DOI:
10.1039/D2TB00709F
(Review Article)
J. Mater. Chem. B, 2022,
10, 7161-7172
Cell membrane-engineered nanoparticles for cancer therapy
Received
31st March 2022
, Accepted 13th May 2022
First published on 26th May 2022
Abstract
Cell membrane-coating nanotechnology involves dressing synthetic nanoparticles (NPs) with membranes derived from different types of cells to endow the NPs with the properties of a specific cell type and to further achieve precise and effective disease treatment. Of great interest is the fact that cell membranes sourced from different cell sources can give different functions to the NPs. In this review, we comprehensively lay out the major advantages of several typical cell membranes, which are derived from red blood cells (RBCs), platelets, neutrophils, immune cells, stem cells, or cancer cells, for the selective fabrication of novel nanotherapeutics and their potential to greatly advance the development of nanomedicine and improve the curative effect of cancer treatments.
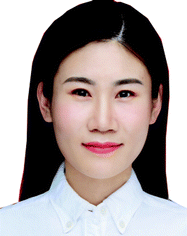
Wenjing Liu
| Wenjing Liu received her PhD from the University of Chinese Academy of Sciences in 2019. Currently, she works in the department of the Key Laboratory of Drug Resistance Tuberculosis Research (Beijing Chest Hospital, Capital Medical University). Her recent research interests include the design, modification, in vivo delivery, and efficacy assessment of biomaterial- and nanomaterial-based nanoantibacterial agents. |
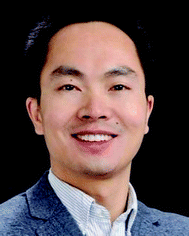
Yuanyu Huang
| Dr Yuanyu Huang received his PhD in Biotechnology from Peking University in 2013. After 3 years of postdoctoral training, he joined the Beijing Institute of Technology to establish his group in 2016. He was promoted to a tenured associate professor in 2020 and a full professor in 2022. Dr Huang's primary research interest focuses on the development of nucleic acid therapeutics, including designing and optimizing nucleic acid molecules (siRNA, mRNA, etc.), exploring innovative drug delivery systems and their translational medicine applications in the management of diverse diseases. He has published >80 peer-reviewed journal articles, >10 book chapters and filed >15 patents. |
1. Introduction
Cancer ranks as the main cause of death and the overriding obstacle to extending people's life expectancy in all countries around the world.1 At present, treatment options such as surgery, chemotherapy, and radiotherapy that have been put into clinical application still face problems such as extensive side effects, low targeting ability, and poor therapeutic outcomes.2 Despite the fact that many scholars have conducted in-depth research on the mechanism and treatment of cancer for decades, the extraordinary prevalence and increasing burden of cancer pose a challenge for finding effective cancer treatments. Nanotechnology has been extensively explored in the past few decades, and was supposed to be an exciting and promising way to treat cancer. In particular, NP-based targeted delivery systems have attracted widespread attention and deep research3 mainly because NPs show many prominent advantages in cancer treatment. First, NPs can accumulate passively and preferentially at tumor sites characterized by leaky vasculature through the well-known enhanced permeability and retention (EPR) effect of solid tumors.4 Second, owing to the presence of diverse functional groups, the surfaces of NPs can be easily introduced with proteins, peptides, and other biomolecules to reduce nonspecific uptake by the reticuloendothelial system (RES) and further specifically bind to overexpressed tumor cell receptors for enhanced accumulation.5 Third, the specific electric potential and large specific surface area of NPs efficiently entrap high payloads of drugs, genes, and other therapeutic molecules and protect them from enzymatic degradation in the complex physiological microenvironment.6 Compared with other vectors, they have higher transport efficiency across cell membranes. Moreover, functionalized NPs are able to control the release of the loaded drug molecules via diverse internal and external stimuli (e.g., pH, GSH, light, ultrasound),7 which helps prevent premature drug leakage in healthy tissues and mitigates the potential side effects. In addition, NPs usually have good biocompatibility and even biodegradability, so they have little impact on cell growth and metabolism. In this regard, ever-increasing research studies have turned to utilizing various strategies to optimally design high-performance NPs, which may substantially improve their therapeutic effectiveness.8
However, the currently explored synthetic NP delivery system still has many shortcomings, which limit its clinical application. Once the NPs enter the human body, they encounter a highly complex environment that is inherently good at identifying and eliminating foreign objects. For example, there are many protein and cell-based components in the blood. Contact with any of them will quickly impair NP performance and reduce their circulation in blood.9 Absorption by the RES before the NPs reach their targets is also one of the main obstacles that almost all platforms must overcome.10 In addition to reducing non-specific recognition and alleviating immune clearance, the key to improving treatment efficacy is the addition of specific tumor targeting and bioadhesive properties, which can promote their preferential accumulation at the site of interest, improve their binding to tumors, and maintain long enough adhesion time to kill a large number of cancer cells.
Traditional surface modification strategies through physical absorption and chemical bonding can give NPs a certain degree of targeting properties and long-cycle performance. Taking the surface functionalization of nano-quantum dots (QDs) as an example, the use of ligand exchange can provide QDs with additional properties such as solubility, mobility, and targeting. The surface silanization of QDs can increase the solubility of QDs in aqueous media while retaining their emission properties. There are other modification methods, such as amphiphilic binding and the adsorption of biological macromolecules or functional molecules, that can improve the performance of NPs themselves and facilitate their application in disease monitoring and treatment.11 Additionally, one of the greatest advantages of surface-functionalized NPs is that they can be produced on a large scale. However, surface functionalized ligands via physical absorption easily come away from NPs in circulation owing to changes in the NP properties induced by complicated physiological environments.12 Functionalized ligands on NPs by chemical bonding may introduce organic solvents that are hazardous to the body. Furthermore, traditional surface modification generally requires modifiable groups on the surface of NPs, limiting the effects of universal modifications. To make matters worse, some surface modifications may cause dysfunction and unexpected immune responses. For example, surface modification of polyethylene glycol (PEG) is the current gold standard for long-term circulation of NPs,13 and it has been proven that PEGylated NPs will cause an immunological response in the body and can be rapidly cleared after the first dose.14 Therefore, bridging the gap between synthetic NPs and biological entities to obtain the desired drug biodistribution and acceptable therapeutic effects remains a challenge.
Very recently, confronted with these problems, scientists have discovered that using cell membranes to functionalize NPs can endow NPs with more properties, such as, tumor-targeting, immune escape performance, immune programming, excellent biocompatibility, and biological interface performances.15 For example, the RBC membrane can afford NPs their most important characteristic, which is prolonged blood circulation.16 In another case, platelet-coated NPs can achieve immune escape and obtain adhesion to tumors and injured sites.17 Another attractive direction is that NPs coated with immune cell membranes can not only achieve immune escape but also reprogram immune cells to stimulate immune responses and enhance the therapeutic effect.18 More interestingly, cancer cell membrane-coated NPs can provide NPs with homologous targeting.19 In addition, stem cell membrane coatings can also equip NPs with tumor targeting properties.20 Furthermore, hybrid membranes formed by the fusion of different cells can provide more functions for NPs.21 Membrane coating can endow NPs with many properties, but unfortunately, due to technical limitations, membrane-coated NPs cannot be mass-produced yet. In this review, we first summarize the cell membrane extraction and NP coating technologies. Then, we outline the characteristics of different types of cell membranes and their roles in cancer treatment. Finally, the potential challenges and future prospects of cell membranes in cancer treatment are discussed.
2. Cell membrane extraction and coating technology
The first steps for membrane-coated NPs are cell membrane extraction and NP synthesis. The synthetic processes of different NPs are different, and the extraction of the cell membrane has a relatively standardized operating procedure (SOP). The SOP for cell membrane extraction mainly includes cell lysis, mechanical disruption, and ultracentrifugation to remove intracellular components.22 Notably, the extraction procedures differ depending on whether the source cells are nucleus-free. Following the acquisition of the membrane and the inner core nanocarrier, the two materials must be fused together so that the membrane can cover the surface of the core, resulting in cell membrane biomimetic NPs. Currently, there are four fusion methods in use: extrusion, sonication, electroporation, and microfluidics (Fig. 1).
