DOI:
10.1039/D2MA00311B
(Review Article)
Mater. Adv., 2022,
3, 5248-5259
Chemical labeling and crosslinking of tobacco mosaic virus via multi-diazonium reagents: examples, applications, and prospects
Received
17th March 2022
, Accepted 13th May 2022
First published on 20th May 2022
Abstract
Tobacco mosaic virus (TMV) is a rod-shaped hollow plant viral nanoparticle (300 nm × 18 nm) and exhibits abundant amino acid residues on the surface of capsid proteins for facile chemical labeling. The use of TMV as a nano-template to produce materials with multiple functions has received particular attention in the past decade. In addition, TMV can be largely produced in gram-scale quantities and is also considered much safe for mammals. Hence, using TMV as building blocks to assemble biomaterials (e.g., hydrogels) has emerged as an attractive field for biomedical applications. This minireview details up-to-date research on the development of bench-stable diazonium reagents and their applications for TMV labeling and crosslinking. The strategy for the preparation of virus-based hydrogels is highlighted. We hope that this review will inspire the development of a large number of plant virus-based biomaterials for various applications in the near future.
1. Introduction
Hydrogels are three-dimensional (3D) networks of hydrophilic polymers joined together by covalent bonds or physical intermolecular attraction.1–5 The presence of hydrophilic moieties such as amide, carboxyl, amino, and hydroxyl groups scattered along the backbone of the 3D networks contributes to the high hydrophilicity of hydrogels.2 Many hydrogels have good biocompatibility,3–5 and therefore the biomedical applications of hydrogels have expanded to many fields, including drug delivery,3 biosensors,6 wound healing,7 tissue engineering,5,8 cell culture,9 antibacterial materials,10 and others.11 These hydrogel materials can be prepared from different building blocks (e.g., cellulose nanofibrils and peptides)12–14 and using different strategies (Fig. 1).15–18 For example, enzyme-triggered peptides can form hydrogels through intermolecular weak interactions and self-assembly (Fig. 1a).19,20 A sort of chain-growth polymerization helps make nanocomposite hydrogels (Fig. 1b).21 The crosslinking (non-covalent interactions & covalent bond formation) between bifunctional molecules and crosslinkers represents one of the most widely used hydrogel production techniques (Fig. 1c).4,17,22 In addition, new multiple crosslinking network hydrogels that can overcome the bottlenecks of mechanical performance of single network hydrogels have flourished in recent years.23 Moreover, we discovered that the direct chemical crosslinking of plant viruses can be used as a new strategy to prepare hydrogels (Fig. 1d).24 In the present minireview, we focus on providing a brief summary of the development of bench-stable diazonium reagents and their labeling of tobacco mosaic virus (TMV) for generation of biomaterials including hydrogels.
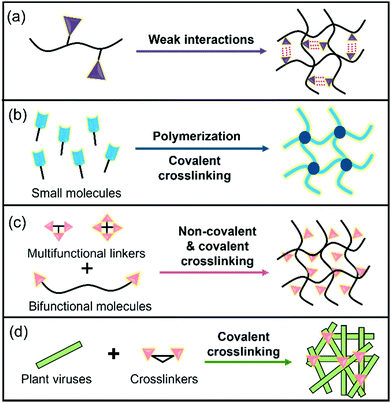 |
| Fig. 1 Construction of hydrogels via different strategies: (a) self-assembly of building blocks via weak interactions; (b) polymerization of small molecules via covalent crosslinking; (c) covalent or non-covalent crosslinking between multifunctional linkers and bifunctional molecules; (d) covalent crosslinking of plant viruses. | |
Plant viruses can be easily obtained in grams with high uniformity and are biocompatible with mammals, and therefore, they have numerous advantages for biological and materials science applications.25–28 Plant viruses can be divided into zero-dimensional (0D) icosahedral capsids and one-dimensional (1D) rod/filamentous-shaped capsids, both of which are virus nanoparticles (VNPs) that can be tens to hundreds of nanometers in size.25 Plant viruses are made up of many copies of one or more identical coat protein components that self-assemble into a capsid that encloses the virus genome. Therefore, both genetic engineering and bioconjugation technologies have allowed plant viruses to be amenable to manipulations (Fig. 2), providing virus-like nanoparticles (VLPs) for the advancement of an array of nanotechnology applications.29–33 In addition, the self-assemblies of viral capsids and viruses have potentially useful characteristics for a variety of nanotechnology applications including encapsulation.34–36 These plant VNPs and VLPs have also been employed as nanotemplates for biomineralization.37,38
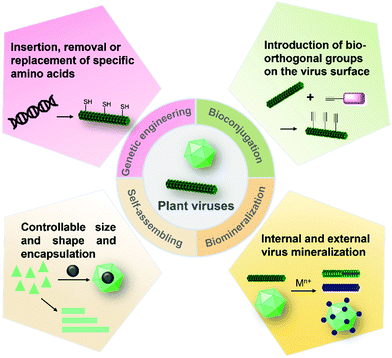 |
| Fig. 2 Modifications and construction of plant VNPs and VLPs. VNPs and VLPs can be generated by genetic engineering, bioconjugation, and self-assembly, all of which can be further functionalized by chemical labeling, biomineralization, or encapsulation.25 | |
TMV is a well-studied plant virus in the past century and was the first viral structure to be examined.39,40 TMV is composed of 2130 identical coat proteins with a molecular weight of 17
534 Da, which is arranged helically around a single-strand, positive-sense RNA molecule to generate a rod-shaped hollow nanoparticle with 300 nm in length and 18 nm in diameter (Fig. 3). Due to its special 1D structure, good biocompatibility, easily chemical and genetic modifications, TMV has aroused great research interest in self-assembly and as a building block for the construction of bio-nano composite materials.41 For example, wide-type TMV can be applied as a nanomaterial template for the construction of 1D nanowires and nanotubes.42,43 In addition, TMV particles contain tyrosine residues (Tyr139) on the external surface and glutamic acid residues (Glu97 and Glu106) at the internal surface (Fig. 3), providing two types of reactive handles for dual modifications on both surfaces.44,45 For example, a common strategy of Tyr modification is by using in situ diazonium generation and coupling,46,47 which may affect the structure of the protein as well as make it difficult to control the stoichiometry of the reaction. To solve this problem, several bench-stable diazonium salts have recently been developed for TMV labeling and crosslinking.24 On the other hand, Cys and Lys residues were genetically engineered onto the virion surface of TMV mutants, producing additional attachment sites for mineralization48,49 and bioconjugation.50–53 As a result, TMV and mutants are widely functionalized by small molecules, polymers, peptides, MRI contrast agents, antigens, and therapeutics for various applications.54–64 We note that there are a series of excellent reviews for TMV-based nanomaterials during the last decade.60–64 However, the current development of stable diazonium reagents for TMV labeling is not summarized. In addition, the 1D lengths and the diameters of the TMV nanoparticle can be tuned and controlled via self-assembly and chemical labeling to generate virus-like particles (VLPs),65–67 which can break the limitation of fixed sizes of natural virus templates. Moreover, dual-diazonium reagents for TMV crosslinking provide an additional strategy for the preparation of hydrogels.24 This recent new progress can inspire various new biomaterials for research in different directions but has not been specially reviewed in previous papers.
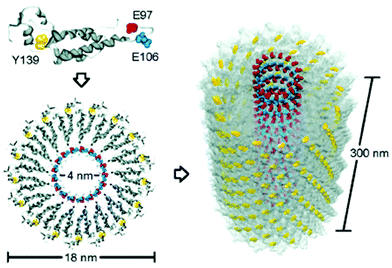 |
| Fig. 3 Schematic illustration of the TMV structures and the potential reactive positions of tyrosine 139 (yellow), glutamate 97 (red), and glutamate 106 (blue) in a capsid monomer.44 Reprinted with permission from ref. 44. Copyright (2005), American Chemical Society. | |
Here, we will provide a full description of the development of stable diazonium reagents from our group as well as others, which may help readers to clearly understand their properties and potential applications in chemical biology and materials science. This minireview also summarizes the recent research on chemical modifications of TMV Tyr139, the construction of controllable TMV-based VLPs, and virus-based hydrogels. The synthesis and methods for making these reagents and biomaterials are described in detail. Because bioorthogonal chemical reactions have emerged as excellent tools for biolabeling,68–75 we discussed the combination between bioorthogonal reactions and diazonium salt reagents. We also outline the future directions for crosslinking of plant viruses via multi-diazonium reagents. We anticipate that this minireview will promote the further development of plant virus-based biomaterials for a variety of applications.
