DOI:
10.1039/D1QO00910A
(Research Article)
Org. Chem. Front., 2021,
8, 5280-5288
Efficient one-pot synthesis of [3]catenanes based on Pt(II) metallacycles with a flexible building block†
Received
17th June 2021
, Accepted 21st July 2021
First published on 21st July 2021
Abstract
Two quadrilateral metallacycles were prepared by coordination-driven self-assembly of a 90° platinum(II) receptor 6 with a flexible donor 1,3-bis(4,4′-bipyridinium)propane 1a or 1,1-bis(4,4′-bipyridinium)methane 1b, respectively. Three [3]catenanes (3, 4 and 5) were further prepared by in situ addition of crown ethers (DB24C8 or DB30C10) to the yielded metallacycles. By comparing the crystal structures of 1a and 4, it was found that the bite angle change of the flexible bidentate donor 1a (111.0° to 112.3°) is much smaller than that of rigid platinum(II) receptor 6 (90.0° to 83.2°) during metalla-cyclization. The multicomponent [3]catenanes were stabilized by multiple supramolecular interactions, including metal coordination, π–π stacking, charge transfer, and hydrogen bonding.
Introduction
Mechanically interlocked molecules (MIMs) are molecules consisting of subcomponents that are connected by mechanical bonds.1 They have gained much attention from chemists because of not only their unique topological architectures but also their prosperous applications in molecular machines, molecular devices, and smart materials.2 Catenanes are a type of MIM composed of two or more mechanically interlocked macrocyclic parts.3 In the past three decades, template-directed strategies for the synthesis of catenanes and other MIMs, such as rotaxanes, Solomon links, and Borromean rings and knots, have been developed impressively.4 However, the highly efficient preparation of catenanes is still a challenge due to the formation of unexpected polymeric or non-interlocked species during the macrocyclization processes.3e–g In particular, when kinetically controlled reactions are used in the final interlocking step, the thermodynamically favorable but kinetically unfavorable nature of macrocyclization processes will hinder the formation of interlocked products. Instead, the use of dynamic covalent chemistry (DCC, such as imine bond formation, disulfide bond formation, and olefin metathesis) and coordination bonds in the final interlocking step can promote the transformations of unexpected intermediates and byproducts into thermodynamically favored MIMs.5 The reversible feature enables product distribution under thermodynamic control, which means that the MIMs with higher thermodynamic stability will be produced with higher efficiency as the reaction reaches an equilibrium.6
Coordination-driven self-assembly has great advantages, including various but predictable geometries, high bonding energy, good bond direction and good reversibility.7 These intrinsic features make it a promising method for the construction of discrete supramolecular coordination complexes (SCCs). A variety of metallacycles, metallacages and other discrete SCCs with fascinating architectures have been prepared through rational design and careful selection of the metal and organic ligands.7,8 Because of its modularity and versatility, coordination-driven self-assembly is also a powerful and facile route to provide metal-based macrocyclic supramolecular elements9 for the construction of MIMs from simple precursors.10 A number of metal-connected MIMs, including catenanes, rotaxanes, molecular necklaces, Solomon links, and Borromean rings and konts, have been constructed by using this method.11 The complementary building blocks used in coordination-driven self-assembly are usually structurally rigid conjugated molecules with predefined bite angles.7 However, the balance of flexibility and rigidity of the ligands also plays a key role in the construction of SCCs. Building blocks with certain flexibility can allow dynamic adaption in the coordination-driven self-assembly, but very flexible linkers are not applicable in most cases. The balance of flexibility and rigidity is also significant in the preparation of catenanes. The use of considerably long and highly flexible linkers usually results in the formation of [2]catenanes during the cyclization processes.3h In order to obtain high-order catenanes with more sub-components, shorter linkers with less flexibility are generally selected. Previously, we have reported the fabrication of metallacycles and metalla-catenanes through Pt(II)-based coordination-driven self-assembly using the 1,2-bis(pyridinium)ethane/dibenzo-24-crown-8 (DB24C8) recognition motif, wherein 1,2-bis(pyridinium)ethane with a slightly flexible ethylene linker acted as a 180° building block.12 Herein, we want to see whether a more flexible donor with a propylene linker, 1,3-bis(pyridinium)propane, can be used in the construction of SCCs and what bite angle it will have. As monitored by 1H NMR, 31P NMR, and mass spectroscopy and X-ray crystallography analyses, we found that not only quadrilateral metallacycles but also metalla-[3]catenanes can be efficiently prepared by employing this flexible bidentate donor.
From the reported results of Peinador13 and us,12 we knew that the bite angles of 1,1-bis(pyridinium)methane and 1,2-bis(pyridinium)ethane were about 109° and 180°, respectively. We speculated that if the sp3-hybridized propylene carbons are all in stable staggered conformations, the bite angle of 1,3-bis(pyridinium)propane should also be around 109° (Fig. S29†). Therefore, although 1,3-bis(pyridinium)propane is more flexible and has more conformers, it may coordinate with metal acceptors to form metallacycles like 1,1-bis(pyridinium)methane. Herein, both 1,3-bis(4,4′-bipyridinium)propane and 1,1-bis(4,4′-bipyridinium)methane were investigated and compared with each other as the building blocks for the Pt(II)-based coordination-driven self-assembly of metallacycles and metalla-catenanes.
Results and discussion
Synthesis of the bis(4,4′-bipyridinium) guests 1a and 1b
As shown in Scheme 1, 1,3-bis(4,4′-bipyridinium)propane 1a and 1,1-bis(4,4′-bipyridinium)methane 1b were conveniently synthesized by N-alkylation of 4,4′-bipyridine and then exchange of anions. Their structures were well characterized by nuclear magnetic resonance (NMR) spectroscopy and electrospray ionization mass (ESI-MS) spectrometry (Figs. S1–S4†).
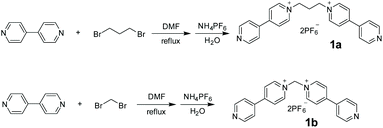 |
| Scheme 1 Synthesis of pyridinium-derived guests 1a and 1b. | |
The crystals of 1a were obtained by vapor diffusion of isopropyl ether into its acetone solution. The single-crystal X-ray analysis (Fig. 1) indicated that the two bipyridinium units of 1a are non-coplanar. Because the three propylene carbons on 1a are arranged in the staggered conformations and the C–C bond (Fig. 1a) and N–C bond (Fig. 1b) are basically parallel, the bite angle between the two pyridinium groups should be the same as the C–C–N angle (111.0°), which is a little smaller than that of 1b shown in its crystal structure (112.3°) reported by Peinador and Quintela (Fig. S29†).13b
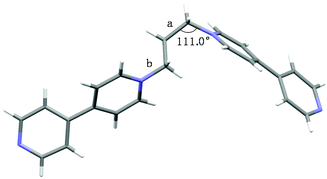 |
| Fig. 1 The crystal structure of 1a. The counterions have been omitted for clarity. Color code: gray = C; white = H; blue = N. | |
Investigation of the host–guest complexation of DB24C8 ⊃ 1a and DB30C10 ⊃ 1a
Since the host–guest complexation of 1a with crown ethers has never been reported, we firstly investigated their complexation in acetone. The 1H NMR spectra (Fig. 2) of its mixture solutions with equivalent DB24C8 or DB30C10 (dibenzo-30-crown-10) showed obvious changes on both hosts and 1a. Peaks of Ha, Hb, Hc, Hd and He on the guest 1a, the aromatic protons H1 and H2 on DB24C8, and the protons H1′, H2′, H3′ and H4′ on DB30C10 all shifted upfield, while H3, H4, H5, H5′ and H6′ moved downfield. Only one set of peaks were found in the 1H NMR spectra of the mixed solutions (Fig. 2b and d), indicating that both of the complexation of DB24C8 ⊃ 1a and DB30C10 ⊃ 1a are fast-exchange systems on the NMR timescale. The Job plots14 (Figs. S22 and S24†) based on the 1H NMR spectroscopic data demonstrated that complexes of 1a with both DB24C8 and DB30C10 have 1
:
1 stoichiometry in solutions.