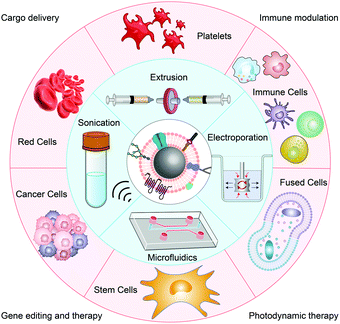 |
| Fig. 1 Schematic illustration of different source-derived cell membranes engineered into NPs by various coating methods for biomedical applications. | |
Extrusion
For cell membrane extrusion, the membrane and inner core nanocarriers can be repeatedly extruded several times through a nanoscale polycarbonate porous membrane using an Avanti micro-extruder. During this extrusion process, the applied mechanical force warps the cell membrane around a substantial range of nanomaterials with varying physical properties and morphologies. Although this method is convenient and effective, it is difficult to achieve large-scale production due to the relatively large material loss caused by material deposition on the filter.16a
Sonication
The sonication approach overcomes the material loss and allows for scaled-up production. Successful coating is often demonstrated following the sonication of a cell membrane and NP mixture, thus preventing the loss of the membrane observed through the extrusion technique. Utilization of the sonication method is reported to yield results indistinguishable from those obtained through the porous membrane extrusion method. It is believed that the cell membrane bilayer is disrupted by the activation energy imparted by the sonication process. There is then a subsequent attraction between the NPs and the cell membrane, followed by the reformation of the often-spherical shape in order to reach a minimum entropy that favors the smallest structures. This sonication approach may not be compatible with some nanocore structures due to the effect of sonication on the size and stability of the materials.23
Electroporation
Cell membrane electroporation relies on the exposure of cell membranes to a strong external electric field, as a result of which multiple pores are created that cause membrane semi-permeability. NPs can diffuse into the cell membrane through these pores. However, the membrane potential may change or fluctuate as a result of this method. This method is becoming increasingly popular among scientists as it does not hinder the stability of NPs by preventing agglomeration.24
Microfluidics
Very recently, a novel microfluidic electroporation technique has been utilized for membrane-coated NP generation. The reported device consists of a Y-shaped merging channel, an S-shaped mixing channel, and an electroporation zone right before the outlet. By fine-tuning the pulse voltage and duration, as well as the flow velocity, high-quality particles can be fabricated. This demonstrates a superior method to mechanical extrusion whereby microfluidic-produced nanocore structures are shown to demonstrate, compared to the conventional method, enhanced colloidal stability and in vivo effects that are indicative of a higher integrity coating. Microfluidic electroporation may play a role in addressing the low throughput of the mechanical extrusion technique.25
Advances in membrane-coating technologies
Effective and continuous membrane attachment is very important for the creation of the membrane-coated NP system. Currently, extrusion and sonication are the main methods for the preparation of membrane-coated NPs, and electroporation and microfluidics are favorable means to prepare stable and uniform membrane-coated NPs. However, these four common methods still have certain limitations, such as cumbersome steps, inability to mass-produce, the dissipation of NPs, and lack of membrane integrity. In recent years, researchers have explored other novel approaches to obtain near-perfect membrane-coated NPs. For example, in the graphene nanoplatform technology, magnetic nanoparticles need to be modified with polyethyleneimine and graphene nanosheets, and are then incubated with target cells until the destructive phospholipids of the cell phospholipid membranes are adsorbed on graphene to obtain membrane-encapsulated biomimetic magnetic nanoparticles.26 Compared with other conventional methods, this technology can integrate membrane extraction and coating, but the process is relatively complicated and the operation technique is difficult. Another promising approach is the in situ encapsulation of cell-derived vesicles. That is, in a serum-free medium, NPs are incubated with cells, and after the cells endocytose the NPs, vesicles containing the NPs will be released under the stimulus of starvation.27 Although this method depends on the endocytosis of NPs by cells, the resulting membrane-coated NPs are able to maintain membrane integrity. This method is expected to be a potential membrane-coated NP technology that can replace extrusion and sonication, and it is also expected that, with the advancement of technology, there will be better membrane-coating technologies for the large-scale production of perfect membrane-coated NP systems.
3. Cell membrane source and its application in cancer therapy
RBC clothes
RBCs are the most prevalent cellular constituents of blood cells in humans owing to their irreplaceable biological function of transporting oxygen through the body. Normally, RBCs have a round cake shape with a convex central concave on both sides, with a diameter of 7–8 μm, and the center of each cell is as thin as 1 μm.28 Furthermore, mature RBCs lack nuclei and organelles and are capable of undergoing changes in shape during blood circulation. Since RBCs can be easily separated from the blood of the contributor, it is very convenient to separate and purify the cell membrane. As a result, RBCs represent a potentially ideal source of cell membranes that are well suited for circulation throughout the blood vessels of patients.29
Other two crucial points regarding why RBCs can be ideal clothes for NPs are their long circulation life of up to 120 days and their ability to evade recognition by the immune system owing to the abundant “self markers” on their membrane surface.30 One of the most common immunomodulatory markers is CD47, and this protein is a transmembrane protein that can be recognized by the RES, thus preventing early uptake by the RES.31 Furthermore, CD47 can emit a “don’t eat me” signal to bypass the uptake by macrophage-like cells and inhibit immune clearance through interactions with the signal regulatory protein-alpha (SIRP-α) receptor.32 In addition, RBCs are completely biodegradable and non-toxic, and their membranes are semi-permeable, which can realize the gradual release of cargo and ensure sustained therapy.33 Based on the above characteristics, RBCs are widely used to camouflage NPs to achieve delivery strategies for various therapeutic agents and change the therapeutic effect of diseases.
Numerous studies have shown that NPs coated with RBC membranes can effectively enhance disease-treatment effects through prolonged circulation time and enhanced immune escape. Zhang's group successfully constructed a biomimetic delivery platform in 2011 using RBC membranes wrapped around biodegradable polymer NPs.16a Importantly, the protein content of this biomimetic platform barely changed during gel electrophoresis and they retained their corresponding functions. Furthermore, their excellent long-circulation properties were confirmed by in vivo experiments (Fig. 2A). This work initially explores the construction of biomimetic carriers with cell membranes as shells. As RBC membrane biomimetic NPs serve as an attractive carrier, more and more researchers have begun to design and construct erythrocyte membrane-based nanocarriers. Green and co-workers at Johns Hopkins University School of Medicine developed a biologically inspired modular multifunctional technology to allow biodegradable polymer NPs to circulate in the blood for a longer period of time.16b It also has a detoxifying effect. The anisotropic material is coated with RBC membranes to form anisotropic membrane-coated NPs, which have enhanced biodistribution, and therapeutic efficacy, and significantly improve the survival rate of sepsis mouse models (Fig. 2B). NPs that encapsulate the RBC membrane can also be further engineered to improve their targeting. Pei et al. used RBC membranes to camouflage MOFs (FeTCPP/Fe2O3) and inserted cholesterol-modified targeting aptamers on the membrane,34 which not only enhanced blood circulation in vivo but also enhanced the enrichment of the MOFs in the tumor domain (Fig. 2C), increasing the therapeutic effects of photodynamic therapy (PDT) and chemodynamic therapy (CDT).
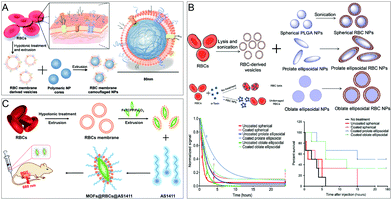 |
| Fig. 2 RBC membrane-coated NPs for treatment. (A) Schematics of the preparation process of the RBC membrane-coated PLGA NPs. Adapted with permission.16a Copyright 2022. National Academy of Sciences. (B) RBC membrane-coated anisotropic NPs for enhanced circulation and toxin removal. Adapted with permission.16b Copyright 2020. American Association for the Advancement of Science. (C) MOFs@RBCs@AS1411 for enhanced PDT and CDT combination therapy. Adapted with permission.34 Copyright 2020. American Chemical Society. | |
Platelet clothes
Platelets are another ubiquitous cellular component in blood derived from megakaryocyte progenitor cells. Usually, platelets are small discoids approximately 1–3 mm in diameter,35 surviving for 7–10 days on average in circulation.36 Platelets are the guardians of the blood circulation that address vascular damage and invasive microorganisms. Additionally, they have the ability to recognize and interact with circulating cancer cells.37 That is why platelets are involved in a wide range of processes, including immunity, wound healing, and tumor metastasis.