2. Development of diazonium salts and reagents for TMV modifications
A traditional diazonium coupling reaction to tyrosine residues of TMV is very useful for the construction of new materials.44,45 For example, Francis and coworkers reported that efficient modifications of TMV capsids were accomplished through a mixture of intact viral capsids and 35 equiv. of diazonium salts (in situ synthesis at 0 °C) in pH 9 buffer for 2 h.44 The formation of 2 using 1 provides ketone sites for further convenient conjugation via the oxime ligation (Fig. 4a). For example, upon exposure of 2 to polyethyleneglycol (PEG)- or biotin-containing alkoxyamines, adducts 3 and 4 were obtained with virtually complete conversion, and >2000 copies of biotin were installed on the capsid exterior. In addition, the generated PEG-labeled capsids 3 was organically soluble TMV rods that may greatly expand the conditions for further bioconjugation reactions as well as future device fabrication in the organic phase.
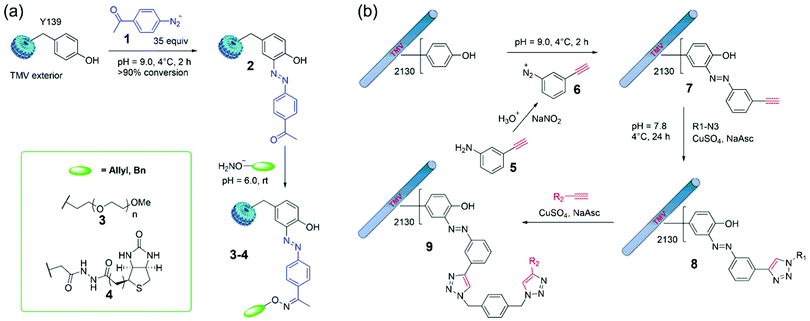 |
| Fig. 4 (a) Site-selective azo-coupling on Y139 in the TMV exterior with in situ generated diazonium salts 1 and further labeling via oxime ligation.44 (b) Formation of a diazonium salt 6in situ and the bioconjugation of TMV by means of CuAAC reactions.77 Reprinted with permission from ref. 77. Copyright (2008) Wiley-VCH Verlag GmbH & Co. KGaA, Weinheim. | |
CuI-catalyzed azide–alkyne 1,3-dipolar cycloaddition (CuAAC), namely the click reaction, has been widely used for highly efficient bioconjugation.76 To this end, Wang and coworkers reported that TMV was first treated with diazonium salt 6 (in situ generated from 5) to obtain alkyne-labeled capsids 7, and then the tandem CuAAC reactions were employed for TMV modifications to obtain single and double click adducts 8 and 9 (Fig. 4b).77 This optimized method was successfully used to program the surface properties of TMV that can modulate cell behaviors grown on TMV-based biomaterials. Further examples of diazonium labeling include the installation of antigens78 and peptides79 on the TMV Tyr residues. Moreover, free radical oxidation of the TMV Tyr also led to acrylate-functionalized viruses with customizable properties.80
Though the TMV modifications using diazonium coupling are successful,77–79 one barrier to the widespread use of these diazonium salts is the prerequisite of in situ preparation from anilines under strongly acidic conditions at 0 °C before use. In addition, such in situ synthesized salts may bring difficulty in controlling the stoichiometry of the reaction. To this end, Barbas and coworkers developed the first bench-stable crystalline diazonium salt (11) that can be used for tyrosine-selective modification of peptides and proteins to introduce the aldehyde tags suitable for classical oxime and hydrazide ligations.8111 was synthesized on a gram scale from commercially available 4-aminobenzaldehyde polymer 10 (Fig. 5a), and the hexafluorophosphate counterion was essential for the stability of the diazonium salt. Direct mixture of 11 and proteins generated the labeled protein 12 with the aldehyde tags suitable for the classical hydrazide ligation to obtain 13 (Fig. 5b). Compared with the diazonlium coupling using the in situ synthesized salts, the protein labeling by the reagent 11 is much more facile for experimental operation. In addition, this bioconjugate technique was used for the facile introduction of functional tags onto model proteins and even to label the surface of live cells.
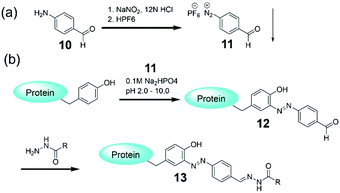 |
| Fig. 5 (a) Synthesis of a diazonium reagent 11. (b) Protein labeling via the reagent 11 followed by an efficient hydrazone ligation chemistry.81 | |
Consequently, Xi, Yi, and coworkers reported that a bench-stable diazonium reagent 14 could be used to label the TMV surface to incorporate thiols for further fluorescence labeling by 15 and gold coating (Fig. 6a).65 On the other hand, based on insights into the TMV assembly mechanism,40 we envisioned that the length of the TMV particle can be controlled by the length of the encapsulated viral RNA, and the Xi group at Nankai University has made efforts to use in vitro transcribed RNAs at different but controllable lengths to achieve VLPs with controllable lengths (Fig. 6b).82 It is noted that Yi and coworkers had also synthesized controllable short TMV-like nanorods (less than 60 nm) based on the self-assembly,67 but we prepared virus-like assemblies up to 400 nm (longer than the wide-type TMV) in a controllable fashion. Furthermore, we can easily modulate both the length of VLPs and the type of surface functionality via the reagent 14. For example, thiol-labeled VLPs were incubated with Cd2+ followed by treatment with H2S to generate a thin layer of CdS coating on the surface of VLPs. It is noted that CdS could be coated on different lengths of virus-like assemblies to generate CdS nanorods with controllable sizes, which represents a new synthetic route for a material tenability. Additionally, there should be plenty of room in the field of the combination of VLPs and chemical labeling for the controllable synthesis of nanocomposites.
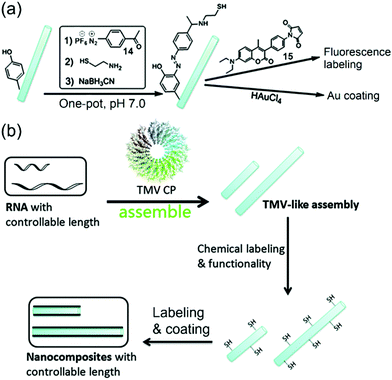 |
| Fig. 6 (a) The schematic drawing of the TMV labeling via the reagent 14 followed by cysteamine and reduction in one pot to produce thiolated TMV (TMV-SH). The TMV-SH could react with 7-diethylamino-3-(4-maleimidophenyl)-4-methylcoumarin (15) or chloroauric acid (HAuCl4). (b) A strategy for the fabrication of size-controllable 1D nanocomposites based on TMV-like assemblies as templates.65 Reprinted with permission from ref. 65. Copyright (2014), Royal Society of Chemistry. | |
Direct introduction of the click groups into proteins/viruses is the key step for further facile bioconjugation. For addressing this issue, Yi, Xi, and coworkers have reported a diazonium salt reagent for facile covalent incorporation of alkyne groups into proteins under mild conditions.83 The reagent 17 was facilely synthesized from commercially available and cheap 16, yielding light-yellow precipitates (57% yield) with good stability under common conditions (Fig. 7a). The small-molecule model studies allowed us to determine the reaction rate as 5.7 M−1 s−1 at pH 8.0, which is about 4.7-fold faster than that at pH 7.0. Moreover, strong fluorescence labeling of bovine serium albumin (BSA) was achieved after the reaction with 17 followed by the click reaction with 20 in one pot (Fig. 7b). More importantly, fluorescence labeling of TMV coated proteins was also achieved by the method (Fig. 7c), and transmission electron microscopy image (TEM) showed that TMV particles were not destroyed by the labeling reactions (Fig. 7d). Considering the mild reaction conditions and facile operations of the labeling strategy, we expect that the method based on the reagent 17 can be extended to other protein materials and plant viruses in the future.
