DOI:
10.1039/D2TB00935H
(Review Article)
J. Mater. Chem. B, 2022,
10, 7450-7459
Recent advances in nanotechnology mediated mitochondria-targeted imaging
Received
29th April 2022
, Accepted 13th July 2022
First published on 18th July 2022
Abstract
Mitochondria play a critical role in cell growth and metabolism. And mitochondrial dysfunction is closely related to various diseases, such as cancers, and neurodegenerative and cardiovascular diseases. Therefore, it is of vital importance to monitor mitochondrial dynamics and function. One of the most widely used methods is to use nanotechnology-mediated mitochondria targeting and imaging. It has gained increasing attention in the past few years because of the flexibility, versatility and effectiveness of nanotechnology. In the past few years, researchers have implemented various types of design and construction of the mitochondrial structure dependent nanoprobes following assorted nanotechnology pathways. This review presents an overview on the recent development of mitochondrial structure dependent target imaging probes and classifies it into two main sections: mitochondrial membrane targeting and mitochondrial microenvironment targeting. Also, the significant impact of previous research as well as the application and perspectives will be demonstrated.
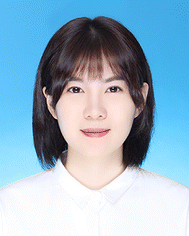
Nannan Zheng
| Dr Nannan Zheng obtained her PhD in Biomedical Engineering from Harbin Institute of Technology in 2021. After graduation, she started her postdoctoral career at Harbin Institute of Technology. Her research interests are mainly focused on the multifunctional nanoparticles for cancer diagnosis and therapy. Dr Zheng's research also focuses on nanoparticles for the treatment of Alzheimer's disease. |
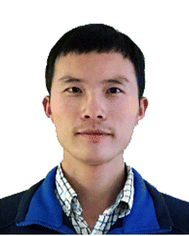
Liangcan He
| Dr Liangcan He graduated from Tsinghua University and National Center for Nanoscience and Technology in 2014 under the guidance of Prof. Zhiyong Tang and Prof. Yadong Li. After that, he joined Prof. Jennifer N. Cha's group in the University of Colorado, Boulder, as a Research Associate, and later worked with Prof. Jennifer N. Cha and Prof. Christopher N. Bowman as a Senior Research Associate. In June 2018, he joined Dr Shawn Chen's lab in the National Institutes of Health as a Postdoc Research Scientist. In May 2021, he started his independent career at Harbin Institute of Technology. His research interests focus on design and synthesis of organic–inorganic nanomaterials for biomedical applications. |
1. Introduction
Known to be the cell's main energy supply organelles, mitochondria are the primary source of intracellular reactive oxygen species and are responsible for modulation of apoptosis.1,2 Also, mitochondria are closely associated with many diseases involving cellular energy disorders and oxidative damage, such as neurodegeneration, cardiovascular diseases and metabolic diseases.3–5 To gain a deeper understanding of the diagnosis and treatment of these diseases, mitochondrial movement and dynamic changes have drawn intense research with recent advances in mitochondrial research, bioenergetics and metabolism, reactive oxygen species and cell signal detection. However, since the ultrastructure of mitochondria is typically close to the diffraction limit in optical microscopy, it would be challenging to directly observe submitochondrial architecture or protein distributions using standard light microscopes. As a result, there is a substantial lack of research and study on subcellular organelles. Thanks to the development of mitochondria-targeted imaging techniques and more advanced microscopy technology, changes in the internal microstructure of mitochondria are gradually becoming better understood. Currently, the techniques used in mitochondria-targeted imaging mainly include implementing small molecule fluorescent probes, polypeptide fluorescent probes, and nanoscale fluorescent probes. The fluorescent probes can be modified to exhibit multifunctional properties, such as anti-tumor therapy function, and selective targeting mitochondrial reactive oxygen species and reductive species attachment.6–8 To be specific, small molecule fluorescent probes, such as commercial MitoTrack ®Green FM and Rhodamine 123, JC-1, are mainly modified by cations to enable them to locate and image mitochondria. Additionally, mitochondria targeted imaging can also be achieved by the introduction of mitochondrial targeting groups, such as tri-phenyl-phosphine (TPP)-Green,9 IR-780 iodide,10 CAI,11etc. As regards polypeptide fluorescent probes, the mitochondrial localization and fluorescence imaging functions can readily be realized by linking the polypeptides with the fluorophore groups.12 Although various organic fluorescent probes have been used for mitochondrial imaging, the development of fluorescent probes with low toxicity, high selectivity and excellent photostability still face challenges. In recent years, with the rapid development of nanotechnology, nanomaterial-based probes not only offer the advantages of commercial mitochondrial imaging, but also can be designed to respond to the changes in the mitochondrial microenvironment. Mitochondrial imaging based on nanotechnology mainly includes targeting mitochondrial structures (such as the mitochondrial outer membrane, mitochondrial inner membrane, cristae, and mtDNA) and targeting the mitochondrial microenvironment (ATP, pH, and ROS) and signaling molecules (H2S). Mitochondria-targeted imaging is useful in a wide range of applications, including long-term imaging of living mitochondria, exploration of the changes in the mitochondrial structure, microenvironment investigation and treatment for diseases. Here, this paper will review the recent progress on the application of nanomaterials in mitochondrial imaging and elaborate the advantages of nanomaterial implemented mitochondrial imaging by exploring the structural and microenvironmental alterations in mitochondria. This review aims to understand and propose new directions of nanomaterial engineering for the study of mitochondria-related diseases. It is organized in three parts: the first one reviews and discusses the mitochondrial targeting imaging of mitochondrial membrane; the second part is dedicated to microenvironmental imaging. Last, our conclusions and proposals for new generation of technology will be presented in the final section.
2. Mitochondrial membrane targeting imaging
The structure of mitochondria can be divided into four functional regions: mitochondrial outer membrane (OMM), mitochondrial inner membrane (IMM), mitochondrial intermembrane space (IMS), and mitochondrial matrix (MM).3,13,14 The design of various small-molecule mitochondrial targeting probes is realized by exploiting the negative potential of the mitochondrial membrane. Consequently, the fluorescent probes that target the mitochondrial outer membrane mainly include actin chromobody targeting,15 mitochondrial membrane potential16–18 and monoamine oxidase.19,20
Due to the oxidation pathway and proton pump operation, mitochondria have a very large membrane potential, reaching up to −180 mV, which is 5–6 times that of the plasma membrane and is quite different from those of other biological membranes.21 Therefore, cationic compounds are more easily labeled in mitochondria than in other organelles,22 which is the reason why cationic fluorescent compounds such as triphenylphosphorous (TPP+), tetramethylrhodamine methyl ester (TMRM), tetramethylrhodamine ethyl ester (TMRE) and 5,5′,6,6′-tetrachloro-1,1′,3,3′-tetraethylbenzimidazolcarbocyanine iodide (JC-1),23,24 (E)-4-(1H-indol-3-ylvinyl)-N-methylpyridiniumiodide (F16),25,26 dequalinium (DQA),27,28 Rhodamine29,30 and guanidine/biguanidine31 have excellent mitochondrial targeting performance. Therefore, grafting mitochondrial targeting molecules such as triphenylphosphine onto the surface of nanoparticles is a simple strategy to obtain mitochondrial targeting nanoprobes.