Platelet membranes contain unique ligands on their surfaces that can promote subendothelial adhesion,38 pathogen interaction,39 and immune escape.40 Therefore, they have attracted considerable attention in the field of membrane-camouflaged NPs. Specifically, platelet membranes can immune escape through CD47 ligand-mediated macrophage evasion, and can also avoid complement activation-mediated immune escape through CD55/59.23 At the same time, the presence of CD44 and P-selectin receptors on the platelet membranes can also make them combine with circulating tumor cells, making them exceptional tools for cancer targeting.41
Platelet membrane-based NPs are excellent in disease treatment due to their ability to target bacteria, cancer cells, and damaged vasculature. As an example, researchers such as Liangfang Zhang and Ronnie H. Fang of the University of California, San Diego, reported a metal–organic framework (MOF) nano-delivery platform wrapped in platelet cell membranes for the targeted delivery of siRNA in vivo (Fig. 3A).17b Platelet membrane-coating on the surface of the MOFs provides a natural way to make biological contact with disease substrates. This strategy can achieve high silencing efficiency for multiple target genes in vitro. Significant anti-tumor targeting and therapeutic effects have also been observed in mouse xenograft models. Platelet membranes not only target tumors but also interact with the tumor microenvironment. The study directed by Zhang Jie and Ronnie H. Fang used platelet membranes to coat polylactic acid (PLA) NPs loaded with the TLR agonist resiquimod (R848) to form PNP-R848 nanomedicines (Fig. 3B).42 Local administration to solid tumors enhanced local immune activation, resulting in complete tumor regression in a colorectal tumor model and delayed tumor growth and suppressed lung metastasis in an aggressive breast cancer model. In addition, such nanodrugs provide protection against repeated tumor rechallenge. Zhen's group also used platelet membrane-coated NPs for the targeted and site-specific delivery of extracellular active drugs and intracellular functional small molecule drugs to improve anti-tumor efficacy, and these can also effectively eliminate circulating tumor cells in vivo and inhibit tumor metastasis (Fig. 3C).43
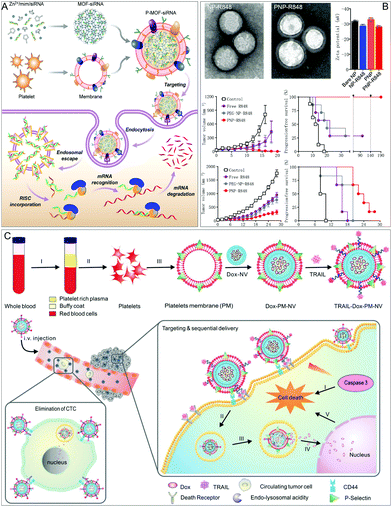 |
| Fig. 3 Platelet membrane-coated NPs for improved disease treatment. (A) Platelet membrane-coated siRNA-loaded MOFs (P-MOF-siRNA) for gene silencing. Adapted with permission.17b Copyright 2020. American Association for the Advancement of Science. (B) PNP-R848 has anti-tumor effects in both colorectal and breast tumors. Adapted with permission.42 Copyright 2021. Springer Nature. (C) TRAIL-Dox-PM-NV captures the CTCs and subsequently triggers TRAIL/Dox-induced cell apoptosis. Adapted with permission.43 Copyright 2015, Wiley-VCH. | |
Immune cell clothes
Neutrophils, macrophages, dendritic cells, T cells, and natural killer cells are commonly used as membrane wraps on immune cells. They form an indispensable part of the immune system and are involved in defending the body against contagious diseases and pathogen invaders. Importantly, the adhesive properties of immune cells enable them to directly associate with tumor cells in the cancer environment or in the bloodstream, and a variety of active recognition receptors on their membrane surfaces have the property of immune system evasion and can cross biological barriers to reach specific areas such as tumors and those with inflammation.44
Neutrophils are the most responsive leukocytes that are recruited to tumors or infectious sites during the inflammatory process, which serves as the first line of defense against infections. Neutrophils have the properties of circulating tumor cells (CTCs) and niche targeting, which is mainly due to the distinct adhesion molecules associated with the membrane of neutrophils.45 Since the half-life span of neutrophils is only 7 hours, it is currently not possible to directly use them as a vehicle for drug delivery. Therefore, Chen et al. used neutrophil-mimicking NPs (NM-NPs) to both target CTCs and the premetastatic niche (Fig. 4A).46 Dual-mode imaging also confirmed the selective targeting efficiency of NM-NPs in the metastasis model. They loaded the protease inhibitor carfilzomib into the NM-NP system, causing selective CTC apoptosis in the blood and preventing nodule formation at an early stage. They verified the apoptosis and inhibitory effects of cancer cells in the metastatic 4T1 model. This biomimetic drug delivery system can effectively prevent and treat cancer.
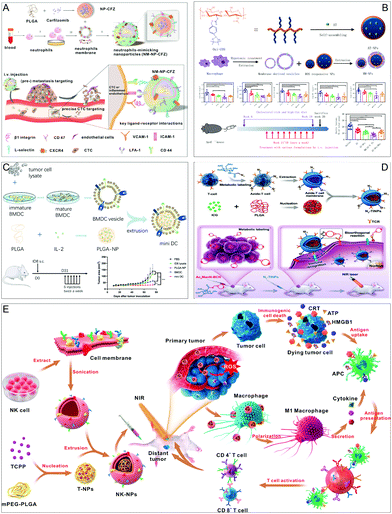 |
| Fig. 4 Membrane-coated NPs from immune cells for potentiating various treatments. (A) The neutrophil-mimicking drug delivery system has higher CTC capture efficiency in vivo. Adapted with permission.46 Copyright 2017. American Chemical Society. (B) Macrophage membrane-encapsulated NPs for improved atherosclerosis therapy. Adapted with permission.18c Copyright 2020. Springer Nature. (C) Dendritic cell membrane-coated NPs exhibit excellent therapeutic and prophylactic efficacy in mouse cancer models. Adapted with permission.49 Copyright 2020. Wiley-VCH. (D) T cell membrane biomimetic NPs for tumor-targeted photothermal therapy. Adapted with permission.50 Copyright 2019. Wiley-VCH. (E) NK cell-membrane-cloaked NPs for PDT-enhanced cell-membrane immunotherapy. Adapted with permission.18b Copyright 2018. American Chemical Society. | |
Macrophages are large and versatile white blood cells that mainly counter tissue damage, bacterial infections, and inflammation. Macrophages are activated and accumulate in lesions, and they can endocytose and digest cell debris and pathogens through toll-like membrane receptors. Macrophages also present tumor-associated antigens (TAA) to T cells and express immunostimulatory cytokines to promote the reproduction and anti-tumor functions of T cells and NK cells in vitro. A large number of macrophages infiltrate the tumor microenvironment, significantly affecting tumor growth, metastasis, and drug treatment. With these characteristics, macrophage membranes that mimic NPs have become a good choice for improving the efficacy of nanomedicine. Ruibing's group exploited the function of the macrophage membrane not only to avoid the clearance of NPs from the RES but also to guide NPs to inflamed tissues and created a biomimetic system of macrophage membrane-encapsulated reactive oxygen species-responsive NPs (MM-NPs) (Fig. 4B).18c The synergistic effect of drug treatment and inflammatory cytokine sequestration by the MM-NP biomimetic system leads to improved therapeutic efficacy in atherosclerosis.