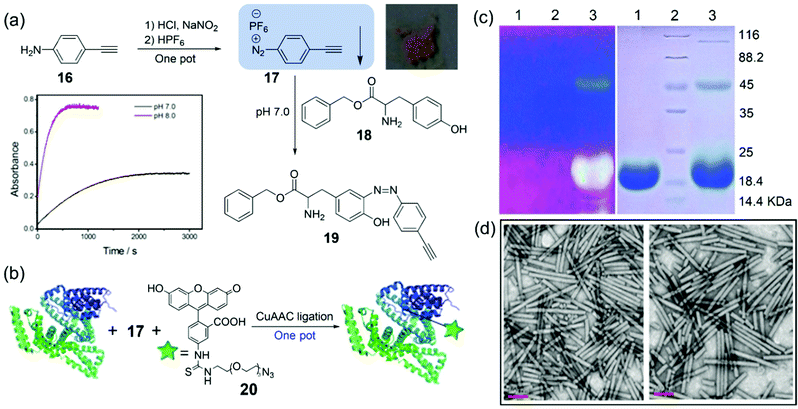 |
| Fig. 7 (a) Synthesis of a diazonium reagent 17 and its reaction with Tyr-containing 18 to generate 19, as identified using the HRMS spectrum. Inset: The intensities at 400 nm versus reaction time for 17 upon treatment with 18 in PBS at pH 7.0 (black line) or pH 8.0 (red line). (b) The fluorescence labeling reactions of BSA via17 followed by 20 in one pot. (c) 10% SDS PAGE of TMV before (lane 1) and after (lane 3) fluorescence labeling by 17 and 18 under a UV lamp or Coomassie blue staining, respectively. (d) TEM images of TMV before and after fluorescence labeling, respectively.83 Reprinted with permission from ref. 83. Copyright (2014), Royal Society of Chemistry. | |
Though several bench-stable diazonium reagents were successfully applied to directly incorporate aldehyde, ketone, or alkyne groups into Tyr residues of proteins, the next-step bioorthogonal reactions based on these reagents may suffer from low reaction efficiency or the need for catalysis. The inverse electron demand Diels–Alder reaction (IEDDA) or tetrazine-ene reaction is one of the best bioorthogonal reactions for catalyst-free covalent ligation.72,74,84,85 Therefore, Yi, Lv, and coworkers developed a tetrazine-containing diazonium reagent 21 (Fig. 8a).86 The bench-stable and water-soluble 21 was successfully employed for the direct, efficient and covalent introduction of tetrazines onto target proteins or virus surfaces. This tetrazinylation was further applied for the tetrazine-ene ligation to achieve protein PEGylation under very mild conditions. The tetrazine-containing TMV was confirmed using mass spectra with an increased mass of 420 Da of the TMV coat protein (the calculated mass, 417 Da) as well as fluorescence labeling using the norbornylene-containing fluorescent dye. In addition, we further demonstrated the utility of 21 through its direct incorporation into total cell lysates. Compared with 17, reagent 21 is more advantageous because the tetrazine-ene ligation is catalyst-free and has tunable rates by choosing different alkenes or alkynes in need. We believe that Tyr labeling based on 21 could provide a useful toolbox for the preparation of new materials in the future.
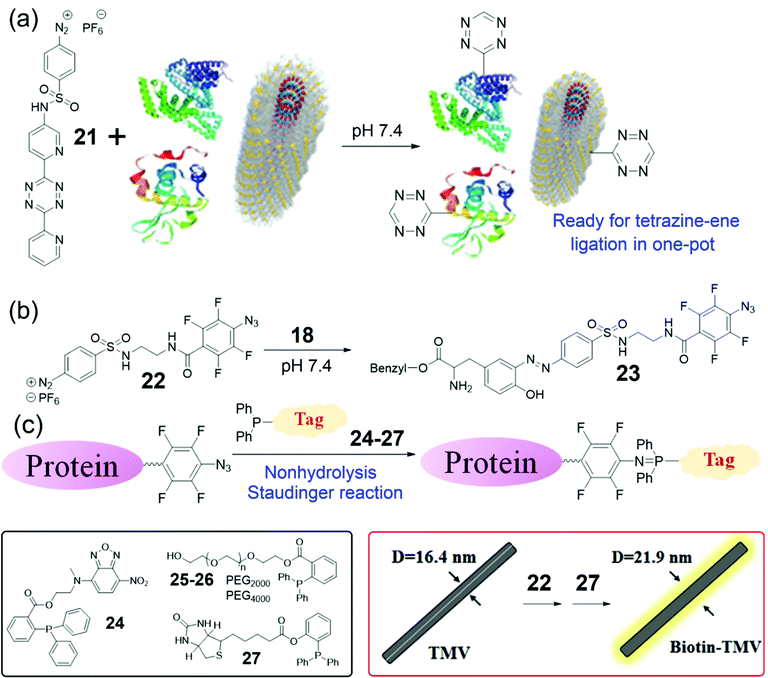 |
| Fig. 8 (a) A tetrazine-containing diazonium reagent 21 for protein/Tyr labeling.86 (b) Reaction of an azide-containing reagent 22 and 18 to form the azo product 23. (c) Schematic illustration of the NSR labeling of tetrafluorinated aromatic azide-labeled proteins. Chemical structures of reagents 24–27 and the biotinylation of TMV are also shown.90 | |
Though the tetrazine-ene ligation is useful for labeling of TMV, other catalysis-free, efficient, and facile bioorthogonal reactions may also be applied for such bioconjugation. In 2017, Yi, Wang, and coworkers found that the o,o′-difluorinated aryl azide could react efficiently with triphenylphosphine to produce a water-stable phosphanimine, which is called nonhydrolysis Staudinger reactions (NSRs).87 Consequently, Xi, Yi, and coworkers employed a tetrafluorinated aryl azide for the development of a faster NSR for protein and RNA labeling.88 The reaction kinetics of this NSR could be monitored by a fluorescence method to generate the reaction rate as 51.8 M−1 s−1, which is the fastest Staudinger ligation in aqueous buffer up to date. It is noted that Yan, Ramström, and co-workers independently discovered a similar NSR and determined the reaction rate as 18 M−1 s−1 by an NMR method.89 All the above NSR are facile, fast, and bioorthogonal for bioconjugation in vitro and in live cells.
To extend the applications of the NSR, Yi, Zhu, and coworkers envisioned that the o,o′,m,m′-tetrafluorinated aromatic azides could be incorporated into proteins and viruses through the diazonium coupling for subsequent NSR labeling. To this end, we developed a highly efficient and bench-stable reagent 22 and investigated the small-molecule model reaction between 22 and Tyr-containing 18 to generate 23 (Fig. 8b).90 Consequently, protein fluorescence labeling, PEGylation, and biotinylation were achieved by further functionalization of the azido-labeled proteins via the fast NSR by 24–27 (Fig. 8c). To our delight, the whole protein labeling processes could be finished in one pot within several hours under catalysis-free conditions.
Though TMV nanoparticles have been widely used as templates for controllable syntheses of nanomaterials,60–65 the diameters of native viruses are restricted to 18 nm. To address this issue, the highly efficient method based on 22 was applied to adjust the size of native viruses (Fig. 8b). The Tyr139 residues of TMV were labeled by 22 followed by 27 (Fig. 8c), and TEM analysis suggested that the diameter of 22–27-TMV rods was increased to 21.8 ± 3.5 nm. Such thickening of viral particles can provide new nano-templates with tunable sizes and shapes compared with that of the wide-type virus.65,90 Compared with that based on reagent 21, the labeling strategy based on the diazo-azide reagent 22 is more facile for the synthesis procedures of the reagents. On the other hand, the reagent 21 provides hydrophilic handles on proteins while the reagent 22 provides hydrophobic handles on proteins. Therefore, the reagent 22 would also expand the toolbox for protein bioconjugation in chemical biology and biomaterials under different conditions.
