. Pei, R. Krishna, H. Wu, W. Zhou, G. Qian, B. Chen and B. Li, A rod-packing hydrogen-bonded organic framework with suitable pore confinement for benchmark ethane/ethylene separation, Angew. Chem., Int. Ed., 2021, 60, 10304–10310 CrossRef PubMed.
- D. Luo, Y.-L. Peng, M. Xie, M. Li, A. A. Bezrukov, T. Zuo, X.-Z. Wang, Y. Wu, Y. Y. Li, A. R. Lowe, M. a. Chorążewski, Y. Grosu, Z. Zhang, M. J. Zaworotko, X.-P. Zhou and D. Li, Improving ethane/ethylene separation performance under humid conditions by spatially modified zeolitic imidazolate frameworks, ACS Appl. Mater. Interfaces, 2022, 14, 11547–11558 CrossRef.
- W. Fan, H. Lin, X. Yuan, F. Dai, Z. Xiao, L. Zhang, L. Luo and R. Wang, Expanded porous metal–organic frameworks by SCSC: organic building units modifying and enhanced gas-adsorption properties, Inorg. Chem., 2016, 55, 6420–6425 CrossRef.
- A. Gupta, S. Chempath, M. J. Sanborn, L. A. Clark and R. Q. Snurr, Object-oriented programming paradigms for molecular modeling, Mol. Simul., 2003, 29, 29–46 CrossRef.
- B. Delley, From molecules to solids with the DMol3 approach, J. Chem. Phys., 2000, 113, 7756–7764 CrossRef.
Footnotes |
† Electronic supplementary information (ESI) available: Simulation details, SEM images, TG curves, IR spectrum, and gas adsorption results. CCDC 2167452. For ESI and crystallographic data in CIF or other electronic format see DOI: https://doi.org/10.1039/d2qi02398a |
‡ These authors contributed equally. |
|
This journal is © the Partner Organisations 2023 |