An interesting property of mature macrophages is their ability to polarize in response to environmental cues. The best known and most studied are classically activated macrophages (M1 macrophages) and alternatively activated macrophages (M2 macrophages). M1 macrophages themselves have strong tumoricidal activity, so M1 cell membrane-coated NPs have better tumor targeting. Based on the tumor targeting of M1 cells, Gao's group performed a series of studies using M1 macrophage membranes to coat nanomedicines. In one of their studies, they coated M1 macrophage membranes on the surface of variable-shaped carriers to improve nanodrug circulation, enhance intracellular communication and retention, and realize the multimodal combined therapy of chemotherapy, photodynamic therapy, and immunotherapy to achieve the therapeutic purpose of inhibiting orthotopic breast cancer tumors and lung metastasis.47 Similarly, in another work, they used M1 macrophage membrane-coated NPs to create a nano-drug system with laser-responsive, size-variable, on-demand drug release and prolonged circulatory retention, achieving safe and effective anti-tumor immunity.48 Overall, M1 cell membrane-coated NPs have excellent stability and tumor targeting ability, and will improve the tumor treatment effect.
Dendritic cells (DC) are specialized antigen-presenting cells in the immune system and have the potential to stimulate or suppress immune responses. The immunomodulatory ability of DC has broad prospects in the treatment of cancer, autoimmune diseases, and the prevention of transplant rejection. However, early clinical preclinical and clinical data indicate that DC vaccination only induces effective anti-tumor immunity in a small number of patients. Therefore, there is an urgent need for alternative strategies to improve the performance of DC-based immunotherapy. In an example, Wang and coworkers wrapped DC membranes in PLGA loaded with interleukin-2 and called them “mini DC” (Fig. 4C).49 Mini DCs can inherit key membrane proteins (including MHC and costimulatory molecules) from its source cells, which can interact with T cell receptors (TCRs) and CD28 on T cells for antigen presentation and the activation of T cells. In a mouse model of ovarian cancer, the researchers demonstrated that mini DCs have excellent therapeutic and preventive effects towards cancer, including delayed tumor growth and reduced tumor metastasis.
T cell immunotherapy is an emerging treatment method that can achieve good therapeutic effects for tumor treatment. Specific immune recognition proteins on T cells, such as TCRs, can recognize related molecules on the surface of tumors, thereby exhibiting a natural high affinity for tumors. The immune recognition properties of T cells make their membranes an effective vehicle for tumor targeting. Due to tumor heterogeneity, the single-target strategy is insufficient, and a dual-targeting strategy can increase the accumulation of NPs in malignant lesions and afford better anti-tumor effects. Based on this, the Cai group used an N3-labeled T cell membrane to coat a nano-photosensitizer (indocyanine green, ICG) to increase its effective accumulation in tumors and achieve a highly efficient PTT effect (Fig. 4D).50
Natural killer cells (NK cells) are an extremely important type of immune cell in the human body, which play a key role in the immune defense against cancer and pathogens because they can induce apoptosis by releasing cytotoxic molecules or connecting through active receptors. NK cells can target and kill tumor cells with the help of RANKL and DNAM1 proteins on the membrane without being exposed to tumor antigens. In addition, membrane proteins such as IRGM1, CB1, and Galectin12 can reprogram macrophages to fight tumors. Based on this fact, the Cai group designed NK cell membrane-coated NPs for effective immunotherapy (Fig. 4E). In their immune strategy, NK cell membranes are used to coat the photosensitizer TCPP to form NK-NPs.18b Firstly, NK cell membranes endow NK-NPs with the ability to target tumors and can reprogram M2-type macrophages into M1 type macrophages, thereby generating anti-tumor immunity. Secondly, TCPP loaded in NK-NPs can induce cancer cell death through photodynamic therapy, thereby realizing the combination of PDT and immunotherapy, achieving the elimination of primary tumors and the suppression of distant tumors.
Cancer cell clothes
Compared with other cells, cancer cells have various unique characteristics, such as unlimited replication potential, immune escape, and homologous targeting ability, and do not need to be obtained from the patient's or donor's plasma. Therefore, it is easy to culture them in vitro and obtain their membrane material. These properties have caused them to attract widespread attention in the treatment of different types of cancer. Although NPs camouflaged by cancer cell membranes (CCMs) have great potential in solving the problems of the insufficient transport of anti-tumor drugs in cells and the accumulation of subcellular drugs, their efficacy is still limited due to ineffective tumor penetration and intracellular transport. In view of this, Gan's group developed a yolk–shell structure NP for chemotherapy and tumor homologous targeting.51 The study highlights that NPs that can promote tumor penetration and powerful intracellular transport have great prospects in cancer chemotherapy. The NP with a yolk–shell structure is made of PEGylated liposomes supported by mesoporous silica NPs (MSNs) as the yolk and wrapped with CCM (CCM@LM) (Fig. 5A). The yolk–shell structure gives CCM@LM a moderate rigidity, which may help it frequently transform into an oval shape during the infiltration process, thereby promoting the infiltration of multicellular spheroids in vitro. CCM@LM also exhibits cell invasion characteristics that mimic the invasion characteristics of enveloped viruses. CCM@LM is directly internalized through membrane fusion, and the PEGylated egg yolk (LM) is then released into the cytoplasm, indicating that the execution of the internalization pathway is similar to that of enveloped viruses. The incoming PEGylated LM is further efficiently transported throughout the cytoskeleton filament network, resulting in enhanced perinuclear aggregation. Finally, CCM@LM was co-encapsulated with a small dose of doxorubicin and the poly(ADP-ribose) polymerase inhibitor mefuparib hydrochloride, which has a significantly stronger anti-tumor effect than the first-line chemotherapy drug Doxil. Cell membranes are not only beneficial in coating nano-NPs to deliver drugs but also have many advantages in the delivery of viral vaccines. Cerullo et al. created an artificially encapsulated viral nanovaccine ExtraCRAd using cancer cell membranes (Fig. 5B).19c ExtraCRAd contains two important parts: one is the oncolytic virus, and the other is the tumor cell membrane carrying tumor antigens. ExtraCRAd slows tumor growth in both preventive and therapeutic settings and activates cancer-specific immune responses in therapeutic and vaccination settings in different murine tumor models.
 |
| Fig. 5 Cancer cell membrane biomimetic system for improving tumor therapy or inhibiting tumor growth. (A) Cancer membrane-coated NPs form yolk–shell structures to enhance cancer chemotherapy. Adapted with permission.51 Copyright 2020. American Chemical Society. (B) Oncolytic viruses are encapsulated in cancer cell membranes for tumor growth inhibition and anti-tumor immune activation. Adapted with permission.19c Copyright 2019. Springer Nature. | |
Stem cell clothes
Stem cells are known for self-renewal, multidirectional differentiation, and low immunogenicity and have been widely used for various therapeutic purposes. Mesenchymal stem cells (MSCs) are adult stem cells that can be isolated from many tissues, such as adipose tissue, peripheral blood, umbilical cord, and placenta. These cells show unique biological characteristics and extraordinary growth ability in vitro, enabling them to grow rapidly to reach the amount required for in vivo treatment. In addition, MSCs have many advantages, such as prolonged circulation in the blood, immune escape, and tumor-promoting properties. Therefore, they are an ideal choice for NP invisible cloaks. He et al. prepared a stem cell membrane-coated gelatin nanogel material (SCMG) by a top-down fabrication method, which contains freshly harvested mesenchymal stem cell vesicles and a nanogel containing the anticancer drug doxorubicin hydrochloride (DOX)52 (Fig. 6A). The produced SCMGs share the biological functions of natural stem cell membranes, which promotes the remarkable stability and tumor-targeting ability of patients in vitro and in vivo. In addition, in vivo experiments have shown that when injected intravenously, the SCMGs were better targeted and accumulated in tumor tissues compared with gelatin–DOX and free-DOX, and the anti-tumor treatment efficiency of SCMG–DOX is significant. Stem cells are different from other cells. If only the function of their membrane is used, some of their stemness may be lost. However, simple stem cell therapy has safety risks. The enucleated human mesenchymal stromal cells (Cargocytes)53 created by Klemke et al. solve the above two problems very well. Cargocytes are a novel biomimetic system that uses density gradient centrifugation to enucleate but retain intrinsic cellular functions. Since it is enucleated, it will not reproduce or be permanently transplanted into the host. In turn, other organelles are preserved, enabling them to produce energy and proteins, adhere to inflammatory endothelial cells via integrins, and actively home to specific chemokine gradients released by diseased tissues. Compared to cell therapy and other biomimetic systems, Cargocytes have the potential to treat a range of diseases in a controlled and effective manner with a higher margin of safety (Fig. 6B).