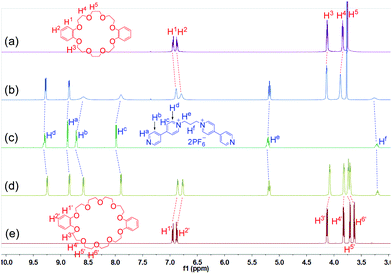 |
| Fig. 2 Partial 1H NMR spectra (500 MHz, acetone-d6, 25 °C) of 12.00 mM DB24C8 (a), 12.00 mM 1a + 12.00 mM DB24C8 (b), 12.00 mM 1a (c), 12.00 mM 1a + 12.00 mM DB30C10 (d), and 12.00 mM DB30C10 (e). | |
Furthermore, the 1
:
1 stoichiometry was confirmed by their ESI-MS spectra. One main peak was observed for [DB24C8 ⊃ 1a − PF6]+: m/z = 947.3587, and one minor peak was found for [[DB24C8]2 ⊃ 1a − PF6]+: m/z = 1395.5655 (Fig. S23†). Nonetheless, the latter is found in very small abundance, indicating that they mainly formed a 1
:
1 complex in solution. Two relevant peaks were found for the complex of DB30C10 ⊃ 1a with 1
:
1 stoichiometry, [DB30C10 ⊃ 1a − PF6]+: m/z = 1035.42387 and [DB30C10 ⊃ 1a − 2PF6]2+: m/z = 445.2301 (Fig. S25†). No peaks were observed for the complexes with other stoichiometries. The association constant (Ka) values of DB24C8 ⊃ 1a and DB30C10 ⊃ 1a were determined to be 2620 (±200) M−1 and 1490 (± 120) M−1, respectively, in CH3CN by using the UV-vis titration method (Figs. S27 and S28†). Both of them are higher than the previously reported association constant13b of DB24C8 ⊃ 1b, 915 ± 35 M−1, in CH3CN, probably because the longer propylene linker with higher flexibility is more space-matching and enables better dynamic adaption to be complexed by the crown ethers than the shorter methylene linker.
Preparation of the quadrilateral metallacycles 2a and 2b
As the flexible bis(4,4′-bipyridinium)propane 1a has a similar coordination angle to 1b, it was supposed that it could coordinate with a 90° platinum(II) acceptor to form discrete quadrilateral metallacycles such as 1b.13 An acetone-d6 solution of 1a or 1b was stirred with an equimolar 90° Pt(II) receptor (6) at room temperature, which gave rise to the formation of metal assemblies 2a and 2b, respectively (Scheme 2). The 1H and 31P{1H} NMR spectra of 2a and 2b, together with their 1H–1H COSY (correlation spectroscopy) NMR spectra and mass spectrometry, provided solid evidence for the formation of discrete metallacycles. Both the 31P{1H} NMR spectra of 2a and 2b displayed a singlet at −0.30 ppm and −0.23 ppm, respectively, accompanied by a set of symmetrical and uniformed 195Pt satellites, which was consistent with the Pt–N coordination environment (Fig. S6 and S9†). All peaks in their 1H NMR (Fig. S5 and S8†) and 1H–1H COSY NMR spectra (Fig. S7 and S10†) were also clearly assigned. Although the X-ray crystal structure shows a nearly 109° bite angle of 1a in the solid state (Fig. 1), other conformations with slightly different bite angles may exist in solution where the assembly was performed due to its relatively higher flexibility. In the 1H NMR spectrum of 2a, we observed tiny impure peaks that might belong to the isomeric self-assembly species resulting from the conformational isomerism of the propylene linker. In comparison, no visible impure peaks were found in the 1H NMR spectrum of 2b, which could be due to the less flexibility of ligand 1b. The ESI-MS measurements further supported the formation of metallacycles 2a and 2b. The ESI-MS spectrum of metallacycle 2a (Fig. S17†) showed two related peaks at m/z = 1224.2068 and 1228.1958, attributed to [M − 2OTf]2+ and [M − 2PF6]2+, respectively. For the metallacycle 2b (Fig. S18†), one peak at m/z = 1383.2289 was observed corresponding to [M + 2K]2+.
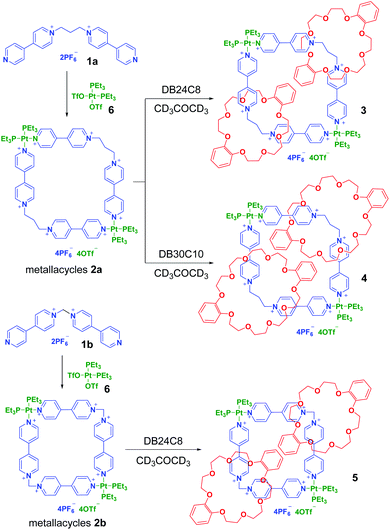 |
| Scheme 2 Synthesis of metallacycles 2a and 2b and [3]catenanes 3, 4, and 5. | |
Synthesis of [3]catenanes 3, 4 and 5
Because of the dynamic nature of the coordination interactions, the in situ addition of crown ethers into the prepared metallacycles 2 resulted in the generation of catenanes. [3]Catenanes 3 and 5 were fabricated by the addition of DB24C8 into the solutions of 2a or 2b (3.0 mM), respectively, at room temperature, while [3]catenane 4 was obtained by the addition of DB30C10 (18.0 mM) into a solution of 2a (3.0 mM). Directly mixing the precursors (1 + 6 + crown ether) in one pot also led to the same results (Fig. S30–35†). After the addition of crown ethers, all the solutions changed from colorless to yellow, which indicated that charge-transfer interactions existed between crown ethers and electron-poor guests.