Lanthanide doped upconversion nanoparticles (UCNPs) can emit high energy UV or visible light under excitation of near-infrared light, which has the potential for biomedical imaging applications.32,33 However, undecorated UCNPs cannot localize to mitochondria to achieve the function of disease diagnosis and treatment. Recently, Zhao's group synthesized Janus nanostructures by growing porphyrin MOF on Nd3+ sensitized upconversion nanoparticles (UCMTs) and the mitochondrial targeting function was achieved by surface modification of triphenylphosphine34 (Fig. 1). In this work, mitochondria-targeted PDT induces the initiation of endogenous apoptotic pathways, resulting in a superior therapeutic effect to that of untargeted therapies.
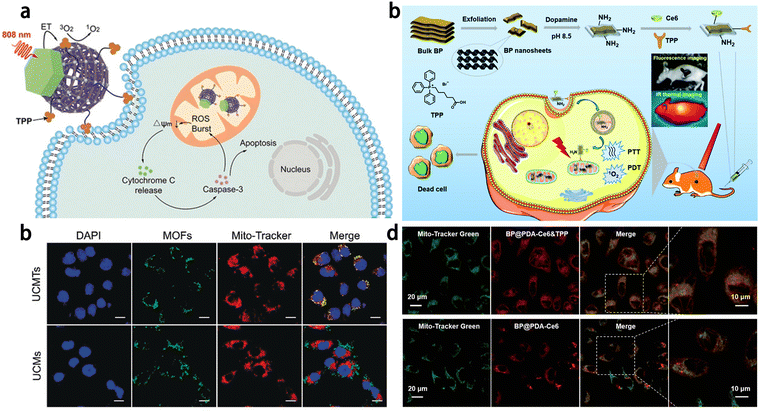 |
| Fig. 1 (a) Schematic of mitochondria-targeted upconversion MOFs for amplified PDT. (b) Confocal fluorescence images of the 4T1 cells treated with UCMTs or UCMs. The green fluorescence is from the MOFs.34 (c) Schematic illustration of the preparation and therapeutic functions of BP@PDA-Ce6&TPP NSs. (d) Confocal fluorescence images of HeLa cells incubated with BP@PDA-Ce6&TPP NSs and BP@PDA-Ce6 NSs (excited at 633 nm) and then Mito-Tracker Green (excited at 488 nm).38 Copyright 2019, wiley and 2018, Royal Society of Chemistry. | |
Black phosphorus (BP) is a novel bioinorganic material with biocompatibility, biodegradability and biosafety. It has been used as a photothermal agent for cancer treatment due to its extensive near infrared absorption.35–37 There is still plenty of potential to improve the therapeutic efficacy of nanomedicine based on BP. In 2018, Dong's group developed an image-guided mitochondrial targeted photothermal/photodynamic nanosystem38 (Fig. 1). Based on dopamine-coated black phosphorus nanosheets, the system covalently linked with Ce6 and TPP to form BP@PDA-Ce6&TPP NSs. TPP modification significantly improved the mitochondrial targeting ability of BP@PDA-Ce6&TPP NSs, and its killing efficiency against tumor cells was significantly improved.
In addition, Zhuang et al. prepared a mitochondrial targeting fluorescent probe AuNC@CS-TPP by combining chitosan-modified Au nanocrystals (AuNC@CS) with TPP through covalent bonding.39 The conjugated compound AuNCs@CS-TPP emits blue fluorescence at 440 nm with a high quantum yield of 8.5%. It is noteworthy that the fluorescence intensity of AuNCs@CS-TPP labeled HeLa cells showed insignificant quenching after irradiation for 8 min, demonstrating its high photostability. Moreover, the studied compound AuNC@CS-TPP can accumulate selectively in the mitochondria of HeLa and HepG2 cells, which suggests its potential for targeted imaging of mitochondria in live cells (Fig. 2). The laser confocal microscope is used in Fig. 2. Combining TPP with traditional nanomaterials, mitochondrial targeting function can be endowed with nanomaterials to achieve mitochondrial imaging and therapeutic effects.
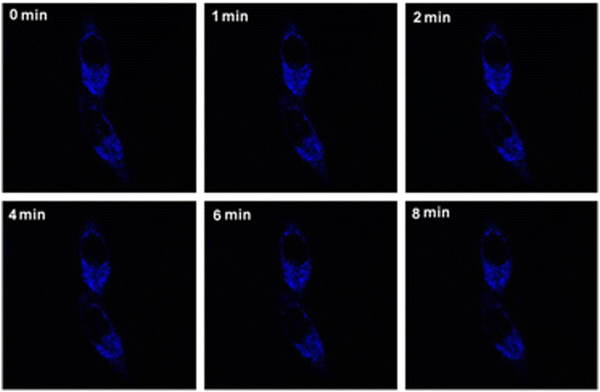 |
| Fig. 2 Fluorescent images of living HeLa cells cultured with AuNCs@CS-TPP (20 μg mL−1) with various incubating times (0–8 min). Excitation wavelength: 405 nm. Reproduced with permission.39 Copyright 2014, Elsevier. | |
Yu et al. constructed a multifunctional nanoplatform (called UR-HAPT) by combining ucNP-based nano-photosensitizer and mitochondrial targeting strategy with a reasonably designed DNA reporter (Fig. 3).40 The platform enables simultaneous monitoring of the subcellular dynamics of human apurinic/apyrimidinic-free endonuclease 1 (APE1) during near-infrared (NIR) light-mediated PDT. APE1 accumulation in mitochondria can be visualized during PDT in vitro and in vivo. Thus, it can be used to screen and evaluate potential enzyme inhibitors to improve the efficacy of PDT.