 |
| Fig. 6 A stem cell membrane biomimetic system and the role of enucleated stem cells in disease treatment. (A) In vivo tumor-targeting drug delivery using stem cell membrane-coated gelatin nanogels (SCMGs). Adapted with permission.52 Copyright 2016. Wiley-VCH. (B) In acute inflammation models, enucleated human mesenchymal stromal cells enhance adhesion to inflamed endothelial cells and actively home to inflammatory diseased tissue, ultimately reducing inflammation and improving disease pathology. Adapted with permission.53 Copyright 2021. Springer Nature. | |
Hybrid cell clothes
The functions that a single type of cell membrane coating can confer on NPs are limited. Hybrid cell membrane-camouflaged NPs can successfully integrate different functions into a single NP, thus providing many advantages for various treatments. For example, fusion of the erythrocyte membrane and cancer cell membrane can afford a hybrid membrane with long blood circulation and homologous targeting performance. Yang's group fused the RBC membrane with the breast cancer cell (MCF-7) membrane to prepare a RBC–cancer cell hybrid membrane (Fig. 7A). Furthermore, melanin NPs (Melanin@RBC-M) were wrapped in a hybrid film to enhance the curative effect of tumor photothermal therapy (PTT).54
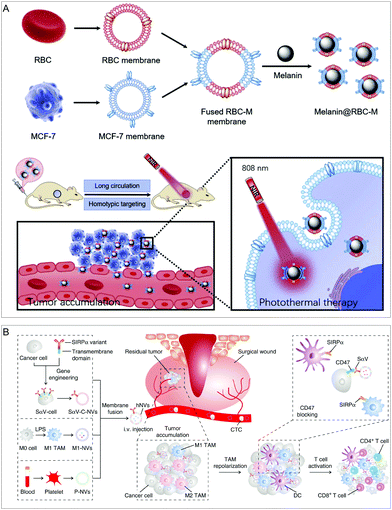 |
| Fig. 7 Hybrid cell membrane-coated NPs. (A) Long-circulation and homotypic targeting erythrocyte–cancer hybrid membrane-coated NPs (Melanin@RBC-M) can improve tumor photothermal therapy. Adapted with permission.54 Copyright 2019. Elsevier Ltd. (B) An engineered cancer cell–M1 macrophage–platelet fusion membrane (hNV) has tumor accumulation and immunomodulatory capabilities. Adapted with permission.21b Copyright 2020. Springer Nature. | |
Fusion of more cell membranes can obtain synergistically enhanced functions. Chen's group fused three kinds of membranes into a new type of hybrid membrane nanovesicle (hNV) with multiple functions (Fig. 7B).21b The hNVs can amplify the immune response of macrophages to cancer recurrence and metastasis. Specifically, genetic engineering was used to obtain membrane vesicles of cancer cells with SIRPα variants (SαV-C-NVs) that have significantly increased affinity for CD47. Then, they fused SαV-C-NVs with an M1-type macrophage membrane (M1-NVs) and platelet membrane (P-NVs) to form the hNVs. Platelet membranes embedded in the hNVs help to recognize and interact with CTCs in the blood and accumulate at the surgical site. M1-NVs and SαV-C-NVs embedded in the hNVs can repolarize tumor-associated macrophages (TAM) to the M1 phenotype and block the CD47-SIRPα interaction pathway, thereby causing macrophages to phagocytose cancer cells and enhancing anti-tumor T cell immunity. In a mouse model of malignant melanoma, by controlling local recurrence and distant metastasis after surgery, the hNVs significantly prolonged overall survival.
Exosome membrane clothes
Another important membrane source is from the naturally occurring nanoscale extracellular vesicles called exosomes that are derived from all living systems and play an important role in intercellular communication.55 Exosomes themselves have the function of intercellular transmission, and their structure is a vesicle composed of lipid bilayers, so they have the ability to carry cargo or therapeutic drugs to tumor cells. Due to the small immunogenicity, small size, easy modification, and tumor antigen-rich nature caused by their own endogenous properties, exosome membrane (EM)-coated NPs also have a variety of immunomodulatory effects and have great potential in cancer treatment.56
Due to the tumor-targeting properties of EMs, the combination of EM-coated NPs with other therapies is expected to provide a new approach for tumor therapy. Jin and colleagues developed tumor cell-derived exosome-camouflaged porous silicon NPs (E-MSNs) as a drug delivery system co-loaded with ICG and DOX (ID@E-MSNs) (Fig. 8A).57 ID@E-MSNs could be efficiently taken up by tumor cells and enhance tumor accumulation via EM. ID@E-MSNs also retained the photothermal effect of ICG and the cytotoxicity of DOX. ID@E-MSNs can accumulate tumor tissue, and under 808 nm near-infrared irradiation, ICG can generate hyperthermia to collapse E-MSN nanocarriers, accelerate drug release, and induce tumor ablation, thereby inhibiting tumor growth and metastasis.
 |
| Fig. 8 Therapeutic applications of exosome-coated nanoparticles. (A) Exosome membrane-coated NPs for combined photothermal therapy and chemotherapy. Adapted with permission.57 Copyright 2020. Frontiers. (B) DNA aptamer-modified exosomal membrane-encapsulated NPs enhance tumor targeting. Adapted with permission.25 Copyright 2020. American Chemical Society. | |
Functionalization of the EM can further improve the performance of NPs. Based on this, Sun et al. designed a strategy for the modification of EM-coated poly(lactic-co-glycolic acid) (PLGA) NPs using microfluidic sonication and cholesterol-modified AS1411 aptamers. The resulting EM-coated NPs exhibited prolonged in vivo circulation time, good biocompatibility, and high tumor-targeting efficiency (Fig. 8B).25 This work demonstrates that fabricated and functionalized EM-coated NPs have the potential for targeted drug delivery.
4. Prospects and challenges
The biomimetic system created by adopting the cell membrane-coating strategy improves some properties of the NPs themselves and endows them with relevant biofilm properties, such as long circulation, biocompatibility, immune escape, tumor targeting, and immunomodulatory functions. These properties contribute to the accumulation of membrane-coated NPs at tumor sites, effectively suppressing tumor growth and improving therapeutic efficacy. Nonetheless, the development of biomimetic systems is still in its infancy, and there are numerous obstacles that must be fully considered and overcome before further progress can be made.58 Several of these include the choice of cell membrane sources, the construction of biomimetic systems, the impact of membrane integrity in biomimetic systems, and the selection of new biomimetic materials.
Firstly, because different cell membranes have different functions, the most important thing for different treatment modalities is the choice of the cell source.59 Since the combination of different cell membranes and different NPs can construct various therapeutic systems, the selection of cell membranes should be made according to the purpose of the therapeutic system.60 For example, if you want to achieve long circulation, you can choose the RBC membrane first; if you want to prepare a circulating tumor targeting system, you can choose the platelet membrane; if you want to target other solid tumors, you can choose the cell membrane derived from homologous tumor cells; tumor-targeting can also be combined with immune activation or immune killing. To achieve this goal, immune cell-derived membranes can be selected or hybrid cell membranes can be used to achieve more functions. In conclusion, the choice of the cell membrane needs to be based on its role in the therapeutic system. However, the difficulty of obtaining various cell membranes is inequable. Currently, only erythrocyte membranes are relatively easy to obtain, but other sources of cell membranes require a lengthy acquisition process and show low yields, which could be a bottleneck in the development of biomimetics. Standardization, yield, sterilization, and risk assessment in the use of various cell membrane extractions are challenges in the field.