The dynamic threading processes of metallacycles 2 (2a or 2b) and crown ether (DB24C8 or DB30C10) were observed real-time by 31P{1H} and 1H NMR spectroscopy. When DB24C8 was added into a 3.0 mM solution of 2a, a new set of peaks corresponding to [3]catenane 3 appeared in the 31P{1H} NMR spectra (Fig. 3). As the amount of DB24C8 increased, 2a gradually converted into 3. Finally, when 18.0 mM DB24C8 was added, the peak related to free metallacycle 2a almost completely disappeared (Fig. 3d). The same trend was also observed in the 1H NMR spectra (Fig. 4). When 6.0 mM DB24C8 was added into a 3.0 mM solution of 2a, the characteristic peaks indicated that the solution mainly contained three different species, the free DB24C8, the free metallacycle 2a and the threaded [3]catenane 3. After more DB24C8 was added, the peaks of 2a decreased gradually. Finally, [3]catenane 3 was formed as the main product (Fig. 4d). Like the host–guest complexation of DB24C8 ⊃ 1a, all aromatic hydrogen signals of the guest moiety 2a shifted upfield, mostly because of the shielding effect and the increase of electron density originated from the charge transfer and CH–O hydrogen bonds, after the formation of 3. The slight upfield shift of the phosphorus signal of 2a should also be attributed to the enrichment of the electron density of 2a after its complexation with the electron-rich crown ethers.
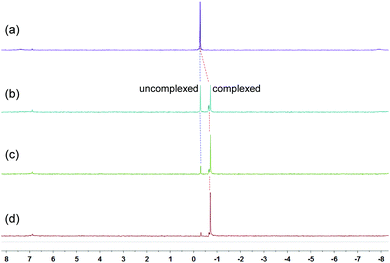 |
| Fig. 3
31P{1H} NMR (202 MHz, acetone-d6, 25 °C) spectra of metallacycle 2a (3.0 mM) (a), 2a (3.0 mM) + DB24C8 (6.0 mM) (b), 2a (3.0 mM) + DB24C8 (12.0 mM) (c), and 2a (3.0 mM) + DB24C8 (18.0 mM) (d). | |
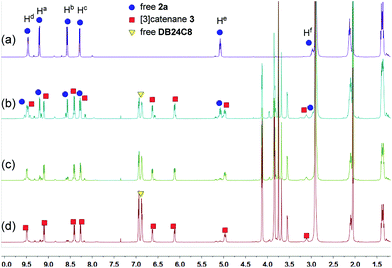 |
| Fig. 4
1H NMR (500 MHz, acetone-d6, 25 °C) spectra of metallacycle 2a (3.0 mM) (a), 2a (3.0 mM) + DB24C8 (6.0 mM) (b), 2a (3.0 mM) + DB24C8 (12.0 mM) (c), and 2a (3.0 mM) + DB24C8 (18.0 mM) (d). | |
These changes in the 31P{1H} and 1H NMR spectra all indicated the gradual formation of [3]catenane 3. Similar changes were also observed for 4 (Fig. 5 and 6) and 5 (Fig. 7 and 8). Since there was no great difference in the association constants of DB24C8 ⊃ 1a, DB30C10 ⊃ 1a and DB24C8 ⊃ 1b, the amounts of crown ethers for complete conversion from free metallacycles to the [3]catenanes 3, 4 and 5 were almost the same.
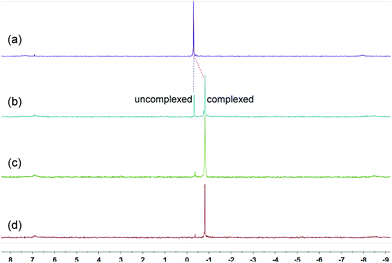 |
| Fig. 5
31P{1H} NMR (202 MHz, acetone-d6, 25 °C) spectra of metallacycle 2a (3.0 mM) (a), 2a (3.0 mM) + DB30C10 (6.0 mM) (b), 2a (3.0 mM) + DB30C10 (12.0 mM) (c), and 2a (3.0 mM) + DB30C10 (18.0 mM) (d). | |
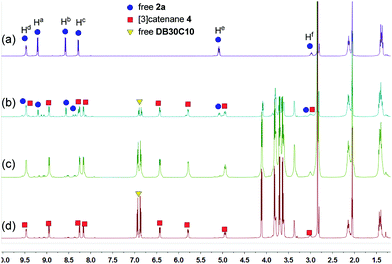 |
| Fig. 6
1H NMR (500 MHz, acetone-d6, 25 °C) spectra of metallacycle 2a (3.0 mM) (a), 2a (3.0 mM) + DB30C10 (6.0 mM) (b), 2a (3.0 mM) + DB30C10 (12.0 mM) (c), and 2a (3.0 mM) + DB30C10 (18.0 mM) (d). | |
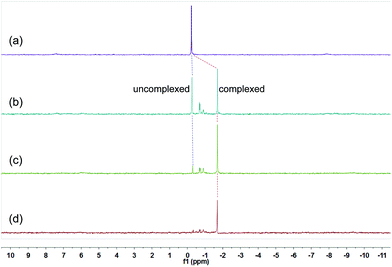 |
| Fig. 7
31P{1H} NMR (202 MHz, acetone-d6, 25 °C) spectra of metallacycle 2b (3.0 mM) (a), 2b (3.0 mM) + DB24C8 (6.0 mM) (b), 2b (3.0 mM) + DB24C8 (12.0 mM) (c), 2b (3.0 mM) + DB24C8 (18.0 mM) (d). | |
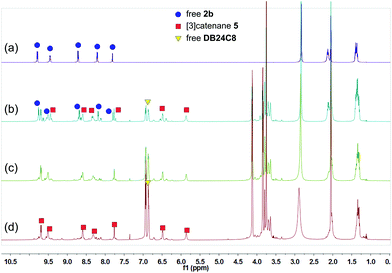 |
| Fig. 8
1H NMR (500 MHz, acetone-d6, 25 °C) spectra of metallacycle 2b (3.0 mM) (a), 2b (3.0 mM) + DB24C8 (6.0 mM) (b), 2b (3.0 mM) + DB24C8 (12.0 mM) (c), and 2b (3.0 mM) + DB24C8 (18.0 mM) (d). | |
ESI-MS studies further supported the formation of [3]catenanes. A few peaks belonging to the intact catenanes were found in their MS spectra, but some of them overlapped with their half-fragment peaks (Fig. S19–S21†). Therefore, we further exchanged the mixed counteranions of OTf− and PF6− to be unified PF6−. The detailed exchange procedures are provided in the ESI.†1H and 31P{1H} NMR spectra of the anion-exchanged metallacycles (7 and 8) and catenanes (9, 10 and 11) (Fig. S36–S45†) indicated that the exchange of anions did not affect their structures. In the MS spectra of the anion-exchanged [3]catenanes, the overlapped half-fragment peaks did not appear. Four related peaks were observed in the MS spectrum of anion-exchanged [3]catenane 9 at m/z = 1668.8662, 1064.3447, 1893.1202 and 1533.5529, attributed to [M − 2PF6]2+, [M − 3PF6]3+, [M + 2MeOH + H2O + 2K]2+ and [M − 4HPF6 + H + Na]2+, respectively (Fig. S46†). For [3]catenane 10, three peaks relevant to the intact [3]catenane self-assembly were found at m/z = 1757.0630, 1122.7149 and 1339.5517, corresponding to [M − 2PF6]2+, [M − 3PF6]3+ and [M − 8HPF6 + Na + NH4]2+, respectively (Fig. S47†). The ESI-MS spectrum of [3]catenane 11 (Fig. S48†) showed one peak at m/z = 1785.5858, corresponding to [M + 2H]2+.