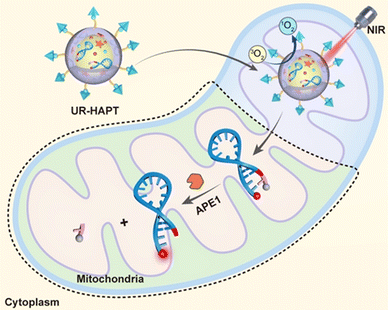 |
| Fig. 3 Schematic illustration of the design of UR-HAPT for precise imaging of subcellular enzymatic dynamics of APE1 during NIR light-mediated, mitochondria-targeted PDT.40 Copyright 2022, Wiley-VCH GmbH. | |
There have been a wide variety of TPP-based compounds that have proved to be useful for mitochondria-targeted imaging, such as NIR Cy-5-TPP/FF,41 WSSe/MnO2-INH-TPP@CM,42 C-dots-TPP43 and Gold-TPP.44
In addition to TPP, which can be used as a small molecular group targeting the mitochondrial outer membrane, PQC is also a group that can target mitochondria. Tang et al.45 designed lipid small molecule hybrid nanoparticles (LPHNPs) for imaging and treatment of in situ glioma models. LPHNPs are prepared by designing a co-assembly of lipids and amphiphilic pheophorbide a-quinolinium conjugate (PQC), a small-molecule-specific mitochondria (Fig. 4). Unlike PQC-NFS, LPHNPs exhibit a spherical nanostructure and ideal particle size, which reduces potential systemic toxicity and enables the administration of nanoparticles by intravenous injection. In addition, compared to traditional liposomes, LPHNPs have a higher drug loading and better stability, and retain the excellent properties of PQC, such as mitochondrial targeting, fluorescence imaging ability and photosensitivity. By integrating the benefits of liposomes and PQC molecules, LPHNPs achieve minimal systemic toxicity, enhanced photodynamic therapy (PDT) efficacy, and the ability to visualize drug biologic distribution and tumor imaging. Mixed nanoparticles showed good efficacy and could significantly prolong the survival time of mice with glioma in situ. Fluorescence imaging showed that LPHNPs accumulated at the tumor site 2 hours after injection and remained there for at least 48 hours. In vitro imaging further confirmed the tumor-targeting ability of LPHNPs. In an in situ glioma model, single-dose LPHNPs combined with laser therapy have a good inhibitory effect on tumor progression. More importantly, they significantly extended the survival time of mice with glioma in situ. The unique co-assembly of lipids and small molecules offers a new potential for the construction of new liposome derived nanoagents and improved cancer therapy.
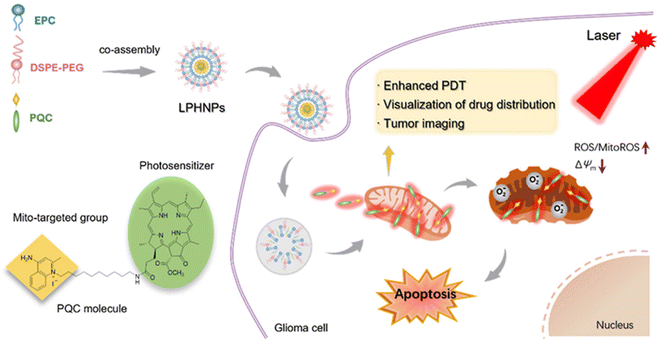 |
| Fig. 4 Schematic illustration of hybrid nanoparticles (LPHNPs) based on amphiphilic lipids and mitochondria targeting PQC molecules.45 Copyright 2022, Elsevier. | |
In addition, the construction of a positively charged surface environment for nanomaterials is another strategy for designing mitochondrial membrane targeted imaging nanoprobes. In 2017, Wu's group prepared fluorescent carbon quantum dots (APTMS CDs) with the mitochondrial targeted imaging function by solvent heat treatment of glycerol and organosilane molecules (3-aminopropyl)trimethoxysilane (APTMS).46 The abundant amino groups on the surface of APTMS CDs lead to a zeta potential of +13.1 mV in water, endowing them with the ability for mitochondrial targeting. Compared with normal cells, cancerous cells have a more negative mitochondrial membrane potential of about −220 mV,47,48 resulting in a stronger electrostatic interaction between APTMS CDs and mitochondrial membrane, and so a brighter fluorescence can be observed in cancerous cells (Fig. 5). Therefore, APTMS CDs can be used to distinguish normal cells and cancerous cells.
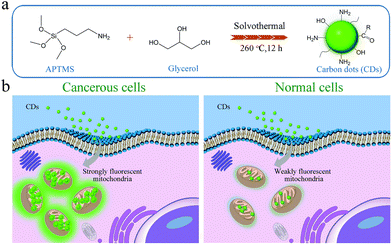 |
| Fig. 5 One-step solvothermal synthesis of APTMS CDs using APTMS and glycerol and applications of APTMS CDs in mitochondrial tracking and normal/cancerous cell differentiation.46 Copyright 2017, Royal Society of Chemistry. | |
3. Mitochondrial microenvironment imaging
3.1 Mitochondrial matrix targeted imaging
Mitochondria matrix is the main structure of mitochondria, and contains numerous proteins/enzymes, nucleic acids, hydrogen sulfide and other biological species for energy supply and signal transduction. These components serve as a medium for mitochondrial imaging.49,50 In order to prolong the retention time of the fluorescence in mitochondria, Grzybowski and Kandere-Grzybowska et al. designed mixed-charged nanocarriers for selective targeting of mitochondria by otherwise nonselective dyes.51 In this work, the mixed-charged nanocarriers traverse an endolysosomal tract to selectively deliver non-covalently bound dye molecules into mitochondria and covalently link them to cysteines of mitochondrial proteins via disulfide bonds (Fig. 6). It is also demonstrated that the nanoparticles of interest can deliver low-toxicity anionic fluorophores into mitochondria to observe the dynamic remodeling process of mitochondrial network and even long-term tracking of mitochondria in dividing cells. Cell mitochondria are imaged using confocal laser scanning microscopy (CLSM).