Secondly, ensuring the integrity and functionality of the cell membrane coating on the NPs, a key metric related to the quality of these biomimetic systems and the resulting biomedical functions, largely affects the properties of the NPs themselves and their internalization mechanisms.61 Most coating methods assume that the cell membrane uniformly covers the entire surface of the NPs to form a core–shell structure. However, this top-down biomimetic procedure requires first disrupting the cell integrity to obtain cell membranes, and then fusing them with the core NPs by applying external forces (such as extrusion or sonication). Whether the protein composition and function of cell membranes are preserved can be characterized by SDS-PAGE and WB and verified in vitro and in vivo. If we hypothesize that these synthetic methods can result in a lack of the full integrity of the re-assembled cell membrane coatings, then these imperfect biomimetic systems may affect their biomedical functions, through factors such as cargo leakage in drug delivery systems, undesired biomolecule adsorption occurring in physiological fluids, and last and least expected, immune clearance. As a result, it is essential to investigate whether biomimetic NPs can replicate cell membrane integrity. Fortunately, researchers have begun to explore the impact of membrane integrity on biomimetic systems.
More importantly, even if the membrane integrity problem is solved in the cell membrane biomimetic system, traditional cell membrane-coated NPs have lost their original organelles and key cellular mechanisms, resulting in the inability to “decipher” key signals and chemical gradients required for chemical orientation, the disability to generate energy and activate high-affinity integrin-mediated endothelial adhesion, and being less able to generate propulsive forces to overflow the vasculature.53 As a result, under the condition of ensuring application safety and controllability, a biomimetic system with cell communication functions that can regulate physiological and pathological processes and have certain chemotactic abilities to diseased tissues may be the biomimetic delivery vehicle of choice in the future. A good example is extracellular vesicles (EVs), which have developed rapidly over the past decade and play an important role in cellular communication,62 in addition to their bilayer membrane properties for drug loading and NP coating.63 Today, researchers have begun to shift from basic biological research on EVs to their clinical diagnostic and therapeutic applications. One of the most important applications of research is to use them as a targeted delivery vehicle for therapeutic molecules.64 Despite the technical challenges in EV separation and inefficiencies in therapeutic cargo loading, once these challenges are overcome, EVs will unlock their full potential in drug delivery applications. In addition, the Cargocyte delivery vehicle developed by Klemke et al.53 may also be a biomimetic carrier in the future.
With the development of membrane-separation and membrane-coating technologies, biomimetic NPs with insufficient membrane integrity and functional defects will be reduced. Although amazing progress has been made in membrane-functionalized NPs, the key bottleneck to realizing their clinical significance is still the standardization of nanotechnology, membrane-separation technology, and membrane-coating technology. So, before that, there are still many difficulties to overcome.
5. Conclusions
Cell membrane-coated NPs possess highly complex biological functions while preserving the inherent physicochemical properties of the NP core. This efficient strategy can improve the delivery of NPs to specific sites by translocating the entire cell membrane from a specific biologically derived cell onto the surface of a synthetic NP. In this article, we focus on four techniques for membrane-coated NPs as well as the application of various sources of derived cell membrane-engineered NPs in various therapies. We also present the prospects and challenges in the synthesis and application of cell membrane-engineered NPs. With the development of nanotechnology, an increasing number of functional NP cores can be developed. All in all, the combination of cell membranes and NPs can construct different therapeutic systems, which is expected to improve their therapeutic effect towards cancer.
Conflicts of interest
There are no conflicts to declare.
Acknowledgements
This work was supported by the National Natural Science Foundation of China (32101148, 31871003, 32171394), the Beijing-Tianjin-Hebei Basic Research Cooperation Project (19JCZDJC64100), the Beijing Nova Program from Beijing Municipal Science & Technology Commission (Z201100006820005), the National Key R&D Program of China (2021YFE0106900, 2021YFA1201000), and the Natural Science Foundation of Guangdong Province (2019A1515010776).
References
- H. Sung, J. Ferlay, R. L. Siegel, M. Laversanne, I. Soerjomataram, A. Jemal and F. Bray, Ca-Cancer J. Clin., 2021, 71, 209–249 CrossRef PubMed.
-
(a) A. Narmani and S. M. Jafari, Carbohydr. Polym., 2021, 272, 118464 CrossRef CAS PubMed;
(b) Z. Cheng, M. Li, R. Dey and Y. Chen, J. Hematol. Oncol., 2021, 14, 1–27 CrossRef PubMed;
(c) X. Kong, R. Cheng, J. Wang, Y. Fang and K. C. Hwang, Nano Today, 2021, 36, 101004 CrossRef CAS.
-
(a) M. S. Goldberg, Nat. Rev. Cancer, 2019, 19, 587–602 CrossRef CAS PubMed;
(b) M. Ferrari, Nat. Rev. Cancer, 2005, 5, 161–171 CrossRef CAS PubMed;
(c) R. S. Riley, C. H. June, R. Langer and M. J. Mitchell, Nat. Rev. Drug Discovery, 2019, 18, 175–196 CrossRef CAS PubMed;
(d) R. M. Williams, S. Chen, R. E. Langenbacher, T. V. Galassi, J. D. Harvey, P. V. Jena, J. Budhathoki-Uprety, M. Luo and D. A. Heller, Nat. Chem. Biol., 2021, 17, 129–137 CrossRef CAS PubMed;
(e) V. R. Devadasu, V. Bhardwaj and M. R. Kumar, Chem. Rev., 2013, 113, 1686–1735 CrossRef CAS PubMed;
(f) Y. Zhang, H. Yang, D. Wei, X. Zhang, J. Wang, X. Wu and J. Chang, Exploration, 2021, 1, 20210115 CrossRef.
-
(a) Y. Matsumura and H. Maeda, Cancer Res., 1986, 46, 6387–6392 CAS;
(b) S. K. Golombek, J.-N. May, B. Theek, L. Appold, N. Drude, F. Kiessling and T. Lammers, Adv. Drug Delivery Rev., 2018, 130, 17–38 CrossRef CAS PubMed.
-
(a) M. Mahmoudpour, S. Ding, Z. Lyu, G. Ebrahimi, D. Du, J. E. N. Dolatabadi, M. Torbati and Y. Lin, Nano Today, 2021, 39, 101177 CrossRef CAS;
(b) R. Mout, D. F. Moyano, S. Rana and V. M. Rotello, Chem. Soc. Rev., 2012, 41, 2539–2544 RSC;
(c) V. Biju, Chem. Soc. Rev., 2014, 43, 744–764 RSC;
(d) M. J. Mitchell, M. M. Billingsley, R. M. Haley, M. E. Wechsler, N. A. Peppas and R. Langer, Nat. Rev. Drug Discovery, 2021, 20, 101–124 CrossRef CAS PubMed;
(e) T. Sun, Y. S. Zhang, B. Pang, D. C. Hyun, M. Yang and Y. Xia, Angew. Chem., Int. Ed., 2014, 53, 12320–12364 CAS.
-
(a) E. Blanco, H. Shen and M. Ferrari, Nat. Biotechnol., 2015, 33, 941–951 CrossRef CAS PubMed;
(b) L. Kou, Y. D. Bhutia, Q. Yao, Z. He, J. Sun and V. Ganapathy, Front. Pharmacol., 2018, 9, 27 CrossRef PubMed.
-
(a) P. T. Wong and S. K. Choi, Chem. Rev., 2015, 115, 3388–3432 CrossRef CAS PubMed;
(b) L. Tu, Z. Liao, Z. Luo, Y. L. Wu, A. Herrmann and S. Huo, Exploration, 2021, 1, 20210329 CrossRef.