3. Preparation of hydrogels from TMV and dual-diazonium reagents
Based on the success of the development of several bench-stable diazonium reagents for TMV modifications, we envisioned that dual-diazonium and multi-diazonium reagents might be developed for protein and virus crosslinking.24,91 In 2018, Xi, Yi, and coworkers reported a new water-soluble reagent 28 with double diazonium sites (Fig. 9a) that might link two Tyr residues to lead to the crosslinking of TMV particles 29 (Fig. 9b).24 The solid 28 was stable for bench use and storage at −20 °C for more than three months. The reactivity of 28 with Tyr is efficient with a rate of up to 19.6 M−1 s−1. We proposed that a 3D network could be formed based on the efficient crosslinking of 28 and TMV (Fig. 9c). We optimized the gelation conditions and found that a direct mixture of the reagent 28 (2.5 mM) and TMV (2.5 mg mL−1) at 37 °C in neutral buffer (pH 7.0) for 30 min incubation led to the formation of a hydrogel (Fig. 9d).24 The TMV-based hydrogel was characterized by protein crosslinking and TEM images of 3D networks. In addition, scanning electron microscopy (SEM) analysis further supported the formation of 3D networks of the hydrogel.
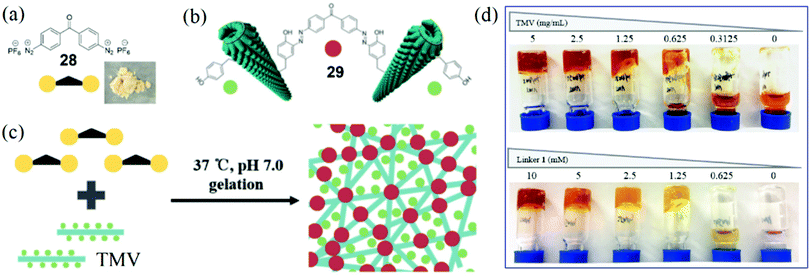 |
| Fig. 9 (a) Chemical structure of a dual-diazonium crosslinker 28 and photograph of the solid reagent. (b) Illustration of the crosslinking of two virus particles via the crosslinker. (c) Schematic representation of hydrogel formation from the reaction of 28 and TMV.24 (d) Photographs illustrating the effects of concentrations of TMV and 28 on the gelation. Reprinted with permission from ref. 24. Copyright (2018), Royal Society of Chemistry. | |
For the first virus-based hydrogel, we found that the 28-TMV hydrogel formation depended on both the crosslinking agent and the highly organized nanostructure of virus particles, and cleavage of the azo bonds by Na2S2O4 was useful for gel degradation. The hydrophobic anticancer drug camptothecin (CPT)92 did not affect the gelation and could be packaged using the 28-TMV hydrogel system, which implied the existence of enough hydrophobic cavities in the hydrogel. More importantly, for the CPT-containing 28-TMV hydrogel, half of the CPT release could be detected after 12 hours of dialysis, and 72% of the CPT release could be achieved after 3 days of dialysis. Moreover, the TMV-based hydrogel was safe for tobacco plants because TMV crosslinking could completely inactivate virus infection toward plants. Because the crosslinker reagent 28 can be easily prepared from commercial chemicals and is stable, we believe that this strategy of direct crosslinking of viruses and 28 may provide a general approach to prepare various kinds of hydrogels for the future biomedical and agriculture applications.
Though the TMV-based hydrogel can be formed by using 28, we envisioned that more reactive linkers would result in more efficient gelation of TMV. To this end, Xi, Yi, and coworkers rationally designed and synthesized three new dual-diazonium reagents (30–32) to investigate the effects of substituents (CO, PO, and SO2) on the crosslinking efficiency and gelation conditions (Fig. 10a).91 We found that 30 showed much smaller k2 (18 M−1 s−1) than that of 31 (71 M−1 s−1), implying that substitutes linked to aromatic cycles could influence the activity of diazonium with Tyr. Compared with 28,24 the substitution of the CO group with the SO2 group greatly enhanced the reaction rate. In addition, the Hammett parameters of the substituents and the reaction rates can be correlated to generate the reaction constant (ρ = +0.45),91 which suggests that the electron-withdrawing substituents can accelerate the crosslinking reaction.
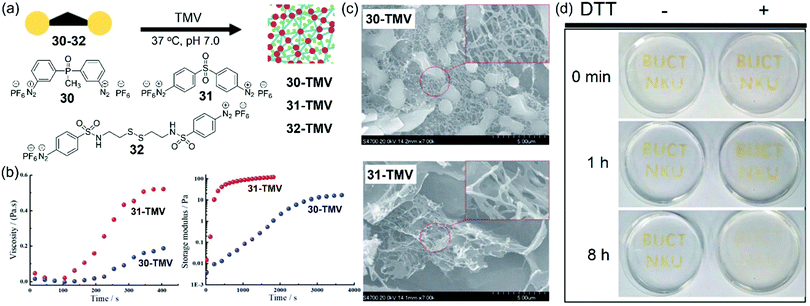 |
| Fig. 10 (a) Chemical structures of dual-diazonium reagents 30, 31, and 32 and their application for the gelation of TMV. (b) Rheological characteristics of two TMV-based hydrogels from 30 and 31. (c) SEM characterization of the TMV-based hydrogels. (d) Photographs for the TMV-based hydrogel degradation via DTT. The capitals were made from the crosslinking of TMV and 32 on the Petri dish. After gelation, the TMV hydrogel was immersed with DTT solution or deionized water.91 Reprinted with permission from ref. 91. Copyright (2019), Royal Society of Chemistry. | |
Because of the faster diazonium-tyrosine coupling reaction, hydrogels from the crosslinking of TMV and the reagent 31 could be formed within 1 min at room temperature. We also tested the rheological characters of the hydrogels formed by TMV and 30 or 31 from the solution phase to gel phase. As shown in Fig. 10b, the TMV-based hydrogel with 31 showed higher viscosity (about 2.5-fold) than that with 30, and the hydrogel based on 31 had a much faster gelation speed with a relatively higher storage modulus than that based on 30. SEM images showed that multiple virus rods in the 30-based hydrogel was crosslinked to form a 3D net structure, while a large number of sheets and less net structure was observed in the 31-based hydrogel (Fig. 10c). Therefore, the crosslinking reagents could be used for tuning the properties of TMV-based hydrogels. Furthermore, we also developed a crosslinker 32 by introducing a disulfide bond on the basis of 31. The TMV-based hydrogels prepared from 32 could be used to make the capitals “BUCT” and “NKU” in the dish (Fig. 10d), which could be degraded by reduction of the disulfide bonds using dithiothreitol (DTT). We also propose that these bench-stable dual-diazonium reagents may provide a general approach to preparing diverse functional hydrogels from other biocompatible viruses for future biomedical applications.
4. Summary and outlook
This minireview summarizes the development and applications of stable diazonium reagents for TMV labeling and crosslinking to generate new biomaterials. We as well as others have synthesized a series of bench-stable diazonium reagents from the corresponding anilines in medium to high yields (Table 1). The hexafluorophosphate counterions are important for the stability of solids of these diazonium reagents. Protein labeling by the bench-stable diazonium reagents can be achieved at pH 7.4. The diazonium-tyrosine coupling efficiencies can be tuned by the different substituted moieties on the aryl ring, and the coupling rate was higher with stronger electron-withdrawing substituents. The sulfonyl group at the p-position of aryldiazonium salts should be a useful structural motif for highly efficient labeling of protein Tyr residues to incorporate into a functional handle. In addition, these reagents provide a toolbox for the introduction of various bioorthogonal groups into proteins for chemical biology and materials science (Table 1).