Moreover, the formation of [3]catenanes was unambiguously confirmed by single-crystal X-ray analysis (Fig. 9). The X-ray-quality yellow crystals of [3]catenane 4 were obtained by slow diffusion of methanol into an acetone solution of 2a (3.0 mM) with excess DB30C10 (18.0 mM). The crystal structure of 4 revealed that the bite angle between the two pyridinium groups (112.3°) almost remains the same as that in the free ligand 2a (111.0°, Fig. 1) and the coordination angle of N–Pt–N reduced 6.8° (from the ideal Pt(II) square coordination angle of 90.0° to 83.2°) to meet the demands for the quadrilateral metallacycle with the guest 1a. Two DB30C10 molecules thread into the metallacycle 2a with an S-shaped conformation and symmetrically locate on the two diagonal corners of the metallacycle, that is, complex around the propylene groups. The two phenyl rings on the crown ethers are almost parallel to the bipyridine units on the guests inside and outside the metallacycle, and the distances are about 3.5 Å (g and h in Fig. 9), which is a favorable distance for π–π stacking interactions. At the same time, the phenyl rings on the two crown ethers inside the metallacycle are also parallel to each other. Additionally, the ether oxygen atoms of DB30C10 have strong hydrogen bond interactions (a–f in Fig. 9) with some of the hydrogen atoms on the propylene groups or on the pyridinium rings of 1a, which not only stabilize the conformations of the host–guest complex but also strengthen the rigidity of the propylene linker.
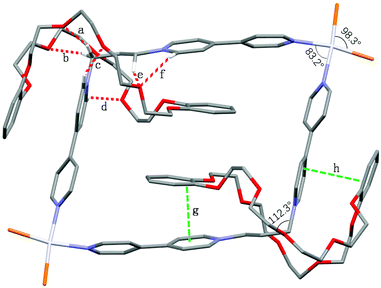 |
| Fig. 9 Single-crystal X-ray structure of [3]catenane 4. The ethyl groups on phosphines, counterions, and H atoms, except for the ones involved in hydrogen bonding, have been omitted for clarity. Hydrogen bond parameters: H⋯O distance (Å), C–H⋯O angle (deg); a, 2.314, 161.3; b, 2.396, 159.6; c, 2.400, 158.5; d, 2.403, 137.5; e, 2.549, 133.2; f, 2.541, 141.5. Color code: gray = C; red = O; white = H; blue = N; white = Pt; orange = P. | |
Conclusions
In summary, we synthesized a flexible bidentate ligand bis(4,4′-bipyridinium)propane 1a using bis(4,4′-bipyridinium)methane 1b as a control compound, which could complex with DB24C8 or DB30C10 with high association constants. By coordination-driven self-assembly of these two donors and a 90° platinum(II) receptor, two quadrilateral metallacycles were fabricated efficiently. Taking advantage of the dynamic coordination bonds, three [3]catenanes were obtained quantitatively by the in situ threading of 2 into the crown ethers. By comparing the crystal structures of the free ligand 1a and the coordinated one in [3]catenane 4, it was found that the bite angle between the two pyridinium groups almost remains the same after the formation of the [3]catenane. The bite angle between the two pyridinium groups either in the free ligand 2a or in the [3]catenane is slightly higher than 109°, while the bite angle of N–Pt–N decreased to 83.2° from the ideal Pt(II) square coordination angle of 90.0° to meet the demands for the formation of the quadrilateral metallacycle. It was believed that the wrapping of crown ethers turns the flexible propylene-linked bidentate ligands 1a rigid and the large bite angle of 1a makes the platinum(II) receptor 6 an adaptable building block in the [3]catenanes. In the future, we will focus on the investigation of ligands with controllable bite angles and their applications in transformable SCCs or molecular machines.
Experimental section
Materials and methods
Unless otherwise noted, materials were obtained from commercial suppliers and used without further purification. Solvents were either employed as purchased or dried according to procedures described in the literature. Column chromatography was performed over silica gel (200–300 mesh). NMR spectra were collected on a Bruker AVANCE DMX-500 spectrometer at room temperature. All NMR data are reported in ppm. 31P{1H} NMR chemical shifts are referenced to an external unlocked sample of 85% H3PO4 (δ 0.0), while 1H NMR and 13C{1H} NMR chemical shifts are reported relative to the residual peak of the deuterated solvents or internal standard tetramethylsilane (TMS). ESI-MS spectra were obtained on an Agilent 1290-6530 UPLC-Q-TOF spectrometer using electrospray ionization. Crystal data were collected on a Bruker D8 Venture (Billerica, Massachusetts, USA). The melting points were measured using a Melting Point M-565 apparatus.
Synthesis of 1,3-bis(4,4′-bipyridinium)propane 1a.
A solution of 4,4′-bipyridine (11.60 g, 74.30 mmol) and 1,3-dibromopropane (1.50 g, 7.43 mmol) in DMF (80 mL) was refluxed for 2 days. After cooling to room temperature, the yellow precipitate was filtered and washed with a small amount of DMF to afford a solid which was then re-dissolved in water (15 mL). An excess of NH4PF6 was added to the solution until no further precipitation was observed. The resulted residue was purified by flash column chromatography (methanol/2 M NH4Cl aqueous solution/nitromethane = 20
:
2
:
1) and then anion-exchanged by using NH4PF6 and dried to afford 1a as a white solid (1.10 g, 23%). M.P. 270–272 °C. 1H NMR (500 MHz, CD3CN) δ (ppm) 8.86 (dd, J = 4.5, 1.7 Hz, 4H), 8.80 (d, J = 6.9 Hz, 4H), 8.38 (d, J = 6.9 Hz, 4H), 7.80 (dd, J = 4.5, 1.7 Hz, 4H), 4.72–4.68 (m, 4H), 2.74–2.68 (m, 2H). 13C NMR (126 MHz, CD3CN) δ (ppm) 155.8, 152.3, 146.1, 142.0, 127.4, 122.8, 58.7, 32.7. ESI-TOF-MS (m/z): calcd for C23H22F6N4P [M − PF6]+: 499.1481, found: 499.1468.
Synthesis of 1,1-bis(4,4′-bipyridinium)methane 1b
13b.
A solution of 4,4′-bipyridine (3.37 g, 21.57 mmol) and dibromomethane (1.50 g, 8.63 mmol) in DMF (50 mL) was refluxed for 2 days. After cooling to room temperature, the yellow precipitate was filtered and washed with a small amount of DMF to afford a solid which was then re-dissolved in water (15 mL). An excess of NH4PF6 was added to the solution until no further precipitation was observed. The solid was filtered and washed with cold water to afford 1b as a white solid (2.40 g, 45%). 1H NMR (500 MHz, acetone-d6) δ (ppm) 9.84 (d, J = 7.0 Hz, 4H), 8.90–8.88 (m, 8H), 8.00 (dd, J = 4.5, 1.6 Hz, 4H), 7.90 (s, 2H).
Synthesis of metallacycle 2a.