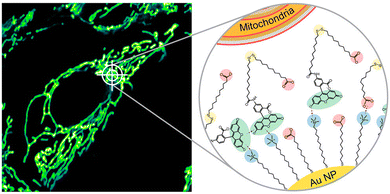 |
| Fig. 6 Mechanism of action of mixed-charge nanocarriers targeting mitochondria.51 Copyright 2021, American Chemical Society. | |
3.2 ATP targeting imaging
Adenosine triphosphate (ATP) consists of three main components: adenine, ribose, and three phosphate groups. When hydrolyzed, a large amount of energy is released, providing a direct source of energy for metabolism and activity.52,53 Mitochondria are the major organelles that produce ATP;54 therefore, mitochondrial imaging can be achieved by monitoring the fluctuation of ATP level in mitochondria or the fluctuation of cellular ATP levels. Chu and coworkers developed a fluorescent ATP probe out of titanium carbide (TC) nanosheets that had been modified with an ROX (X-rhodamine)-labeled ATP aptamer (TC/Apt).55 TC in the TC/Apt shows superior quenching efficiency for ROX (e.g., ∼97%). In the presence of ATP, the ROX-tagged aptamer is released from the TC surface, resulting in the recovery of ROX fluorescence under 545 nm excitation (Fig. 7). Through this procedure, the researchers successfully obtained the fluorescence detection and imaging of ATP in living cells, body fluids (e.g., urine and serum), and a mouse tumor model.
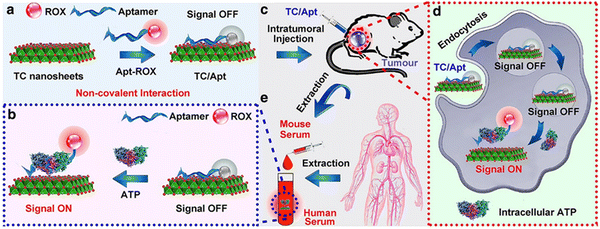 |
| Fig. 7 Scheme of TC/Apt fluorescent probes. (a) Schematic diagram of fabrication of TC/Apt-based fluorescent probes. (b) TC/Apt-based fluorescent probes for the accurate detection of ATP based on a signal off/on switch mechanism. (c) TC/Apt-based fluorescent probes for in vivo imaging of ATP in tumors of a mouse model. (d) TC/Apt-based fluorescent probes for imaging analysis of intracellular ATP in live cells. (e) TC/Apt-based fluorescent probes for quantitative detection of ATP in real samples, such as mouse and human serum. Reproduced with permission.55 Copyright 2021, Biomedcentral. | |
Deng et al. reported the imaging of subcellular mitochondria and mitochondrial ATP in living cells56 targeted by nano-ZIF-90 that self-assembled from Zn2+ and imidazole-2-carboxyaldehyde (2-ICA). After ATP triggering the breakdown of ZIF-90, the encapsulation of fluorescent Rhodamine B (RhB) into ZIF-90 inhibits the emission of RhB. The release of RhB for ATP induction is caused by a competitive coordination between ATP and the metal nodes of ZIF-90 (Fig. 8). The electrostatic interaction between the positively charged ZIF-90 and the negatively charged mitochondria inner membrane facilitates the accumulation of RhB/ZIF-90 within mitochondria. This method allows for imaging mitochondrial ATP in living cells and studying fluctuations in ATP levels during glycolysis and apoptosis.
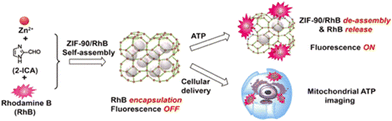 |
| Fig. 8 Schematic representation of modulating the host–guest chemistry of nanoscale RhB/ZIF-90 for fluorescent ATP sensing and mitochondrial ATP imaging. Reproduced with permission.56 Copyright 2017, American Chemical Society. | |
In addition, graphene quantum dots (GQDs), as a new class of carbon-based quantum dots, have unique properties such as stable photoluminescence, low toxicity and good biocompatibility. It can effectively avoid the limitation of poor luminescence stability of organic probes and has a great potential for mitochondrial targeted imaging. Liu et al. synthesized single-layered graphene quantum dots (s-GQDs) with yellow fluorescence for ATP imaging in living cells.57 Macrocyclic aromatic conjugated structure and positively charged sites in s-GQDS can achieve specific recognition of ATP/GTP and good mitochondrial targeting ability. The results showed that s-GQDS successfully monitored the ATP fluctuation in HeLa cells after Ca2+ and sodium azide treatment, respectively. This study offers an effective solution to monitor mitochondrial ATP fluctuations caused by ATP activation and inhibition in active cells. In other words, s-GQDs provide a new platform for studying cell metabolism diseases and mitochondrial dysfunction conditions.
3.3 H2O2 targeting imaging
As an important member of intracellular active species, hydrogen peroxide is one of the key by-products of energy metabolism. The physiological levels of hydrogen peroxide act as a molecular messenger and provide essential information for the regulation of intracellular processes, such as immune cell activation and vascular remodeling in mammals. There has been a link established between high levels of hydrogen peroxide and major diseases such as malignant tumors, neurodegenerative Parkinson's disease and Alzheimer's syndrome. Therefore, it is of great significance to detect the concentration levels of hydrogen peroxide in mitochondria for the prevention, diagnosis and pathological studies of certain diseases. The ratiometric fluorescence technique based on the fluorescence resonance energy transfer (FRET) mechanism is highly accurate and shows significantly low interference, thus enabling real-time detection and imaging of hydrogen peroxide in mitochondria. Wu and coworkers designed a multifunctional fluorescent nanoprobe for detecting mitochondrial H2O2.58 A mitochondria-targeting ligand (TPP) and an H2O2 recognition element (PFl) are covalently linked onto carbon dots to construct the nanoprobe (CDs). Mito-CD-PF1 is non-cytotoxic and easily loaded into cells for ratiometric detection of mitochondrial H2O2 variations in the presence of exogenous or endogenous H2O2 (Fig. 9). This work offers some important insights into the design and construction of fluorescence detection and imaging system at the subcellular level. Another example of FRET based imaging was presented by Niu and Qiao.59 In their work, the ratiometric detection of mitochondrial H2O2 in live cells was successfully realized by the introduction of a self-assembled polymeric micelle that carried tetraphenylethene (TPE), fluorescent boronate and triphenylphosphonium as the donor, a H2O2-responsive acceptor, and a mitochondria-targeted moiety, respectively. To further extend the study, one can incorporate multi-functional molecules into the nanoprobes to enable them to be accessible for targeting, sensing, and energy transfer.