Table 1 Summary of properties and applications for synthetic diazonium reagents
Reagent number |
Yield (%) |
Color (solid) |
k
2, (M−1 s−1) |
The tandem reactions |
TMV functionalization |
N.D., no detection. |
11
|
42 |
Off-white |
N.D. |
Oxime ligation; hydrazide ligation |
N.D. |
14
|
22 |
Light-grey |
N.D. |
Imine formation; oxime ligation; hydrazide ligation |
Fluorescence labeling; Au coating; CdS coating |
17
|
57 |
Light-yellow |
5.7 (pH 8.0) |
CuAAC ligation |
Fluorescence labeling; PEGylation |
21
|
46 |
Red |
N.D. |
Tetrazine–ene ligation |
Fluorescence labeling |
22
|
50 |
Yellow |
N.D. |
Nonhydrolysis Staudinger reaction |
Fluorescence labeling; PEGylation; biotinylation |
28
|
46 |
Light-yellow |
19.6 |
— |
Virus-based hydrogels |
30
|
60 |
Off-white |
18.4 |
— |
Virus-based hydrogels |
31
|
78 |
Yellow |
71.2 |
— |
Virus-based hydrogels |
32
|
86 |
Yellow |
N.D. |
— |
Virus-based hydrogels |
Though bioorthogonal groups including aldehydes,81 ketones,65 alkynes,83 tetrazines,86 and tetrafluorinated aryl azides,90 have been successfully employed for bench-stable diazonium reagents, we believe that new diazonium reagents using other bioorthogonal groups (Table 2)93–105 can be further developed for biolabeling under different conditions. Based on our experiences, there should be at least two-point criteria for the bioorthogonal groups in diazonium reagents: (1) the groups should be acid resistant because of the strong acid synthesis conditions of diazonium salts; (2) the groups should not react with diazonium. In future work, functional groups like aryl iodide and aryl boronic acid can be used to synthesize new diazonium for biolabeling via bioorthogonal organopalladium reactions (e.g. Mizoroki–Heck, Suzuki–Miyaura, and Sonogashira reactions).93–98 Other groups of oxyamine, hydrazide, and cyanobenzothiazole (Table 2) may also be used for the development of diazonium reagents for catalysis-free biolabeling.99,100 In addition, some photo-induced reactive groups (e.g. 9,10-phenanthrenequinone and tetrazole)101–104 should be possible for the preparation of new bench-stable diazonium reagents.
Table 2 Selected bioorthogonal reactions for the future development of bench-stable diazonium reagents
Palladium-mediated ligation |
|
Oxime/hydrazone ligation |
|
Cysteine-based ligation |
|
Photo-induced 1,3-dipolar cycloadditions |
|
Visible light-initiated photoclick cycloaddition |
|
We also believe that different types of linkers (e.g. flexible PEG or rigid polyphenylene vinylene) could be introduced between the two diazonium cations to obtain new crosslinking reagents (Fig. 11a). Furthermore, new crosslinking reagents with three, four, and five diazonium tags (Fig. 11b) can be developed for the multiple cross-linking of biomolecules and viruses. Therefore, the development of bench-stable diazonium reagents is still in its infancy, and we hope that this review will facilitate the development of future reagents for biolabeling.
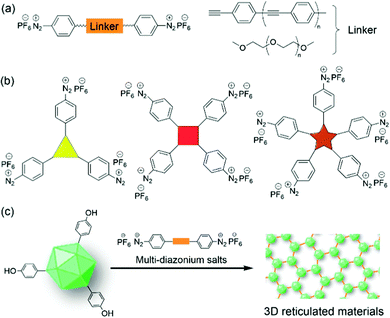 |
| Fig. 11 (a) Construction of new dual-diazonium reagents from different kinds of linkers (rigid or flexible structures). (b) Schematic illustration of triple-diazonium, quadruple-diazonium and quintuple-diazonium reagents. (c) Crosslinking of spherical viruses and multi-diazonium salts to generate 3D reticulated materials. | |
On the other hand, the size, length, and diameter of native TMV can be tuned in a controllable fashion by the combination of molecular biology and chemical biology methods. Therefore, there should be extremely large space for the construction of these TMV-based VLPs as nanotemplates for (in)organics as well as scaffolds for biotechnology. Additionally, the labeling of other plant virus nanostructures via the diazonium reagents will provide large chemical space for further modification and functionalization. For example, the preparation and properties of other biopolymer materials based on the combination of multi-diazonium reagents and other plant viruses can be further explored to provide novel 3D reticulated materials (Fig. 11c). Moreover, the plant virus-based hydrogels could be extended for biomedical and agriculture applications, including packing and releasing the antitumor drugs, defending plants from pathogenic attack, and so on. All in all, we hope that this minireview will facilitate the further development of multiple diazonium salts and their labeling with proteins/viruses for the preparation of new biomaterials including hydrogels.
Conflicts of interest
The authors declare no competing financial interests.
Acknowledgements
We thank Prof. Zhen Xi at Nankai University for kind support. This work was supported by NSFC (21572019, 21877008 and 22177010).
Notes and references
- C. Zhang, B. Wu, Y. Zhou, F. Zhou, W. Liu and Z. Wang, Mussel-inspired hydrogels: from design principles to promising applications, Chem. Soc. Rev., 2020, 49, 3605–3637 RSC.
- E. M. Ahmed, Hydrogel: preparation, characterization, and applications: a review, J. Adv. Res., 2015, 6, 105–121 CrossRef CAS PubMed.
- W. F. Lai and Z. D. He, Design and fabrication of hydrogel-based nanoparticulate systems for in vivo drug delivery, J. Controlled Release, 2016, 243, 269–282 CrossRef CAS PubMed.
- B.-X. Wang, W. Xu, Z. Yang and Y. Wu, and F. Pi, An overview on recent progress of the hydrogels: from material resources, properties, to functional applications, Macromol. Rapid Commun., 2022, 2100785 CrossRef CAS PubMed.
- S. Mantha, S. Pillai, P. Khayambashi, A. Upadhyay, Y. Zhang, O. Tao, H. M. Pham and S. D. Tran, Smart hydrogels in tissue engineering and regenerative medicine, Materials, 2019, 12, 3323 CrossRef CAS PubMed.
- L. Yang, R. Peltier, M. Zhang, D. Song, H. Huang, G. Chen, Y. Chen, F. Zhou, Q. Hao, L. Bian, M. L. He, Z. Wang, Y. Hu and H. Sun, Desuccinylation-triggered peptide self-assembly: live cell imaging of SIRT5 activity and mitochondrial activity modulation, J. Am. Chem. Soc., 2020, 142, 18150–18159 CrossRef CAS PubMed.
- S. An, E. J. Jeon, J. Jeon and S.-W. Cho, A serotonin-modified hyaluronic acid hydrogel for multifunctional hemostatic adhesives inspired by a platelet coagulation mediator, Mater. Horiz., 2019, 6, 1169–1178 RSC.
- S. Talebian, M. Mehrali, N. Taebnia, C. P. Pennisi, F. B. Kadumudi, J. Foroughi, M. Hasany, M. Nikkhah, M. Akbari, G. Orive and A. Dolatshahi-Pirouz, Self-healing hydrogels: the next paradigm shift in tissue engineering?, Adv. Sci., 2019, 6, 1801664 CrossRef PubMed.
- W. Li, Z. Yan, J. Ren and X. Qu, Manipulating cell fate: dynamic control of cell behaviors on functional platforms, Chem. Soc. Rev., 2018, 47, 8639–8684 RSC.
- S. Li, S. Dong, W. Xu, S. Tu, L. Yan, C. Zhao, J. Ding and X. Chen, Antibacterial hydrogels, Adv. Sci., 2018, 5, 1700527 CrossRef PubMed.
- Z. Wang, Y. Cong and J. Fu, Stretchable and tough conductive hydrogels for flexible pressure and strain sensors, J. Mater. Chem. B, 2020, 8, 3437–3459 RSC.
- H. Du, W. Liu, M. Zhang, C. Si, X. Zhang and B. Li, Cellulose nanocrystals and cellulose nanofibrils based hydrogels for biomedical applications, Carbohydr. Polym., 2019, 209, 130–144 CrossRef CAS PubMed.
- J. Li, R. Xing, S. Bai and X. Yan, Recent advances of self-assembling peptide-based hydrogels for biomedical applications, Soft Matter, 2019, 15, 1704–1715 RSC.
- M. C. Catoira, L. Fusaro, D. Di Francesco, M. Ramella and F. Boccafoschi, Overview of natural hydrogels for regenerative medicine applications, J. Mater. Sci.: Mater. Med., 2019, 30, 115 CrossRef PubMed.
- L. Li, J. M. Scheiger and P. A. Levkin, Design and applications of photoresponsive hydrogels, Adv. Mater., 2019, 31, 1807333 CrossRef PubMed.