-
(a) X. Fu, Y. Shi, T. Qi, S. Qiu, Y. Huang, X. Zhao, Q. Sun and G. Lin, Signal Transduct. Target. Ther., 2020, 5, 1–15 CrossRef;
(b) P. Navya, A. Kaphle, S. Srinivas, S. K. Bhargava, V. M. Rotello and H. K. Daima, Nano Convergence, 2019, 6, 1–30 CrossRef CAS PubMed;
(c) B. B. Mendes, D. P. Sousa, J. Conniot and J. Conde, Trends Cancer, 2021, 7, 847–862 CrossRef CAS PubMed;
(d) J. Shi, P. W. Kantoff, R. Wooster and O. C. Farokhzad, Nat. Rev. Cancer, 2017, 17, 20–37 CrossRef CAS PubMed;
(e) M. J. Mitchell, M. M. Billingsley, R. M. Haley, M. E. Wechsler and R. Langer, Nat. Rev. Drug Discovery, 2020, 20, 101–124 CrossRef PubMed.
-
(a) C. von Roemeling, W. Jiang, C. K. Chan, I. L. Weissman and B. Y. Kim, Trends Biotechnol., 2017, 35, 159–171 CrossRef CAS PubMed;
(b) T. Cedervall, I. Lynch, S. Lindman, T. Berggård, E. Thulin, H. Nilsson, K. A. Dawson and S. Linse, Proc. Natl. Acad. Sci. U. S. A., 2007, 104, 2050–2055 CrossRef CAS PubMed;
(c) S. Tenzer, D. Docter, J. Kuharev, A. Musyanovych, V. Fetz, R. Hecht, F. Schlenk, D. Fischer, K. Kiouptsi and C. Reinhardt, Nat. Nanotechnol., 2013, 8, 772–781 CrossRef CAS PubMed.
- Y. Tang, X. Wang, J. Li, Y. Nie, G. Liao, Y. Yu and C. Li, ACS Nano, 2019, 13, 13015–13026 CrossRef CAS PubMed.
- A. S. Karakoti, R. Shukla, R. Shanker and S. Singh, Adv. Colloid Interface, 2015, 215, 28–45 CrossRef CAS PubMed.
- Y. Zhao, Z. Zhang, Z. Pan and Y. Liu, Exploration, 2021, 1, 20210089 CrossRef.
- N. Hadjesfandiari and A. Parambath, Eng. Biomater. Drug Delivery Sys., 2018, 345–361 CAS.
- M. R. Qelliny, A. K. Hussein and K. A. Khaled, J. Adv. Biomed. Pharmaceut. Sci., 2022, 5, 45–53 Search PubMed.
-
(a) L. Chen, W. Hong, W. Ren, T. Xu, Z. Qian and Z. He, Signal Transduct. Target. Ther., 2021, 6, 1–25 CrossRef PubMed;
(b) V. Chugh, K. Vijaya Krishna and A. Pandit, ACS Nano, 2021, 15, 17080–17123 CrossRef CAS PubMed.
-
(a) C.-M. J. Hu, L. Zhang, S. Aryal, C. Cheung, R. H. Fang and L. Zhang, Proc. Natl. Acad. Sci. U. S. A., 2011, 108, 10980–10985 CrossRef CAS PubMed;
(b) E. Ben-Akiva, R. A. Meyer, H. Yu, J. T. Smith, D. M. Pardoll and J. J. Green, Sci. Adv., 2020, 6, eaay9035 CrossRef CAS PubMed;
(c) M. Xuan, J. Shao, J. Zhao, Q. Li, L. Dai and J. Li, Angew. Chem., Int. Ed., 2018, 57, 6049–6053 CrossRef CAS PubMed;
(d) X. Han, S. Shen, Q. Fan, G. Chen, E. Archibong, G. Dotti, Z. Liu, Z. Gu and C. Wang, Sci. Adv., 2019, 5, eaaw6870 CrossRef CAS PubMed;
(e) W. Yu, X. He, Z. Yang, X. Yang, W. Xiao, R. Liu, R. Xie, L. Qin and H. Gao, Biomaterials, 2019, 217, 119309 CrossRef CAS PubMed.
-
(a) M. Li, J. Li, J. Chen, Y. Liu, X. Cheng, F. Yang and N. Gu, ACS Nano, 2020, 14, 2024–2035 CrossRef CAS PubMed;
(b) J. Zhuang, H. Gong, J. Zhou, Q. Zhang, W. Gao, R. H. Fang and L. Zhang, Sci. Adv., 2020, 6, eaaz6108 CrossRef CAS PubMed.
-
(a) L. Feng, C. Dou, Y. Xia, B. Li, M. Zhao, P. Yu, Y. Zheng, A. M. El-Toni, N. F. Atta and A. Galal, ACS Nano, 2021, 15, 2263–2280 CrossRef CAS PubMed;
(b) G. Deng, Z. Sun, S. Li, X. Peng, W. Li, L. Zhou, Y. Ma, P. Gong and L. Cai, ACS Nano, 2018, 12, 12096–12108 CrossRef CAS PubMed;
(c) C. Gao, Q. Huang, C. Liu, C. H. Kwong, L. Yue, J.-B. Wan, S. M. Lee and R. Wang, Nat. Commun., 2020, 11, 1–14 CrossRef PubMed.
-
(a) R. Yang, J. Xu, L. Xu, X. Sun, Q. Chen, Y. Zhao, R. Peng and Z. Liu, ACS Nano, 2018, 12, 5121–5129 CrossRef CAS PubMed;
(b) L. Rao, G. T. Yu, Q. F. Meng, L. L. Bu, R. Tian, L. S. Lin, H. Deng, W. Yang, M. Zan and J. Ding, Adv. Funct. Mater., 2019, 29, 1905671 CrossRef CAS;
(c) M. Fusciello, F. Fontana, S. Tähtinen, C. Capasso, S. Feola, B. Martins, J. Chiaro, K. Peltonen, L. Ylösmäki and E. Ylösmäki, Nat. Commun., 2019, 10, 1–13 CrossRef PubMed;
(d) M. Z. Alyami, S. K. Alsaiari, Y. Li, S. S. Qutub, F. A. Aleisa, R. Sougrat, J. S. Merzaban and N. M. Khashab, J. Am. Chem. Soc., 2020, 142, 1715–1720 CrossRef CAS PubMed.
-
(a) M. Wang, Y. Xin, H. Cao, W. Li, Y. Hua, T. J. Webster, C. Zhang, W. Tang and Z. Liu, Biomater. Sci., 2021, 9, 1088–1103 RSC;
(b) J. Tang, D. Shen, T. G. Caranasos, Z. Wang, A. C. Vandergriff, T. A. Allen, M. T. Hensley, P.-U. Dinh, J. Cores and T.-S. Li, Nat. Commun., 2017, 8, 1–9 CrossRef PubMed.
-
(a) Y. Zhao, A. Li, L. Jiang, Y. Gu and J. Liu, Biomacromolecules, 2021, 22, 3149–3167 CrossRef CAS PubMed;
(b) L. Rao, L. Wu, Z. Liu, R. Tian, G. Yu, Z. Zhou, K. Yang, H.-G. Xiong, A. Zhang and G.-T. Yu, Nat. Commun., 2020, 11, 1–13 CrossRef PubMed.
- Y. Zhai, J. Su, W. Ran, P. Zhang, Q. Yin, Z. Zhang, H. Yu and Y. Li, Theranostics, 2017, 7, 2575 CrossRef CAS PubMed.
- C.-M. J. Hu, R. H. Fang, K.-C. Wang, B. T. Luk, S. Thamphiwatana, D. Dehaini, P. Nguyen, P. Angsantikul, C. H. Wen and A. V. Kroll, Nature, 2015, 526, 118–121 CrossRef CAS PubMed.
- L. Rao, B. Cai, L.-L. Bu, Q.-Q. Liao, S.-S. Guo, X.-Z. Zhao, W.-F. Dong and W. Liu, ACS Nano, 2017, 11, 3496–3505 CrossRef CAS PubMed.
- Z. Han, W. Lv, Y. Li, J. Chang, W. Zhang, C. Liu and J. Sun, ACS Appl. Bio. Mater., 2020, 3, 2666–2673 CrossRef CAS PubMed.
- X. Zhou, B. Luo, K. Kang, Y. Zhang, P. Jiang, F. Lan, Q. Yi and Y. Wu, Small, 2019, 15, 1900558 CrossRef PubMed.