The 90° Pt(II) precursor (6) (4.38 mg, 0.006 mmol) and 1a (3.87 mg, 0.006 mmol) were placed in a 2-dram vial, followed by the addition of acetone-d6 (0.6 mL). After 5 h of stirring at room temperature, the reaction solution was directly used for characterization. 1H NMR (500 MHz, acetone-d6,) (Fig. S5†): δ (ppm) 9.47 (d, J = 4.8 Hz, 8H), 9.21 (d, J = 7.0 Hz, 8H), 8.58 (d, J = 7.0 Hz, 8H), 8.29 (d, J = 6.6 Hz, 8H), 5.13–5.04 (m, 8H), 3.02–2.95 (m, 4H), 2.18–2.09 (m, 24H), 1.38 (dt, J = 17.5, 7.5 Hz, 36H). 31P{1H} NMR (202 MHz, acetone-d6) (Fig. S6†): δ (ppm) −0.30 (s, 195Pt satellites, JPt–P = 3087 Hz). ESI-TOF-MS (m/z): calcd for calcd for [M − 2PF6]2+: 1228.1934, found: 1228.1958; [M − 2OTf]2+: 1224.2094, found: 1224.2068.
Synthesis of metallacycle 2b.
The 90° Pt(II) precursor (6) (4.38 mg, 0.006 mmol) and 1b (3.69 mg, 0.006 mmol) were placed in a 2-dram vial, followed by the addition of acetone-d6 (0.6 mL). After 5 h of stirring at room temperature, the reaction solution was directly used for characterization. 1H NMR (500 MHz, acetone-d6) (Fig. S8†): δ (ppm) 9.79 (d, J = 6.9 Hz, 8H), 9.45 (d, J = 4.9 Hz, 8H), 8.72 (d, J = 6.9 Hz, 8H), 8.21 (d, J = 6.4 Hz, 8H), 7.81 (s, 4H), 2.20–2.09 (m, 24H), 1.38 (dt, J = 17.3, 7.4 Hz, 36H). 31P{1H} NMR (202 MHz, acetone-d6) (Fig. S9†): δ (ppm) −0.23 (s, 195Pt satellites, JPt–P = 3089 Hz). ESI-TOF-MS (m/z): calcd for [M + 2K]2+: 1383.1305, found: 1383.2289.
Synthesis of [3]catenane 3.
Various equivalents of DB24C8 (see Fig. 3 and 4) were added directly into a solution of metallacycle 2a in acetone-d6. The mixture was then stirred for 2 days at room temperature to generate [3]catenane 3. This initial reaction solution was directly used for the following characterization. 1H NMR (500 MHz, acetone-d6) (Fig. S11†): δ (ppm) 9.49 (d, J = 5.1 Hz, 8H), 9.10 (d, J = 6.7 Hz, 8H), 8.41 (d, J = 6.7 Hz, 8H), 8.26 (d, J = 6.3 Hz, 8H), 6.63 (dd, J = 5.8, 3.7 Hz, 8H), 6.12 (dd, J = 5.9, 3.4 Hz, 8H), 4.99–4.93 (m, 8H), 3.83 (s, 16H), 3.68 (s, 16H), 3.54 (d, J = 5.6 Hz, 16H), 3.10 (br, 4H), 2.15–2.08 (m, 24H), 1.40–1.34 (m, 36H). 31P{1H} NMR (202 MHz, acetone-d6) (Fig. S12†): δ (ppm) −0.71 (s, 195Pt satellites, JPt–P = 3101 Hz). ESI-TOF-MS (m/z): calcd for [M − 2PF6]2+: 1676.4032, found: 1676.4027; calcd for [M − 2OTf]2+: 1672.4153, found: 1672.4119.
Synthesis of [3]catenane 4.
Various equivalents of DB30C10 (see Fig. 5 and 6) were added directly into a solution of metallacycle 2a in acetone-d6. The solution was then stirred for 2 days at room temperature to generate [3]catenane 4. This initial reaction solution was directly used for the following characterization. 1H NMR (500 MHz, acetone-d6) (Fig. S13†): δ (ppm) 9.47 (d, J = 4.8 Hz, 8H), 8.95 (d, J = 6.8 Hz, 8H), 8.25 (d, J = 6.8 Hz, 8H), 8.17 (d, J = 6.6 Hz, 8H), 6.48–6.40 (m, 8H), 5.84–5.75 (m, 8H), 4.99–4.90 (m, 8H), 3.81–3.78 (m, 16H), 3.68 (dd, J = 5.2, 3.3 Hz, 16H), 3.61 (d, J = 4.6 Hz, 16H), 3.37 (d, J = 2.7 Hz, 16H), 3.04–2.98 (m, 4H), 2.18–2.10 (m, 24H), 1.42 (dt, J = 17.4, 7.5 Hz, 36H). 31P{1H} NMR (202 MHz, acetone-d6) (Fig. S14†): δ (ppm) −0.81 (s, 195Pt satellites, JPt–P = 3111 Hz). ESI-TOF-MS (m/z): calcd for [M − 2OTf]2+: 1760.4678, found: 1760.4725; calcd for [M − 2PF6]2+: 1764.4556, found: 1764.4629; calcd for [M − 3OTf]3+: 1123.9968, found: 1123.9954.
Synthesis of [3]catenane 5.
Various equivalents of DB24C8 (see Fig. 7 and 8) were added directly into a solution of metallacycle 2b in acetone-d6. The solution was then stirred for 2 days at room temperature to generate [3]catenane 5. This initial reaction solution was directly used for the following characterization. 1H NMR (500 MHz, acetone-d6) (Fig. S15†): δ (ppm) 9.69 (d, J = 6.9 Hz, 8H), 9.51 (s, 8H), 8.59 (d, J = 6.4 Hz, 8H), 8.32 (s, 8H), 7.76 (s, 4H), 6.50–6.46 (m, 8H), 5.86 (s, 8H), 3.80 (s, 16H), 3.69 (d, J = 5.0 Hz, 16H), 3.65 (s, 16H), 2.02–2.00 (m, 24H), 1.35–1.30 (m, 36H). 31P{1H} NMR (202 MHz, acetone-d6) (Fig. S16†): δ (ppm) −1.64 (s, 195Pt satellites, JPt–P = 3141 Hz). ESI-TOF-MS (m/z): calcd for [M − 2PF6]2+: 1648.3718, found: 1648.3750; calcd for [M − 2OTf]2+: 1644.3879, found: 1644.3840.
Author contributions
Investigation, Y. S., L. H., Q. M., T. H., and X. Y.; writing – original draft, Y. S. and Z. Z.; funding acquisition and project administration, S. L.; conceptualization, supervision, validation, and writing – review and editing, S. L. and P. S. All authors have read and agreed to the published version of the manuscript.
Conflicts of interest
There are no conflicts to declare.
Acknowledgements
This work was supported by the National Natural Science Foundation of China (21572042 and 21773052), the Program for Changjiang Scholars and Innovative Research Team in the Chinese University (IRT 1231), and the Science & Technology Innovation Program of Zhejiang Province (2018R52051). The authors thank Jiyong Liu (Department of Chemistry, Zhejiang University, Hangzhou 310027, P. R. China) for the single-crystal characterization of 1a and 4.