- S. Yigit, R. Sanyal and A. Sanyal, Fabrication and functionalization of hydrogels through “click” chemistry, Chem. – Asian J., 2011, 6, 2648–2659 CrossRef CAS PubMed.
- W. Hu, Z. Wang, Y. Xiao, S. Zhang and J. Wang, Advances in crosslinking strategies of biomedical
hydrogels, Biomater. Sci., 2019, 7, 843–855 RSC.
- A. C. Daly, L. Riley, T. Segura and J. A. Burdick, Hydrogel microparticles for biomedical applications, Nat. Rev. Mater., 2020, 5, 20–43 CrossRef CAS PubMed.
- H. He, W. Tan, J. Guo, M. Yi, A. N. Shy and B. Xu, Enzymatic noncovalent synthesis, Chem. Rev., 2020, 120, 9994–10078 CrossRef CAS PubMed.
- C. Jiang, H. Huang, X. Kang, L. Yang, Z. Xi, H. Sun, M. D. Pluth and L. Yi, NBD-based synthetic probes for sensing small molecules and proteins: design, sensing mechanisms and biological applications, Chem. Soc. Rev., 2021, 50, 7436–7495 RSC.
- K. L. Shantha and D. R.-K. Harding, Synthesis and evaluation of sucrose-containing polymeric hydrogels for oral drug delivery, J. Appl. Polym. Sci., 2002, 84, 2597–2604 CrossRef CAS.
- D. A. Gyles, L. D. Castro, J. O.-C. Silva and R. M. Ribeiro-Costa, A review of the designs and prominent biomedical advances of natural and synthetic hydrogel formulations, Eur. Polym. J., 2017, 88, 373–392 CrossRef CAS.
- X. Xu, V. V. Jerca and R. Hoogenboom, Bioinspired double network hydrogels: from covalent double network hydrogels via hybrid double network hydrogels to physical double network hydrogels, Mater. Horiz., 2021, 8, 1173–1188 RSC.
- D. Ma, J. Zhang, C. Zhang, Y. Men, H. Sun, L. Y. Li, L. Yi and Z. Xi, A highly efficient dual-diazonium reagent for protein crosslinking and construction of a virus-based gel, Org. Biomol. Chem., 2018, 16, 3353–3357 RSC.
- C. Dickmeis, L. Kauth and U. Commandeur, From infection to healing: the use of plant viruses in bioactive hydrogels, Wiley Interdiscip. Rev.: Nanomed. Nanobiotechnol., 2021, 13, e1662 CAS.
- A. M. Wen and N. F. Steinmetz, Design of virus-based nanomaterials for medicine, biotechnology, and energy, Chem. Soc. Rev., 2016, 45, 4074–4126 RSC.
- E. M. Plummer and M. Manchester, Viral nanoparticles and virus-like particles: platforms for contemporary vaccine design, Wiley Interdiscip. Rev.: Nanomed. Nanobiotechnol., 2011, 3, 174–196 CAS.
- I. Yildiz, S. Shukla and N. F. Steinmetz, Applications of viral nanoparticles in medicine, Curr. Opin. Biotechnol, 2011, 22, 901–908 CrossRef CAS PubMed.
- K. Z. Lee, V. B. Pussepitiyalage, Y.-H. Lee, L. S. Loesch-Fries, M. T. Harris, S. Hemmati and K. V. Solomon, Engineering tobacco mosaic virus and its virus-like-particles for synthesis of biotemplated nanomaterials, Biotechnol. J., 2021, 16, 2000311 CrossRef CAS PubMed.
- D. S. Peabody, A viral platform for chemical modification and multivalent display, J. Nanobiotechnol., 2003, 1, 1–8 CrossRef PubMed.
- A. D. Brown, L. Naves, X. Wang, R. Ghodssi and J. N. Culver, Carboxylate-directed in vivo assembly of virus-like nanorods and tubes for the display of functional peptides and residues, Biomacromolecules, 2013, 14, 3123–3129 CrossRef CAS PubMed.
- K. J. Koudelka, G. Destito, E. M. Plummer, S. A. Trauger, G. Siuzdak and M. Manchester, Endothelial targeting of cowpea mosaic virus (CPMV) via surface vimentin, PLoS Pathog., 2009, 5, e1000417 CrossRef PubMed.
- V. Vignali, B. S. Miranda, I. Lodoso-Torrecilla, C. A.-J. van Nisselroy, B. J. Hoogenberg, S. Dantuma, F. Hollmann, J. W. de Vries, E. M. Warszawik, R. Fischer, U. Commandeur and P. van Rijn, Biocatalytically induced surface modification of the tobacco mosaic virus and the bacteriophage M13, Chem. Commun., 2018, 55, 51–54 RSC.
- T. Douglas and M. Young, Host-guest encapsulation of materials by assembled virus protein cages, Nature, 1998, 393, 152 CrossRef CAS.
- J. N. Culver, Tobacco mosaic virus assembly and disassembly: determinants in pathogenicity and resistance, Annu. Rev. Phytopathol., 2002, 40, 287–308 CrossRef CAS PubMed.
- M.-C. Daniel, I. B. Tsvetkova, Z. T. Quinkert, A. Murali, M. De, V. M. Rotello, C. C. Kaoand and B. Dragnea, Role of surface charge density in nanoparticle-templated assembly of bromovirus protein cages, ACS Nano, 2010, 4, 3853–3860 CrossRef CAS PubMed.
- A. J. Love, V. Makarov, I. Yaminsky, N. O. Kalinina and M. E. Taliansky, The use of tobacco mosaic virus and cowpea mosaic virus for the production of novel metal nanomaterials, Virology, 2014, 449, 133–139 CrossRef CAS PubMed.
- D. J. Evans, The bionanoscience of plant viruses: templates and synthons for new materials, J. Mater. Chem., 2008, 18, 3746–3754 RSC.
- K.-B. G. Scholthof, Tobacco mosaic virus: a model system for plant biology, Annu. Rev. Phytopathol., 2004, 42, 13–34 CrossRef CAS PubMed.
- A. Klug, The tobacco mosaic virus particle: structure and assembly, Philos. Trans. R. Soc., B, 1999, 354, 531–535 CrossRef CAS PubMed.
- P. Liu, Y. Ning, Q. Zhou, G. Mao and Z. Niu, One-dimensional rod-like tobacco mosaic virus: self-assembly and applications, Prog. Chem., 2015, 27, 1425–1434 CAS.
- M. Knez, M. Sumser, A. M. Bittner, C. Wege, H. Jeske, T. P. Martin and K. Kern, Spatially selective nucleation of metal clusters on the tobacco mosaic virus, Adv. Funct. Mater., 2004, 14, 116–124 CrossRef CAS.
- L. Wu, J. Zang, L. A. Lee, Z. Niu, G. C. Horvatha, V. Braxtona, A. C. Wibowo, M. A. Bruckman, S. Ghoshroy, H.-C. zur Loye, X. Li and Q. Wang, Electrospinning fabrication, structural and mechanical characterization of rod-like virus-based composite nanofibers, J. Mater. Chem., 2011, 21, 8550–8557 RSC.
- T. L. Schlick, Z. Ding, E. W. Kovacs and M. B. Francis, Dual-surface modification of the tobacco mosaic virus, J. Am. Chem. Soc., 2005, 127, 3718–3723 CrossRef CAS PubMed.
- M. A. Bruckman and N. F. Steinmetz, Chemical modification of the inner and outer surfaces of tobacco mosaic virus (TMV), Methods Mol. Biol., 2014, 1108, 173–185 CrossRef CAS PubMed.
- M. W. Jones, G. Mantovani, C. A. Blindauer, S. M. Ryan, X. Wang, D. J. Brayden and D. M. Haddleton, Direct peptide bioconjugation/PEGylation at tyrosine with linear and branched polymeric diazonium salts, J. Am. Chem. Soc., 2012, 134, 7406–7413 CrossRef CAS PubMed.
- S. Chen and M.-L. Tsao, Genetic incorporation of a 2-naphthol group into proteins for site-specific azo coupling, Bioconjugate Chem., 2013, 24, 1645–1649 CrossRef CAS PubMed.