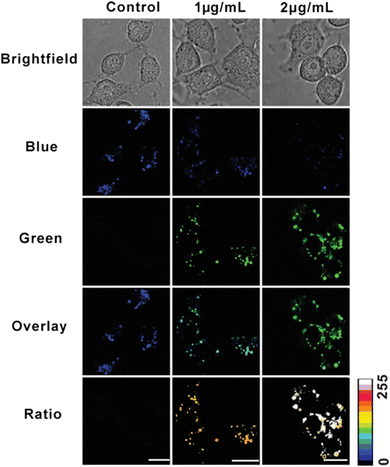 |
| Fig. 9 Confocal fluorescence images of Mito-CD-PF1-stained Raw 264.7 cells upon stimulation by PMA with varied concentrations. Columns from left to right: control (cells with no PMA), cells treated with 1 μg mL−1 and 2 μg mL−1 PMA. Rows from top to bottom: brightfield channel, blue channel, green channel, overlay of blue and green channel, and ratio image, respectively. Concentration of Mito-CD-PF1 in culture media: 0.15 mg mL−1. Images were acquired under 405 nm excitation and fluorescent emission windows: blue = 425–475 nm, green = 500–550 nm. Scale bar: 15 μm. Reproduced with permission.58 Copyright 2013, Wiley-VCH GmbH. | |
3.4 H2S targeting imaging
Hydrogen sulfide (H2S) is a multipurpose regulator in cells and is involved in a variety of diseases caused by mitochondrial dysfunction.60,61 As the major metabolic locus of H2S in organisms,62 the concentration change of H2S indirectly reflects the function of mitochondria. Additionally, the active chemical properties of H2S can also be used as a medium for mitochondrial imaging and reflect the physiological and pathological states of mitochondria. Therefore, various imaging probes have been developed in order to evaluate the function of mitochondria through monitoring the level of H2S in mitochondria.63–65 Currently, the organic molecule probes used for mitochondrial H2S imaging are not suitable for long-term imaging in vivo for multiple reasons. First, the organic molecule probes cannot be effectively penetrated by excitation irradiation. Second, they exhibit short blood circulation time. Last, they easily bind with proteins before even reaching target cells. To address these problems, there have been a growing number of reports that apply upconversion nanoparticles (UCNPs) in the field of bioimaging, owing to their unique properties of negligible photobleaching, deep penetration depth, and high resistance to autofluorescence interference.66 In 2018, Huang et al. developed a lysosome-assisted mitochondrial targeting probe based on triphenylamine-merocyanine (TPAMC) modified UCNPs (TPAMC-UCNPs@PHIS-PEG) with an acid-activated targeting strategy for ratiometric detection and imaging of H2S in mitochondria.67 In the imaging system, the absorption spectra of TPAMC matched well with the green upconversion luminescence (UCL), resulting in a strong luminescence resonance energy transfer (LRET) from UCNPs to TPAMC. Thus, only a weak UCL signal in the green channel was observed for TPAMC-UCNPs@PHIS-PEG nanoparticles under 980 nm excitation. In mitochondria, TPAMC on the surface of nanoparticles reacts with H2S, and its adsorption decreases sharply, leading to the recovery of green UCL. In order to improve the stability of the nanoprobe in blood circulation, a poly(ethylene glycol) (PEG) shell composed of 1,2-distearoyl-sn-glycero-3-phosphoethanolamine-N-[methoxy-(poly(ethylene glycol))-2000] (mDSPE-PEG) and poly(L-histidine)-b-PEG (PHIS-PEG) were further coated on the surface of TPAMC-UCNPs. PEG shells reduce the possibility that TPAMC-UCNPs will adhere to biomolecules and endow it with acid-activated properties (Fig. 10). In vitro and in vivo experiments indicated that TPAMC-UCNPs@PHIS-PEG nanoprobe was able to be used not only for ratiometric UCL imaging of mitochondrial H2S generation in living cells, but also for monitoring mitochondrial H2S levels in colon cancer mouse models.
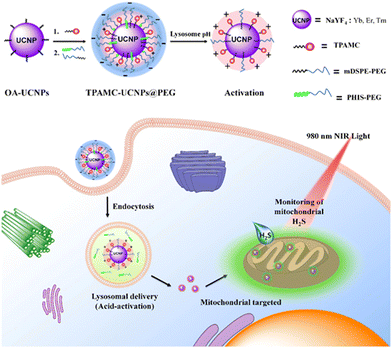 |
| Fig. 10 Design of TPAMC-UCNPs@PEG, and the illustration of lysosome-assisted mitochondrial targeting process and UCL monitoring of mitochondrial H2S in cells. Reproduced with permission.67 Copyright 2018, American Chemical Society. | |
3.5 Other mitochondrial microenvironment targeting imaging
In the above sections, we have reviewed the development of study in mitochondrial microenvironments by targeting matrix, ATP, H2O2, and H2S. In this section, we will focus on nanoprobes that target other microenvironmental factors, such as pH and peroxynitrite. Xu and Zhou prepared a nanoprobe by covalently linking a mitochondria-targeted ligand (triphenylphosphonium, TPP) and a pH recognition fluorescence indicator (rhodamine, RhB) onto the surface of MoS2 quantum dots (QDs). MoS2 QDs serve as a fluorescent reference for the ratiometric signal as well as a nanocarrier for the targeted ligand and pH fluorescent indicator in this multifunctional fluorescent nanoprobe. The pH-sensitive fluorescence of the RhB-based group was compared to the pH-resistant fluorescence of MoS2 QDs for ratiometric pH detection. After absorption in live cells, the nanoprobe was able to label mitochondria with great precision, allowing for imaging and pH monitoring.68 Peroxynitrite (ONOO−) is a group of biological oxidants that play a role in both physiological and pathological processes. It has proved difficult to detect ONOO− in biological systems due to its exceptionally short half-life and low steady-state concentration. Zhao and Yang prepared a ratiometric fluorescent nanoprobe for ONOO− by covalently connecting graphene quantum dots (GQDs) with cyanine 5.5 (Cy5.5). This nanoprobe (GQD-Cy5.5) has two main fluorescence emission bands that peak at 520 and 694 nm, respectively. In the presence of ONOO−, the intensity of 520 nm centered emission increased whereas that of 694 nm centered emission decreased. The fluorescence intensity ratio of the two emission bands demonstrated a strong linear relationship with ONOO− concentration.69 These unique luminescent properties make the studied nanoprobe, a great tool to image endogenous ONOO− in cell mitochondria using ratiometric fluorescence imaging.