References
-
(a)
Molecular catenanes, rotaxanes and knots, ed. J.-P. Sauvage and C. Dietrich-Buchecker, Wiley-VCH, Weinheim, 1999 Search PubMed;
(b) R. S. Forgan, J.-P. Sauvage and J. F. Stoddart, Chemical topology: complex molecular knots, links, and entanglements, Chem. Rev., 2011, 111, 5434–5464 CrossRef CAS PubMed;
(c) J. E. Beves, B. A. Blight, C. J. Campbell, D. A. Leigh and R. T. McBurney, Strategies and tactics for the metal-directed synthesis of rotaxanes, knots, catenanes, and higher order links, Angew. Chem., Int. Ed., 2011, 50, 9260–9327 CrossRef CAS PubMed;
(d) M. Xue, Y. Yang, X. Chi, X. Yan and F. Huang, Development of pseudorotaxanes and rotaxanes: from synthesis to stimuli-responsive motions to applications, Chem. Rev., 2015, 115, 7398–7501 CrossRef CAS PubMed;
(e)
C. J. Bruns and J. F. Stoddart, The Nature of the Mechanical Bond: From Molecules to Machines, Wiley, New Jersey, 2017 Search PubMed;
(f) K. M. Bak, K. Porfyrakis, J. J. Davis and P. D. Beer, Exploiting the mechanical bond for molecular recognition and sensing of charged species, Mater. Chem. Front., 2020, 4, 1052–1073 RSC.
-
(a) E. R. Kay, D. A. Leigh and F. Zerbetto, Synthetic molecular motors and mechanical machines, Angew. Chem., Int. Ed., 2007, 46, 72–191 CrossRef CAS PubMed;
(b) Z. Niu and H. W. Gibson, Polycatenanes, Chem. Rev., 2009, 109, 6024–6046 CrossRef CAS PubMed;
(c) A. Harada, A. Hashidzume, H. Yamaguchi and Y. Takashima, Polymeric rotaxanes, Chem. Rev., 2009, 109, 5974–6023 CrossRef CAS;
(d) L. Fang, M. A. Olson, D. Benítez, E. Tkatchouk, W. A. Goddard III and J. F. Stoddart, Mechanically bonded macromolecules, Chem. Soc. Rev., 2010, 39, 17–29 RSC;
(e) M. Zhang, K. Zhu and F. Huang, Improved complexation of paraquat derivatives by the formation of crown ether-based cryptands, Chem. Commun., 2010, 46, 8131–8141 RSC;
(f) A. Coskun, M. Banaszak, R. D. Astumian, J. F. Stoddart and B. A. Grzybowski, Great expectations: can artificial molecular machines deliver on their promise?, Chem. Soc. Rev., 2012, 41, 19–30 RSC;
(g) M. Zhang, X. Yan, F. Huang, Z. Niu and H. W. Gibson, Stimuli-responsive host-guest systems based on the recognition of cryptands by organic guests, Acc. Chem. Res., 2014, 47, 1995–2005 CrossRef CAS;
(h) Z. Zhang, K. Sun, L. Jin, C. Xie and S. Li, Preparation of a mechanically interlocked polymer from a linear supramolecular polymer, Org. Chem. Front., 2020, 7, 1453–1462 RSC.
-
(a) D. A. Leigh, J. K. Y. Wong, F. Dehez and F. Zerbetto, Unidirectional rotation in a mechanically interlocked molecular rotor, Nature, 2003, 424, 174–179 CrossRef CAS PubMed;
(b) J.-P. Sauvage, Transition metal-complexed catenanes and rotaxanes as molecular machine prototypes, Chem. Commun., 2005, 1507–1510 RSC;
(c) O. Š. Miljanić and J. F. Stoddart, Dynamic donor–acceptor [2]catenanes, Proc. Natl. Acad. Sci. U. S. A., 2007, 104, 12966–12970 CrossRef PubMed;
(d) M. Liu, S. Li, M. Hu, F. Wang and F. Huang, Selectivity algorithm for the formation of two cryptand/paraquat catenanes, Org. Lett., 2010, 12, 760–763 CrossRef CAS PubMed;
(e) G. Gil-Ramírez, D. A. Leigh and A. J. Stephens, Catenanes: fifty years of molecular links, Angew. Chem., Int. Ed., 2015, 54, 6110–6150 CrossRef;
(f) H. Y. Au-Yeung, C.-C. Yee, A. W. H. Ng and K. Hu, Strategies to assemble catenanes with multiple interlocked macrocycles, Inorg. Chem., 2018, 57, 3475–3485 CrossRef CAS;
(g) N. H. Pérez and J. E. M. Lewis, Synthetic strategies towards mechanically interlocked oligomers and polymers, Org. Biomol. Chem., 2020, 18, 6757–6780 RSC;
(h) F.-Z. Yan, Y.-G. Shao, Z. Zhang, Y.-F. Shen, X.-C. Huang, P.-L. Zhang and S. Li, Synthesis of catenanes from a BMP32C10-based cryptand tuned by the linkage length of paraquat salts, Synthesis, 2021, 53, 338–343 CrossRef CAS;
(i) R. C. Knighton and P. D. Beer, Sodium cation-templated synthesis of an ion-pair binding heteroditopic [2]catenane, Org. Chem. Front., 2021, 8, 2468–2472 RSC.
-
(a) M. S. Vickers and P. D. Beer, Anion templated assembly of mechanically interlocked structures, Chem. Soc. Rev., 2007, 36, 211–225 RSC;
(b) J. D. Crowley, S. M. Goldup, A.-L. Lee, D. A. Leigh and R. T. McBurney, Active metal template synthesis of rotaxanes, catenanes and molecular shuttles, Chem. Soc. Rev., 2009, 38, 1530–1541 RSC;
(c) S. Li, K. Zhu, B. Zheng, X. Wen, N. Li and F. Huang, A bis(m-phenylene)-32-crown-10/paraquat [2]rotaxane, Eur. J. Org. Chem., 2009, 1053–1057 CrossRef CAS;
(d) J. E. M. Lewis, P. D. Beer, S. J. Loeb and S. M. Goldup, Metal ions in the synthesis of interlocked molecules and materials, Chem. Soc. Rev., 2017, 46, 2577–2591 RSC.
-
(a) J. M. Lehn, Dynamic combinatorial chemistry and virtual combinatorial libraries, Chem. – Eur. J., 1999, 5, 2455–2463 CrossRef CAS;
(b) S. J. Rowan, S. J. Cantrill, G. R. L. Cousins, J. K. M. Sanders and J. F. Stoddart, Dynamic covalent chemistry, Angew. Chem., Int. Ed., 2002, 41, 898–952 CrossRef;
(c) P. T. Corbett, J. Leclaire, L. Vial, K. R. West, J. L. Wietor, J. K. M. Sanders and S. Otto, Dynamic combinatorial chemistry, Chem. Rev., 2006, 106, 3652–3711 CrossRef CAS PubMed;
(d) J. M. Lehn, From supramolecular chemistry towards constitutional dynamic chemistry and adaptive chemistry, Chem. Soc. Rev., 2007, 36, 151–160 RSC;
(e) F. B. L. Cougnons and J. K. M. Sanders, Evolution of dynamic combinatorial chemistry, Acc. Chem. Res., 2012, 45, 2211–2221 CrossRef.