- E. Royston, A. Ghosh, P. Kofinas, M. T. Harris and J. N. Culver, Self-assembly of virus-structured high surface area nanomaterials and their application as battery electrodes, Langmuir, 2008, 24, 906–912 CrossRef CAS PubMed.
- H. Yi, S. Nisar, S.-Y. Lee, M. A. Powers, W. E. Bentley, G. F. Payne, R. Ghodssi, G. W. Rubloff, M. T. Harris and J. N. Culver, Patterned assembly of genetically modified viral nanotemplates via nucleic acid hybridization, Nano Lett., 2005, 5, 1931–1936 CrossRef CAS PubMed.
- U. Gallwize, L. King and R. N. Perham, Preparation of an isomorphous heavy-atom derivative of tobacco mosaic virus by chemical modification with 4-sulpho-phenylisothiocyanate, J. Mol. Biol., 1974, 87, 257–260 CrossRef.
- M. L. Smith, J. A. Lindbo, S. Dillard-Telm, P. M. Brosio, A. B. Lasnik, A. A. McCormick, L. V. Nguyen and K. E. Palmer, Modified tobacco mosaic virus particles as scaffolds for display of protein antigens for vaccine applications, Virology, 2006, 348, 475–488 CrossRef CAS PubMed.
- L. A. Lee, H. G. Nguyena and Q. Wang, Altering the landscape of viruses and bionanoparticles, Org. Biomol. Chem., 2011, 9, 6189–6195 RSC.
- R. A. Miller, A. D. Presley and M. B. Francis, Self-assembling light-harvesting systems from synthetically modified tobacco mosaic virus coat proteins, J. Am. Chem. Soc., 2007, 129, 3104–3109 CrossRef CAS PubMed.
- X. Liu, B. Liu, S. Gao, Z. Wang, Y. Tian, M. Wu, S. Jiang and Z. Niu, Glyco-decorated tobacco mosaic virus as a vector for cisplatin delivery, J. Mater. Chem. B, 2017, 5, 2078–2085 RSC.
- D. L. Kernan, A. M. Wen, A. S. Pitek and N. F. Steinmetz, Delivery of chemotherapeutic vcMMAE using tobacco mosaic virus nanoparticles, Exp. Biol. Med., 2017, 242, 1405–1411 CrossRef CAS PubMed.
- O. Jacobson, D. O. Kiesewetter and X. Chen, Interface of physics and biology: engineering virus-based nanoparticles for biophotonics, Bioconjugate Chem., 2015, 26, 51–62 CrossRef PubMed.
- Z. Yin, H. G. Nguyen, S. Chowdhury, P. Bentley, M. A. Bruckman, A. Miermont, J. C. Gildersleeve, Q. Wang and X. Huang, Tobacco mosaic virus as a new carrier for tumor associated carbohydrate antigens, Bioconjugate Chem., 2012, 23, 1694–1703 CrossRef CAS PubMed.
- Y. Tian, S. Gao, M. Wu, X. Liu, J. Qiao, Q. Zhou, S. Jiang and Z. Niu, Tobacco mosaic virus-based 1D nanorod-drug carrier via the integrin-mediated endocytosis pathway, ACS Appl. Mater. Interfaces, 2016, 8, 10800–10807 CrossRef CAS PubMed.
- A. E. Czapar, Y.-R. Zheng, I. A. Riddell, S. Shukla, S. G. Awuah, S. J. Lippard and N. F. Steinmetz, Tobacco mosaic virus delivery of phenanthriplatin for cancer therapy, ACS Nano, 2016, 10, 4119–4126 CrossRef CAS PubMed.
- S. Chu, A. D. Brown, J. N. Culver and R. Ghodssi, Tobacco mosaic virus as a versatile platform for molecular assembly and device fabrication, Biotechnol. J., 2018, 13, 1800147 CrossRef PubMed.
- X. Z. Fan, E. Pomerantseva, M. Gnerlich, A. Brown, K. Gerasopoulos, M. McCarthy, J. Culver and R. Ghodssi, Tobacco mosaic virus: a biological building block for micro/nano/bio systems, J. Vac. Sci. Technol., A, 2013, 31, 050815 CrossRef.
- C. Koch, F. J. Eber, C. Azucena, A. Förste, S. Walheim, T. Schimmel, A. M. Bittner, H. Jeske, H. Gliemann, S. Eiben, F. C. Geiger and C. Wege, Novel roles for well-known players: from tobacco mosaic virus pests to enzymatically active assemblies, Beilstein J. Nanotechnol., 2016, 7, 613–629 CrossRef CAS PubMed.
- C. Hou, H. Xu, X. Jiang, Y. Li, S. Deng, M. Zang, J. Xu and J. Liu, Virus-based supramolecular structure and materials: concept and prospects, ACS Appl. Bio Mater., 2021, 4, 5961–5974 CrossRef CAS PubMed.
- M. A. Bruckman, S. Hern, K. Jiang, C. A. Flask, X. Yu and N. F. Steinmetz, Tobacco mosaic virus rods and spheres as supramolecular high-relaxivity MRI contrast agents, J. Mater. Chem. B, 2013, 1, 1482–1490 RSC.
- D. Ma, Y. Xie, J. Zhang, D. Ouyang, L. Yi and Z. Xi, Self-assembled controllable virus-like nanorods as templates for construction of one-dimensional organic–inorganic nanocomposites, Chem. Commun., 2014, 50, 15581–15584 RSC.
- Z. Niu, M. Bruckman, V. S. Kotakadi, J. He, T. Emrick, T. P. Russell, L. Yang and Q. Wang, Study and characterization
of tobacco mosaic virus head-to-tail assembly assisted by aniline polymerization, Chem. Commun., 2006, 3019–3021 RSC.
- J. M. Rego, J.-H. Lee, D. H. Lee and H. Yi, Biologically inspired strategy for programmed assembly of viral building blocks with controlled dimensions, Biotechnol. J., 2013, 8, 237–246 CrossRef CAS PubMed.
- C. P. Ramil and Q. Lin, Bioorthogonal chemistry: strategies and recent developments, Chem. Commun., 2013, 49, 11007–11022 RSC.
- C. S. McKay and M. G. Finn, Click chemistry in complex mixtures: bioorthogonal bioconjugation, Chem. Biol., 2014, 21, 1075–1101 CrossRef CAS PubMed.
- O. Boutureira and G. J.-L. Bernardes, Advances in chemical protein modification, Chem. Rev., 2015, 115, 2174–2195 CrossRef CAS PubMed.
- J. Li and P. R. Chen, Development and application of bond cleavage reactions in bioorthogonal chemistry, Nat. Chem. Biol., 2016, 12, 129–137 CrossRef CAS PubMed.
- B. L. Oliveira, Z. Guo and G. J.-L. Bernardes, Inverse electron demand Diels–Alder reactions in chemical biology, Chem. Soc. Rev., 2017, 46, 4895–4950 RSC.
- T. K. Heiss, R. S. Dorn and J. A. Prescher, Bioorthogonal reactions of triarylphosphines and related analogues, Chem. Rev., 2021, 121, 6802–6849 CrossRef CAS PubMed.
- F.-G. Zhang, Z. Chen, X. Tang and J.-A. Ma, Triazines: syntheses and inverse electron-demand Diels−Alder reactions, Chem. Rev., 2021, 121, 14555–14593 CrossRef CAS PubMed.
- V. M. Lechner, M. Nappi, P. J. Deneny, S. Folliet, J. C.-K. Chu and M. J. Gaunt, Visible-light-mediated modification and manipulation of biomacromolecules, Chem. Rev., 2022, 122, 1752–1829 CrossRef CAS PubMed.
- V. V. Rostovtsev, L. G. Green, V. V. Fokin and K. B. Sharpless, A stepwise Huisgen cycloaddition process: copper(I)-catalyzed regioselective “ligation” of azides and terminal alkynes, Angew. Chem., Int. Ed., 2002, 41, 2596–2599 CrossRef CAS PubMed.
- M. A. Bruckman, G. Kaur, L. A. Lee, F. Xie, J. Sepulveda, R. Breitenkamp, X. Zhang, M. Joralemon, T. P. Russell, T. Emrick and Q. Wang, Surface modification of tobacco mosaic virus with “click” chemistry, ChemBioChem, 2008, 9, 519–523 CrossRef CAS PubMed.