3.6 Mitochondrial micro RNA targeting imaging
Mitochondrial DNA (mtDNA) has 16.5 kb base pairs and encodes 13 complexes, and it plays an important role in regulation of immune responses.70–72 There is only one type of mtDNA in an organism, and mtDNA possesses a unique structural characteristic, an extremely small double-stranded circular structure. However, most of the mtDNA probes are small molecules, which were proved to have low photostability and broad emission bands. And there have been very few reported imaging probes and techniques constructed by nanotechnology. Therefore, it's highly necessary to develop more ideal imaging probes, especially with the aid of mitochondrial targeting microRNA (mitomiR) that can affect mitochondrial morphology, metabolism, redox homeostasis, autophagy and apoptosis by regulating the mitochondrial gene expression. The abnormal expression of mitomiR is closely related to metabolic diseases, cardiovascular diseases, neurodegenerative diseases and tumors. Studies have found that mitomiR is involved in chemotherapy tolerance, cancer metastasis, and recurrence. Therefore, the precise in situ imaging of mitomiR is of great significance for exploring the physiological and pathological functions of mitomiR and disease diagnosis. Li and coworkers presented a study that makes an original contribution to mitochondrial microRNA imaging.73 Nanoreporters based on the combination of PD (photocleavable linker into a designed DNA sensor probe) and UCNPs were synthesized using the mitochondria targeting strategy. Upconverted NaGdF4:Yb3+,Tm3+ core–shell nanocomposites efficiently convert NIR light to ultraviolet (UV) light. During the targeting procedure, the DNA nanoreporters could be localized to mitochondria in live cells with blocked sensing function. Then, the UV light-activatable PD sensing function could be triggered noninvasively by the UV emission emitted by UCNPs upon NIR excitation at a certain time and location. To be specific, the UV emission triggers strand displacement reactions (SDRs), thus ensuring accurate mitomiR imaging at a specific location in suborganelles.
4. Conclusion and perspectives
Mitochondria are the main energy supply organelles in cells, the main source of intracellular reactive oxygen species, and one of the regulatory hubs of cell apoptosis. It has now been demonstrated that the dynamic changes in the mitochondrial structure and function, as well as the microenvironment in mitochondria can cause cellular energy disturbances and oxidative damage, which may result in neurodegenerative diseases, metabolic problems, cardiovascular diseases and others. Mitochondrial imaging is a useful tool to track the changes in the mitochondrial internal structure and monitor the performance of mitochondria. Used in one of the three main techniques that are used for mitochondria targeted imaging, the small molecule fluorescent probes are easy to use, water-soluble, and can be passively transported across the cell membrane and directly aggregated on active mitochondria. However, one disadvantage regarding this technique is that the performance of small molecule fluorescent probes strongly depends on mitochondrial membrane potential. The probes will fall off from mitochondria if the mitochondrial membrane potential is lost. This finding significantly limits their use and application in tracking the environmental changes in mitochondria. In contrast, nanomaterials exhibit unique properties of robust photobleaching resistance and great photostability. More importantly, they enable us to detect the changes in mitochondrial microenvironment, making them a promising solution for mitochondrial imaging.
It is also noted that more advanced microscopy techniques make a crucial impact on the development of the mitochondria imaging. The cristae of mitochondria can only be observed by simultaneously applying novel photostable mitochondrial probes and state-of-the-art microscopic imaging techniques such as Hessian SIM, STED superresolution microscopy and algorithm-assisted super-resolution imaging. Despite the fact that there has been a rapid development of mitochondria targeted imaging by using fluorescent nanoprobes, there are several limitations that need further research and study. First, nanoprobes usually require complicated sample preparation processes. Second, the broad and visible only luminescence spectra result in strong background signal and weak signal-to-noise ratio. Therefore, it is necessary to develop new probes with a narrow luminescence interval, strong photostability and good biocompatibility, which would be of far-reaching significance to the understanding of mitochondria related diseases and the development of mitochondria targeting drugs.
Author contributions
All authors (N. Z., Q. W., S. Z., C. M., L. H., and S. L.) contributed to writing, reviewing and editing of this work and consented to the final version. All authors have read and agreed to the published version of the manuscript.
Funding
This work was supported by the National Science Fund for Distinguished Young Scholars (No. 51825202), the National Natural Science Foundation of China (U20A20339) and the Fundamental Research Funds for the Central Universities (AUGA5710052421).
Conflicts of interest
The authors declare no conflict of interest.
References
- D. J. Pagliarini and J. Rutter, Genes Dev., 2013, 27, 2615–2627 CrossRef CAS PubMed.
- J. B. Spinelli and M. C. Haigis, Nat. Cell Biol., 2018, 20, 745–754 CrossRef CAS PubMed.
- J. Nunnari and A. Suomalainen, Cell, 2012, 148, 1145–1159 CrossRef CAS PubMed.
- S. B. Vafai and V. K. Mootha, Nature, 2012, 491, 374–383 CrossRef CAS PubMed.
- H. Wang, B. Fang, B. Peng, L. Wang, Y. Xue, H. Bai, S. Lu, N. H. Voelcker, L. Li, L. Fu and W. Huang, Front. Chem., 2021, 9, 683220 CrossRef CAS PubMed.
- Y. Chu, J. Park, E. Kim and S. Lee, Materials, 2021, 14, 4180 CrossRef CAS PubMed.
- J. Yang, Y. Guo, M. Pistolozzi and J. Yan, Dyes Pigm., 2021, 193, 109466 CrossRef CAS.
- C. Ma, F. Xia and S. O. Kelley, Bioconjugate Chem., 2020, 31, 2650–2667 CrossRef CAS PubMed.
- H. Yuan, H. Cho, H. H. Chen, M. Panagia, D. E. Sosnovik and L. Josephson, Chem. Commun., 2013, 49, 10361–10363 RSC.
- C. Zhang, T. Liu, Y. Su, S. Luo, Y. Zhu, X. Tan, S. Fan, L. Zhang, Y. Zhou, T. Cheng and C. Shi, Biomaterials, 2010, 31, 6612–6617 CrossRef CAS PubMed.
- F. Miao, W. Zhang, Y. Sun, R. Zhang, Y. Liu, F. Guo, G. Song, M. Tian and X. Yu, Biosens. Bioelectron., 2014, 55, 423–429 CrossRef CAS PubMed.
- A. Martin, A. Byrne, C. S. Burke, R. J. Förster and T. E. Keyes, J. Am. Chem. Soc., 2014, 136, 15300–15309 CrossRef CAS PubMed.
- V. Jayashankar and S. M. Rafelski, Curr. Opin. Cell Biol., 2014, 26, 34–40 CrossRef CAS PubMed.
- T. G. Frey and C. A. Mannella, Trends Biochem. Sci., 2000, 25, 319–324 CrossRef CAS PubMed.
- C. R. Schiavon, T. Zhang, B. Zhao, A. S. Moore, P. Wales, L. R. Andrade, M. Wu, T. C. Sung, Y. Dayn, J. W. Feng, O. A. Quintero, G. S. Shadel, R. Grosse and U. Manor, Nat. Methods, 2020, 17, 917–921 CrossRef CAS PubMed.