-
(a) S. Li, M. Liu, B. Zheng, K. Zhu, F. Wang, N. Li, X.-L. Zhao and F. Huang, Taco complex templated syntheses of a cryptand/paraquat [2]rotaxane and a [2]catenane by olefin metathesis, Org. Lett., 2009, 11, 3350–3353 CrossRef CAS PubMed;
(b) Y. W. Wu, S. T. Tung, C. C. Lai, Y. H. Liu, S. M. Peng and S. H. Chiu, Cyclic [2]catenane dimers, trimers, and tetramers, Angew. Chem., Int. Ed., 2015, 54, 11745–11749 CrossRef CAS PubMed;
(c) H. Li, H. C. Zhang, A. D. Lammer, M. Wang, X. P. Li, V. M. Lynch and J. L. Sessler, Quantitative self-assembly of a purely organic three-dimensional catenane in water, Nat. Chem., 2015, 7, 1003–1008 CrossRef CAS;
(d) S. P. Black, D. M. Wood, F. B. Schwarz, T. K. Ronson, J. J. Holstein, A. R. Stefankiewicz, C. A. Schalley, J. K. M. Sanders and J. R. Nitschke, Catenation and encapsulation induce distinct reconstitutions within a dynamic library of mixed ligand Zn4L6 cages, Chem. Sci., 2016, 7, 2614–2620 RSC.
-
(a) S. Leininger, B. Olenyuk and P. J. Stang, Self-assembly of discrete cyclic nanostructures mediated by transition metals, Chem. Rev., 2000, 100, 853–908 CrossRef CAS;
(b) M. Yoshizawa, J. K. Klosterman and M. Fujita, Functional molecular flasks: new properties and reactions within discrete, self-assembled hosts, Angew. Chem., Int. Ed., 2009, 48, 3418–3438 CrossRef CAS PubMed;
(c) R. Chakrabarty, P. S. Mukherjee and P. J. Stang, Supramolecular coordination: self-assembly of finite two- and three-dimensional ensembles, Chem. Rev., 2011, 111, 6810–6918 CrossRef CAS PubMed;
(d) T. R. Cook and P. J. Stang, Recent developments in the preparation and chemistry of metallacycles and metallacages via coordination, Chem. Rev., 2015, 115, 7001–7045 CrossRef CAS PubMed;
(e) A. J. McConnell, C. S. Wood, P. P. Neelakandan and J. R. Nitschke, Stimuli-responsive metal−ligand assemblies, Chem. Rev., 2015, 115, 7729–7793 CrossRef CAS PubMed;
(f) L.-J. Chen and H.-B. Yang, Construction of stimuli-responsive functional materials via hierarchical self-assembly involving coordination interactions, Acc. Chem. Res., 2018, 51, 2699–2710 CrossRef CAS PubMed;
(g) G.-Y. Wu, L.-J. Chen, L. Xu, X.-L. Zhao and H.-B. Yang, Construction of supramolecular hexagonal metallacycles via coordination-driven self-assembly: structure, properties and application, Coord. Chem. Rev., 2018, 369, 39–75 CrossRef CAS;
(h) H. Sepehrpour, W. Fu, Y. Sun and P. J. Stang, Biomedically relevant self-assembled metallacycles and metallacages, J. Am. Chem. Soc., 2019, 141, 14005–14020 CrossRef CAS;
(i) Y. Sun and P. J. Stang, Metallacycles, metallacages, and their aggregate/optical behavior, Aggregate, 2021, e94, DOI:10.1002/agt2.94;
(j) D. Zhang, T. K. Ronson, Y.-Q. Zou and J. R. Nitschke, Metal-organic cages for molecular separations, Nat. Rev. Chem., 2021, 5, 168–182 CrossRef CAS.
-
(a) K. Acharyya, S. Bhattacharyya, H. Sepehrpour, S. Chakraborty, S. Lu, B. Shi, X. Li, P. S. Mukherjee and P. J. Stang, Self-assembled fluorescent Pt(II) metallacycles as artificial light-harvesting systems, J. Am. Chem. Soc., 2019, 141, 14565–14569 CrossRef CAS PubMed;
(b) T. Hong, Z. Zhang, Y. Sun, J.-J. Tao, J.-D. Tang, C. Xie, M. Wang, F. Chen, S.-S. Xie, S. Li and P. J. Stang, Chiral metallacycles as catalysts for asymmetric conjugate addition of styrylboronic acids to α,β-enones, J. Am. Chem. Soc., 2020, 142, 10244–10249 CrossRef CAS PubMed;
(c) Z. Zhang, Z. Zhao, L. Wu, S. Lu, S. Ling, G. Li, L. Xu, L. Ma, Y. Hou, X. Wang, X. Li, G. He, K. Wang, B. Zou and M. Zhang, Emissive platinum(II) cages with reverse fluorescence resonance energy transfer for multiple sensing, J. Am. Chem. Soc., 2020, 142, 2592–2600 CrossRef CAS PubMed;
(d) H. Zhu, Q. Li, B. Shi, H. Xing, Y. Sun, S. Lu, L. Shangguan, X. Li, F. Huang and P. J. Stang, Formation of planar chiral platinum triangles via pillar[5]arene for circularly polarized luminescence, J. Am. Chem. Soc., 2020, 142, 17340–17345 CrossRef CAS PubMed;
(e) P. Howlader, S. Mondal, S. Ahmed and P. S. Mukherjee, Guest-induced enantioselective self-assembly of a Pd6 homochiral octahedral cage with a C3-symmetric pyridyl donor, J. Am. Chem. Soc., 2020, 142, 20968–20972 CrossRef CAS PubMed.
- H.-W. Schmidt and F. Würthner, A periodic system of supramolecular elements, Angew. Chem., 2020, 59, 8766–8775 CrossRef CAS PubMed.