- A. K. A. Silva, R. Di Corato, T. Pellegrino, S. Chat, G. Pugliese, N. Luciani, F. Gazeau and C. Wilhelm, Nanoscale, 2013, 5, 11374–11384 RSC.
- B. S. Ahluwalia, P. McCourt, A. Oteiza, J. S. Wilkinson, T. R. Huser and O. G. Hellesø, Analyst, 2015, 140, 223–229 RSC.
- H. Chen, H. Sha, L. Zhang, H. Qian, F. Chen, N. Ding, L. Ji, A. Zhu, Q. Xu and F. Meng, Int. J. Nanomed., 2018, 13, 5347 CrossRef CAS PubMed.
- X. Han, C. Wang and Z. Liu, Bioconjugate Chem., 2018, 29, 852–860 CrossRef CAS PubMed.
- P.-A. Oldenborg, A. Zheleznyak, Y.-F. Fang, C. F. Lagenaur, H. D. Gresham and F. P. Lindberg, Science, 2000, 288, 2051–2054 CrossRef CAS PubMed.
- L. Rao, L. L. Bu, J. H. Xu, B. Cai, G. T. Yu, X. Yu, Z. He, Q. Huang, A. Li and S. S. Guo, Small, 2015, 11, 6225–6236 CrossRef CAS PubMed.
- W. Tang, W. Fan, J. Lau, L. Deng, Z. Shen and X. Chen, Chem. Soc. Rev., 2019, 48, 2967–3014 RSC.
- Y. Zhao, J. Wang, X. Cai, P. Ding, H. Lv and R. Pei, ACS Appl. Mater. Interfaces, 2020, 12, 23697–23706 CrossRef CAS PubMed.
- Y. Lu, Q. Hu, C. Jiang and Z. Gu, Cur. Opin. Biotechnol., 2019, 58, 81–91 CrossRef CAS PubMed.
- H. Han, R. Bártolo, J. Li, M.-A. Shahbazi and H. A. Santos, Eur. J. Pharm. Biopharm., 2022, 172, 1–15 CrossRef CAS PubMed.
- P. Kanikarla-Marie, M. Lam, D. G. Menter and S. Kopetz, Cancer Metast. Rev., 2017, 36, 235–248 CrossRef CAS PubMed.
- B. Nieswandt and S. P. Watson, Blood, 2003, 102, 449–461 CrossRef CAS PubMed.
- J. R. Fitzgerald, T. J. Foster and D. Cox, Nat. Rev. Microbiol., 2006, 4, 445–457 CrossRef CAS PubMed.
- M. Olsson, P. Bruhns, W. A. Frazier, J. V. Ravetch and P.-A. Oldenborg, Blood, 2005, 105, 3577–3582 CrossRef CAS PubMed.
- H. Ye, K. Wang, M. Wang, R. Liu, H. Song, N. Li, Q. Lu, W. Zhang, Y. Du and W. Yang, Biomaterials, 2019, 206, 1–12 CrossRef CAS PubMed.
- B. Bahmani, H. Gong, B. T. Luk, K. J. Haushalter, E. DeTeresa, M. Previti, J. Zhou, W. Gao, J. D. Bui and L. Zhang, Nat. Commun., 2021, 12, 1–12 CrossRef PubMed.
- Q. Hu, W. Sun, C. Qian, C. Wang, H. N. Bomba and Z. Gu, Adv. Mater., 2015, 27, 7043–7050 CrossRef CAS PubMed.
- T. F. Gajewski, H. Schreiber and Y.-X. Fu, Nat. Immunol., 2013, 14, 1014–1022 CrossRef CAS PubMed.
- F. Oroojalian, M. Beygi, B. Baradaran, A. Mokhtarzadeh and M. A. Shahbazi, Small, 2021, 17, 2006484 CrossRef CAS PubMed.
- T. Kang, Q. Zhu, D. Wei, J. Feng, J. Yao, T. Jiang, Q. Song, X. Wei, H. Chen and X. Gao, ACS Nano, 2017, 11, 1397–1411 CrossRef CAS PubMed.
- R. Liu, Y. An, W. Jia, Y. Wang, Y. Wu, Y. Zhen, J. Cao and H. Gao, J. Controlled Release, 2020, 321, 589–601 CrossRef CAS PubMed.
- C. Hu, T. Lei, Y. Wang, J. Cao, X. Yang, L. Qin, R. Liu, Y. Zhou, F. Tong and C. S. Umeshappa, Biomaterials, 2020, 255, 120159 CrossRef CAS PubMed.
- S. Cheng, C. Xu, Y. Jin, Y. Li, C. Zhong, J. Ma, J. Yang, N. Zhang, Y. Li and C. Wang, Adv. Sci., 2020, 7, 1903301 CrossRef CAS PubMed.
- Y. Han, H. Pan, W. Li, Z. Chen, A. Ma, T. Yin, R. Liang, F. Chen, Y. Ma and Y. Jin, Adv. Sci., 2019, 6, 1900251 CrossRef PubMed.
- D. Nie, Z. Dai, J. Li, Y. Yang, Z. Xi, J. Wang, W. Zhang, K. Qian, S. Guo and C. Zhu, Nano Lett., 2019, 20, 936–946 CrossRef PubMed.
- C. Gao, Z. Lin, B. Jurado-Sánchez, X. Lin, Z. Wu and Q. He, Small, 2016, 12, 4056–4062 CrossRef CAS PubMed.
- H. Wang, C. N. Alarcón, B. Liu, F. Watson, S. Searles, C. K. Lee, J. Keys, W. Pi, D. Allen and J. Lammerding, Nat. Biomed. Eng., 2021, 1–16 Search PubMed.
- Q. Jiang, Y. Liu, R. Guo, X. Yao, S. Sung, Z. Pang and W. Yang, Biomaterials, 2019, 192, 292–308 CrossRef CAS PubMed.
- Z. G. Zhang, B. Buller and M. Chopp, Nat. Rev. Neurol., 2019, 15, 193–203 CrossRef PubMed.
- A. Mukherjee, B. Bisht, S. Dutta and M. K. Paul, Acta Pharmacol. Sin., 2022, 1–18 Search PubMed.
- R. Tian, Z. Wang, R. Niu, H. Wang, W. Guan and J. Chang, Front. Bioeng. Biotechnol., 2020, 1010 CrossRef PubMed.
- X. Liu, X. Zhong and C. Li, Chinese Chem. Lett., 2021, 32, 2347–2358 CrossRef CAS.
- W. Liu, Q. Yan, C. Xia, X. Wang, A. Kumar, Y. Wang, Y. Liu, Y. Pan and J. Liu, J. Mater. Chem. B, 2021, 9, 4459–4474 RSC.
- W. Zhang, F. Wang, C. Hu, Y. Zhou, H. Gao and J. Hu, Acta Pharm. Sin. B, 2020, 10, 2037–2053 CrossRef CAS PubMed.
- L. Liu, X. Bai, M.-V. Martikainen, A. Kårlund, M. Roponen, W. Xu, G. Hu, E. Tasciotti and V.-P. Lehto, Nat. Commun., 2021, 12, 1–12 CrossRef CAS.
- G. van Niel, D. R. Carter, A. Clayton, D. W. Lambert, G. Raposo and P. Vader, Nat. Rev. Mol. Cell Biol., 2022, 1–14 Search PubMed.
- R. C. de Abreu, H. Fernandes, P. A. da Costa Martins, S. Sahoo, C. Emanueli and L. Ferreira, Nat. Rev. Cardiol., 2020, 17, 685–697 CrossRef PubMed.
-
(a) L. Cheng and A. F. Hill, Nat. Rev. Drug Discovery, 2022, 1–21 Search PubMed;
(b) I. K. Herrmann, M. J. A. Wood and G. Fuhrmann, Nat. Nanotechnol., 2021, 16, 748–759 CrossRef CAS PubMed.
|
This journal is © The Royal Society of Chemistry 2022 |
Click here to see how this site uses Cookies. View our privacy policy here.