- B. Lin, Y. Liu, X. Zhang, L. Fan, Y. Shu and J. Wang, ACS Sens., 2021, 6, 4009–4018 CrossRef CAS PubMed.
- L. Zhang, J. L. Wang, X. X. Ba, S. Y. Hua, P. Jiang, F. L. Jiang and Y. Liu, ACS Appl. Mater. Interfaces, 2021, 13, 7945–7954 CrossRef CAS PubMed.
- D. Ma, Q. Zong, Y. Du, F. Yu, X. Xiao, R. Sun, Y. Guo, X. Wei and Y. Yuan, Acta Biomater., 2021, 135, 628–637 CrossRef CAS PubMed.
- J. Wu, C. Han, X. Cao, Z. Lv, C. Wang, X. Huo, L. Feng, B. Zhang, X. Tian and X. Ma, Anal. Chim. Acta, 2022, 1199, 339573 CrossRef CAS PubMed.
- J. Huang, D. Hong, W. Lang, J. Liu, J. Dong, C. Yuan, J. Luo, J. Ge and Q. Zhu, Analyst, 2019, 144, 3703–3709 RSC.
- A. T. Hoye, J. E. Davoren, P. Wipf, M. P. Fink and V. E. Kagan, Acc. Chem. Res., 2008, 41(1), 87–97 CrossRef CAS PubMed.
- G. Zhang, Y. Sun, X. He, W. Zhang, M. Tian, R. Feng, R. Zhang, X. Li, L. Guo, X. Yu and S. Zhang, Anal. Chem., 2015, 87(24), 12088–12095 CrossRef CAS PubMed.
- S. Jakobs, Biochim. Biophys. Acta, Mol. Cell Res., 2006, 1763(5), 561–575 CrossRef CAS PubMed.
- B. A. D. Neto, J. R. Corrêa and R. G. Silva, RSC Adv., 2013, 3(16), 5291–5301 RSC.
- A. Rathinavelu, K. Alhazzani, S. Dhandayuthapani and T. Kanagasabai, Tumor Biol., 2017, 39, 1010428317726841 CrossRef PubMed.
- M. V. Dubinin, A. A. Semenova, A. I. Ilzorkina, N. V. Penkov, D. A. Nedopekina, V. A. Sharapov, E. I. Khoroshavina, E. V. Davletshin, N. V. Belosludtseva, A. Y. Spivak and K. N. Belosludtsev, Free Radical Biol. Med., 2021, 168, 55–69 CrossRef CAS PubMed.
- P. Sancho, E. Galeano, E. Nieto, M. D. Delgado and A. I. Garcia-Perez, Leuk. Res., 2007, 31, 969–978 CrossRef CAS PubMed.
- Y. F. Song, D. Z. Liu, Y. Cheng, M. Liu, W. L. Ye, B. L. Zhang, X. Y. Liu and S. Y. Zhou, Sci. Rep., 2015, 5, 16125 CrossRef CAS PubMed.
- L. V. Johnson, M. L. Walsh and L. B. Chen, Proc. Natl. Acad. Sci. U. S. A., 1980, 77, 990–994 CrossRef CAS PubMed.
- R. L. Pan, W. Q. Hu, J. Pan, L. Huang, C. C. Luan and H. M. Shen, Neural Regener. Res., 2020, 15, 1086–1093 CrossRef PubMed.
- S. A. Dyshlovoy, E. K. Kudryashova, M. Kaune, T. N. Makarieva, L. K. Shubina, T. Busenbender, V. A. Denisenko, R. S. Popov, J. Hauschild, S. N. Fedorov, C. Bokemeyer, M. Graefen, V. A. Stonik and G. von Amsberg, Sci. Rep., 2020, 10, 9764 CrossRef CAS PubMed.
- F. Auzel, Chem. Rev., 2004, 104(1), 139–174 CrossRef CAS PubMed.
- M. Haase and H. Schäfer, Angew. Chem., Int. Ed., 2011, 50(26), 5808–5829 CrossRef CAS PubMed.
- C. Liu, B. Liu, J. Zhao, Z. Di, D. Chen, Z. Gu, L. Li and Y. Zhao, Angew. Chem., Int. Ed., 2020, 59(7), 2634–2638 CrossRef CAS PubMed.
- X. Qian, Z. Gu and Y. Chen, Mater. Horiz., 2017, 4(5), 800–816 RSC.
- Z. Sun, H. Xie, S. Tang, X.-F. Yu, Z. Guo, J. Shao, H. Zhang, H. Huang, H. Wang and P. K. Chu, Angew. Chem., Int. Ed., 2015, 54(39), 11526–11530 CrossRef CAS PubMed.
- J. Shao, H. Xie, H. Huang, Z. Li, Z. Sun, Y. Xu, Q. Xiao, X.-F. Yu, Y. Zhao, H. Zhang, H. Wang and P. K. Chu, Nat. Commun., 2016, 7(1), 12967 CrossRef CAS PubMed.
- X. Yang, D. Wang, J. Zhu, L. Xue, C. Ou, W. Wang, M. Lu, X. Song and X. Dong, Chem. Sci., 2019, 10(13), 3779–3785 RSC.
- Q. Zhuang, H. Jia, L. Du, Y. Li, Z. Chen, S. Huang and Y. Liu, Biosens. Bioelectron., 2014, 55, 76–82 CrossRef CAS PubMed.
- F. Yu, Y. Shao and X. Chai,
et al.
, Angew. Chem., Int. Ed., 2022, 61, e202203238 CAS.
- P. C. Saha, T. Bera, T. Chatterjee, J. Samanta, A. Sengupta, M. Bhattacharyya and S. Guha, Bioconjugate Chem., 2021, 32, 833–841 CrossRef CAS PubMed.
- Y. Cheng, F. Yang, K. Zhang, Y. Zhang, Y. Cao, C. Liu, H. Lu, H. Dong and X. Zhang, Adv. Funct. Mater., 2019, 29, 1903850 CrossRef CAS.
- X. Wu, S. Sun, Y. Wang, J. Zhu, K. Jiang, Y. Leng, Q. Shu and H. Lin, Biosens. Bioelectron., 2017, 90, 501–507 CrossRef CAS PubMed.
- O. Oladimeji, J. Akinyelu, A. Daniels and M. Singh, Int. J. Mol. Sci., 2021, 22, 5072 CrossRef CAS PubMed.