- X. Zhao, L. Chen, J. A. Luckanagul, X. Zhang, Y. Lin and Q. Wang, Enhancing antibody response against small molecular hapten with tobacco mosaic virus as a polyvalent carrier, ChemBioChem, 2015, 16, 1279–1283 CrossRef CAS PubMed.
- P. Sitasuwan, L. A. Lee, K. Li, H. G. Nguyen and Q. Wang, RGD-conjugated rod-like viral nanoparticles on 2D scaffold improve bone differentiation of mesenchymal stem cells, Front. Chem., 2014, 2, 31 Search PubMed.
- V. Vignali, B. S. Miranda, I. Lodoso-Torrecilla, C. A.-J. van Nisselroy, B.-J. Hoogenberg, S. Dantuma, F. Hollmann, J. W. de Vries, E. M. Warszawik, R. Fischer, U. Commandeure and P. van Rijn, Biocatalytically induced surface modification of the tobacco mosaic virus and the bacteriophage M13, Chem. Commun., 2019, 55, 51–54 RSC.
- J. Gavrilyuk, H. Ban, M. Nagano, W. Hakamata and C. F. Barbas III, Formylbenzene diazonium hexafluorophosphate reagent for tyrosine-selective modification of proteins and the introduction of a bioorthogonal aldehyde, Bioconjugate Chem., 2012, 23, 2321–2328 CrossRef CAS PubMed.
-
L. Lv, The size control of tobacco mosaic virus nanoparticle and the modification of its coat protein, Master thesis, Nankai University, China, 2012 Search PubMed.
- J. Zhang, D. Ma, D. Du, Z. Xi and L. Yi, An efficient reagent for covalent introduction of alkynes into proteins, Org. Biomol. Chem., 2014, 12, 9528–9531 RSC.
- R. J. Blizzard, D. R. Backus, W. Brown, C. G. Bazewicz, Y. Li and R. A. Mehl, Ideal bioorthogonal reactions using a site-specifically encoded tetrazine amino acid, J. Am. Chem. Soc., 2015, 137, 10044 CrossRef CAS PubMed.
- J. Wang, X. Wang, X. Fan and P. R. Chen, Unleashing the power of bond cleavage chemistry in living systems, ACS Cent. Sci., 2021, 7, 929–943 CrossRef CAS PubMed.
- J. Zhang, Y. Men, S. Lv, L. Yi and J. F. Chen, Protein tetrazinylation via diazonium coupling for covalent and catalyst-free bioconjugation, Org. Biomol. Chem., 2015, 13, 11422–11425 RSC.
- J. Zhang, Y. Gao, X. Kang, Z. Zhu, Z. Wang, Z. Xi and L. Yi, o,o-Difluorination of aromatic azide yields a fast-response fluorescent probe for H2S detection and for improved bioorthogonal reactions, Org. Biomol. Chem., 2017, 15, 4212–4217 RSC.
- Y. Xie, L. Cheng, Y. Gao, X. Cai, X. Yang, L. Yi and Z. Xi, Tetrafluorination of aromatic azide yields a highly efficient Staudinger reaction: kinetics and biolabeling, Chem. – Asian J., 2018, 13, 1791–1796 CrossRef CAS PubMed.
- M. Sundhoro, S. Jeon, J. Park, O. Ramström and M. Yan, Perfluoroaryl azide Staudinger reaction: a fast and bioorthogonal reaction, Angew. Chem., Int. Ed., 2017, 56, 12117–12121 CrossRef CAS PubMed.
- D. Ma, X. Kang, Y. Gao, J. Zhu, L. Yi and Z. Xi, Design and synthesis of a highly efficient labelling reagent for incorporation of tetrafluorinated aromatic azide into proteins, Tetrahedron, 2019, 75, 888–893 CrossRef CAS.
- D. Ma, Z. Chen, L. Yi and Z. Xi, Development of improved dual-diazonium reagents for faster crosslinking of tobacco mosaic virus to form hydrogels, RSC Adv., 2019, 9, 29070–29077 RSC.
- M. Hridoy, M. Z.-H. Gorapi, S. Noor, N. S. Chowdhury, M. M. Rahman, I. Muscari, F. Masia, S. Adorisio, D. V. Delfino and M. A. Mazid, Putative anticancer compounds from plant-derived endophytic fungi: a review, Molecules, 2022, 27, 296 CrossRef CAS PubMed.
- M. Yang, J. Li and P. R. Chen, Transition metal-mediated bioorthogonal protein chemistry in living cells, Chem. Soc. Rev., 2014, 43, 6511–6526 RSC.
- K. Kodama, S. Fukuzawa, H. Nakayama, K. Sakamoto, T. Kigawa, T. Yabuki, N. Matsuda, M. Shirouzu, K. Takio, S. Yokoyama and K. Tachibana, Site-specific functionalization of proteins by organopalladium reactions, ChemBioChem, 2007, 8, 232–238 CrossRef CAS PubMed.
- N. Li, R. K.-V. Lim, S. Edwardraja and Q. Lin, Copper-free Sonogashira cross-coupling for functionalization of alkyne-encoded proteins in aqueous medium and in bacterial cells, J. Am. Chem. Soc., 2011, 133, 15316–15319 CrossRef CAS PubMed.
- J. Li, S. Lin, J. Wang, S. Jia, M. Yang, Z. Hao, X. Zhang and P. R. Chen, Ligand-free palladium-mediated site-specific protein labeling inside Gram-negative bacterial pathogens, J. Am. Chem. Soc., 2013, 135, 7330–7338 CrossRef CAS PubMed.
- S. V. Chankeshwara, E. Indrigo and M. Bradley, Palladium-mediated chemistry in living cells, Curr. Opin. Chem. Biol., 2014, 21, 128–135 CrossRef CAS PubMed.
- J. M. Chalker, C. S.-C. Wood and B. G. Davis, A convenient catalyst for aqueous and protein Suzuki-Miyaura cross-coupling, J. Am. Chem. Soc., 2009, 131, 16346–16347 CrossRef CAS PubMed.
- H. F. Gaertner, K. Rose, R. Cotton, D. Timms, R. Camble and R. E. Offord, Construction of protein analogues by site-specific condensation of unprotected fragments, Bioconjugate Chem., 1992, 3, 262–268 CrossRef CAS PubMed.
- H. Ren, F. Xiao, K. Zhan, Y.-P. Kim, H. Xie, Z. Xia and J. Rao, A biocompatible condensation reaction for the labeling of terminal cysteine residues on proteins, Angew. Chem., Int. Ed., 2009, 48, 9658–9662 CrossRef CAS PubMed.
- J. Li, H. Kong, L. Huang, B. Cheng, K. Qin, M. Zheng, Z. Yan and Y. Zhang, Visible light-initiated bioorthogonal photoclick cycloaddition, J. Am. Chem. Soc., 2018, 140, 14542–14546 CrossRef CAS PubMed.
- H. Huang, G. Zhang, L. Gong, S. Zhang and Y. Chen, Visible-light-induced chemoselective deboronative alkynylation under biomolecule-compatible conditions, J. Am. Chem. Soc., 2014, 136, 2280–2283 CrossRef CAS PubMed.
- Y. Wang, W. J. Hu, W. Song, R. K.-V. Lim and Q. Lin, Discovery of long-wavelength photoactivatable diaryltetrazoles for bioorthogonal 1,3-dipolar cycloaddition reactions, Org. Lett., 2008, 10, 3725–3728 CrossRef CAS PubMed.
- W. Song, Y. Wang, J. Qu, M. M. Madden and Q. Lin, A photoinducible 1,3-dipolar cycloaddition reaction for rapid, selective modification of tetrazole-containing proteins, Angew. Chem., Int. Ed., 2008, 47, 2832–2835 CrossRef CAS PubMed.
- T. Deb, J. Tu and R. M. Franzini, Mechanisms and substituent effects of metal-free bioorthogonal reactions, Chem. Rev., 2021, 121, 6850–6914 CrossRef CAS PubMed.
Footnote |
† These authors contributed equally to this work. |
|
This journal is © The Royal Society of Chemistry 2022 |
Click here to see how this site uses Cookies. View our privacy policy here.