- B. Mta, L. Kai and B. Mr,
et al., A mitochondria-targeting lipid-small molecule hybrid nanoparticle for imaging and therapy in an orthotopic glioma model, Acta Pharm. Sin. B, 2022, 12(6), 2672–2682 CrossRef PubMed.
- G. Gao, Y.-W. Jiang, J. Yang and F.-G. Wu, Nanoscale, 2017, 9(46), 18368–18378 RSC.
- C. Gui, E. Zhao, R. T. K. Kwok, A. C. S. Leung, J. W. Y. Lam, M. Jiang, H. Deng, Y. Cai, W. Zhang, H. Su and B. Z. Tang, Chem. Sci., 2017, 8(3), 1822–1830 RSC.
- Q. Hu, M. Gao, G. Feng and B. Liu, Angew. Chem., Int. Ed., 2014, 53(51), 14225–14229 CrossRef CAS PubMed.
- M. H. Chen, F. X. Wang, J. J. Cao, C. P. Tan, L. N. Ji and Z. W. Mao, ACS Appl. Mater. Interfaces, 2017, 9, 13304–13314 CrossRef CAS PubMed.
- A. M. Aleardi, G. Benard, O. Augereau, M. Malgat, J. C. Talbot, J. P. Mazat, T. Letellier, J. Dachary-Prigent, G. C. Solaini and R. Rossignol, J. Bioenerg. Biomembr., 2005, 37, 207–225 CrossRef CAS PubMed.
- D. V. Kolygina, M. Siek, M. Borkowska, G. Ahumada, P. Barski, D. Witt, A. Y. Jee, H. Miao, J. C. Ahumada, S. Granick, K. Kandere-Grzybowska and B. A. Grzybowski, ACS Nano, 2021, 15, 11470–11490 CrossRef CAS PubMed.
- X. Li, X. Guo, L. Cao, Z. Xun, S. Wang, S. Li, Y. Li and G. Yang, Angew. Chem., Int. Ed., 2014, 53, 7809–7813 CrossRef CAS PubMed.
- J. L. Tang, C. Y. Li, Y. F. Li and C. X. Zou, Chem. Commun., 2014, 50, 15411–15414 RSC.
- K. Y. Tan, C. Y. Li, Y. F. Li, J. Fei, B. Yang, Y. J. Fu and F. Li, Anal. Chem., 2017, 89, 1749–1756 CrossRef CAS PubMed.
- B. Chu, A. Wang, L. Cheng, R. Chen, H. Shi, B. Song, F. Dong, H. Wang and Y. He, J. Nanobiotechnol., 2021, 19, 187 CrossRef CAS PubMed.
- J. Deng, K. Wang, M. Wang, P. Yu and L. Mao, J. Am. Chem. Soc., 2017, 139, 5877–5882 CrossRef CAS PubMed.
- J. H. Liu, R. S. Li, B. Yuan, J. Wang, Y. F. Li and C. Z. Huang, Nanoscale, 2018, 10, 17402–17408 RSC.
- F. Du, Y. Min, F. Zeng, C. Yu and S. Wu, Small, 2014, 10, 964–972 CrossRef CAS PubMed.
- J. Qiao, Z. Liu, Y. Tian, M. Wu and Z. Niu, Chem. Commun., 2015, 51, 3641–3644 RSC.
- C. Szabo, Nat. Rev. Drug Discovery, 2007, 6, 917–935 CrossRef CAS PubMed.
- D. J. Lefer, Proc. Natl. Acad. Sci. U. S. A., 2007, 104, 17907–17908 CrossRef CAS PubMed.
- W. Guo, J. T. Kan, Z. Y. Cheng, J. F. Chen, Y. Q. Shen, J. Xu, D. Wu and Y. Z. Zhu, Oxid. Med. Cell. Longevity, 2012, 2012, 878052 Search PubMed.
- S. K. Bae, C. H. Heo, D. J. Choi, D. Sen, E.-H. Joe, B. R. Cho and H. M. Kim, J. Am. Chem. Soc., 2013, 135, 9915–9923 CrossRef CAS PubMed.
- X. Wang, J. Sun, W. Zhang, X. Ma, J. Lv and B. Tang, Chem. Sci., 2013, 4, 2551–2556 RSC.
- Y. Chen, C. Zhu, Z. Yang, J. Chen, Y. He, Y. Jiao, W. He, L. Qiu, J. Cen and Z. Guo, Angew. Chem., Int. Ed., 2013, 52, 1688–1691 CrossRef CAS PubMed.
- S. Wen, J. Zhou, K. Zheng, A. Bednarkiewicz, X. Liu and D. Jin, Nat. Commun., 2018, 9, 2415 CrossRef PubMed.
- X. Li, H. Zhao, Y. Ji, C. Yin, J. Li, Z. Yang, Y. Tang, Q. Zhang, Q. Fan and W. Huang, ACS Appl. Mater. Interfaces, 2018, 10, 39544–39556 CrossRef CAS PubMed.
- S. Xu, G. Zheng and K. Zhou, Anal. Biochem., 2022, 640, 114545 CrossRef CAS PubMed.
- K. Yang, L. Hou, Z. Li, T. Lin, J. Tian and S. Zhao, Talanta, 2021, 231, 122421 CrossRef CAS PubMed.
- K. Ishikawa, K. Takenaga, M. Akimoto, N. Koshikawa, A. Yamaguchi, H. Imanishi, K. Nakada, Y. Honma and J. Hayashi, Science, 2008, 320, 661–664 CrossRef CAS PubMed.
- K. McArthur, L. W. Whitehead, J. M. Heddleston, L. Li, B. S. Padman, V. Oorschot, N. D. Geoghegan, S. Chappaz, S. Davidson, H. San Chin, R. M. Lane, M. Dramicanin, T. L. Saunders, C. Sugiana, R. Lessene, L. D. Osellame, T. L. Chew, G. Dewson, M. Lazarou, G. Ramm, G. Lessene, M. T. Ryan, K. L. Rogers, M. F. van Delft and B. T. Kile, Science, 2018, 359, 883–895 CrossRef CAS PubMed.
- K. Gao, M. Cheng, X. Zuo, J. Lin, K. Hoogewijs, M. P. Murphy, X. D. Fu and X. Zhang, Cell Res., 2021, 31, 219–228 CrossRef CAS PubMed.
- J. Zhao, Z. Li, Y. Shao, W. Hu and L. Li, Angew. Chem., Int. Ed., 2021, 60, 17937–17941 CrossRef CAS PubMed.
|
This journal is © The Royal Society of Chemistry 2022 |
Click here to see how this site uses Cookies. View our privacy policy here.