-
(a) D. Whang, K.-M. Park, J. Heo, P. Ashton and K. Kim, Molecular necklace: quantitative self-assembly of a cyclic oligorotaxane from nine molecules, J. Am. Chem. Soc., 1998, 120, 4899–4900 CrossRef CAS;
(b) M. Fujita, N. Fujita, K. Ogura and K. Yamaguchi, Spontaneous assembly of ten components into two interlocked, identical coordination cages, Nature, 1999, 400, 52–55 CrossRef CAS;
(c) K.-M. Park, S.-Y. Kim, J. Heo, D. Whang, S. Sakamoto, K. Yamaguchi and K. Kim, Designed self-assembly of molecular necklaces, J. Am. Chem. Soc., 2001, 124, 2140–2147 CrossRef;
(d) Y. Liu, A. Bruneau, J. He and Z. Abliz, Palladium(II)-directed self-assembly of dynamic donor−acceptor [2]catenanes, Org. Lett., 2008, 10, 765–768 CrossRef CAS PubMed;
(e) C. Peinador, V. Blanco and J. M. Quintela, A new doubly interlocked [2]catenane, J. Am. Chem. Soc., 2009, 131, 920–921 CrossRef CAS PubMed;
(f) R. S. Forgan, D. C. Friedman, C. L. Stern, C. J. Bruns and J. F. Stoddart, Directed self-assembly of a ring-in-ring complex, Chem. Commun., 2010, 46, 5861–5863 RSC;
(g) V. Blanco, M. D. Garcia, C. Peinador and J. M. Quintela, Self-assembly of new fluorescent Pd(II) and Pt(II) 2,7-diazapyrenium-based metallocycles and study of their inclusion complexes and [3]catenane, Chem. Sci., 2011, 2, 2407–2411 RSC;
(h) Z.-T. Zhang, Y.-M. Zhang and Y. Lu, Controlled molecular self-assembly behaviors between cucurbituril and bispyridinium derivatives, J. Org. Chem., 2011, 76, 4682–4685 CrossRef CAS PubMed;
(i) S. Freye, J. Hey, A. T. Galan, D. Stalke, R. H. Irmer, M. John and G. H. Clever, Allosteric binding of halide anions by a new dimeric interpenetrated coordination cage, Angew. Chem., Int. Ed., 2012, 51, 2191–2194 CrossRef CAS;
(j) S. P. Black, A. R. Stefankiewicz, M. M. J. Smulders, D. Sattler, C. A. Schalley, J. R. Nitschke and J. K. M. Sanders, Generation of a dynamic system of three-dimensional tetrahedral polycatenanes, Angew. Chem., Int. Ed., 2013, 52, 5749–5752 CrossRef CAS PubMed;
(k) H. Lee, P. Elumalai, N. Singh, H. Kim, S. U. Lee and K.-W. Chi, Selective synthesis of ruthenium(II) metalla[2]catenane via solvent and guest-dependent self-assembly, J. Am. Chem. Soc., 2015, 137, 4674–4677 CrossRef CAS;
(l) T. Sawada, M. Yamagami, K. Ohara, K. Yamaguchi and M. Fujita, Peptide [4]catenane by folding and assembly, Angew. Chem., Int. Ed., 2016, 55, 4519–4522 CrossRef CAS PubMed;
(m) G.-Y. Wu, X. Shi, H. Phan, H. Qu, Y.-X. Hu, G.-Q. Yin, X.-L. Zhao, X. Li, L. Xu, Q. Yu and H.-B. Yang, Efficient self-assembly of heterometallic triangular necklace with strong antibacterial activity, Nat. Commun., 2020, 11, 3178–3188 CrossRef CAS PubMed;
(n) J. Singh, D. H. Kim, E.-H. Kim, H. Kim, R. Hadiputra, J. Jung and K.-W. Chi, The first quantitative synthesis of a closed three-link chain (613) using coordination and noncovalent interactions-driven self-assembly, J. Am. Chem. Soc., 2020, 142, 9327–9336 CrossRef CAS PubMed.
-
(a) S.-L. Huang, T. S. A. Hor and G.-X. Jin, Metallacyclic assembly of interlocked superstructures, Coord. Chem. Rev., 2017, 333, 1–26 CrossRef CAS;
(b) Y. Lu, H.-N. Zhang and G.-X. Jin, Molecular borromean rings based on half-sandwich organometallic rectangles, Acc. Chem. Res., 2018, 51, 2148–2158 CrossRef CAS PubMed;
(c) W.-X. Gao, H.-J. Feng, B.-B. Guo, Y. Lu and G.-X. Jin, Coordination-directed construction of molecular links, Chem. Rev., 2020, 120, 6288–6325 CrossRef CAS PubMed;
(d) L.-L. Dang, X. Gao, Y.-J. Lin and G.-X. Jin, Selective synthesis and structural transformation between a molecular ring-in-ring architecture and an abnormal trefoil knot, Chem. Sci., 2020, 11, 8013–8019 RSC;
(e) Z. Cui, Y. Lu, X. Gao, H.-J. Feng and G.-X. Jin, Stereoselective synthesis of a topologically chiral solomon link, J. Am. Chem. Soc., 2020, 142, 13667–13671 CrossRef CAS PubMed;
(f) Y. Ye, Z.-P. Lin, W.-L. Jin, S.-P. Wang, J. Wu and S. Li, Self-assembly of mechanically interlocked structures via metal-mediated coordination cooperating with host-guest recognition, Prog. Chem., 2015, 27, 763–774 CAS;
(g) T. Kim, N. Singh, J. Oh, E.-H. Kim, J. Jung, H. Kim and K.-W. Chi, Selective synthesis of molecular borromean rings: engineering of supramolecular topology via coordination-driven self-assembly, J. Am. Chem. Soc., 2016, 138, 8368–8371 CrossRef CAS PubMed;
(h) N. Singh, D. Kim, D. H. Kim, E.-H. Kim, H. Kim, M. S. Lah and K.-W. Chi, Selective synthesis of iridium(III)-derived molecular Borromean rings, [2]catenane and ring-in-ring macrocycles via coordination-driven self-assembly, Dalton Trans., 2017, 46, 571–577 RSC;
(i) D. H. Kim, N. Singh, J. Oh, E.-H. Kim, J. Jung, H. Kim and K.-W. Chi, Coordination-driven self-assembly of a molecular knot comprising sixteen crossings, Angew. Chem., Int. Ed., 2018, 57, 5669–5673 CrossRef CAS PubMed;
(j) J. Singh, H. Kim and K.-W. Chi, Non-covalent interaction-directed coordination-driven self-assembly of non-trivial supramolecular topologies, Chem. Rec., 2021, 21, 574–593 CrossRef CAS PubMed.
-
(a) S. Li, J. Huang, T. R. Cook, B. J. Pollock, H. Kim, K.-W. Chi and P. J. Stang, Formation of [3]catenanes from 10 precursors via multicomponent coordination-driven self-assembly of metallarectangles, J. Am. Chem. Soc., 2013, 135, 2084–2087 CrossRef CAS PubMed;
(b) S. Li, J. Huang, F. Zhou, T. R. Cook, X. Yan, Y. Ye, B. Zhu, B. Zheng and P. J. Stang, Self-assembly of triangular and hexagonal molecular necklaces, J. Am. Chem. Soc., 2014, 136, 5908–5911 CrossRef CAS PubMed;
(c) Y. Ye, S.-P. Wang, B. Zhu, T. R. Cook, J. Wu, S. Li and P. J. Stang, Self-assembly of [3]catenanes and a [4]molecular necklace based on a cryptand/paraquat recognition motif, Org. Lett., 2015, 17, 2804–2807 CrossRef CAS PubMed.
-
(a) V. Blanco, M. Chas, D. Abella, E. Pía, C. Platas-Iglesias, C. Peinador and J. M. Quintela, Self-assembly of 1: 2 inclusion complexes between a metallocycle host and dihydroxyaromatic guests: a redox controlled complexation process, Org. Lett., 2008, 10, 409–412 CrossRef CAS PubMed;
(b) V. Blanco, M. Chas, D. Abella, C. Peinador and J. M. Quintela, Molecular catenation via metal-directed self-assembly and π-donor/π-acceptor interactions: efficient one-pot synthesis, characterization, and crystal structures of [3]catenanes based on Pd or Pt dinuclear metallocycles, J. Am. Chem. Soc., 2007, 129, 13978–13986 CrossRef CAS PubMed.
- P. Job, Formation and Stability of Inorganic Complexes in Solution, Ann. Chim., 1928, 9, 113–203 CAS.
Footnote |
† Electronic supplementary information (ESI) available: NMR and mass spectra of the guests, metallacycles and catenanes, host–guest complexation investigation and X-ray analysis data of the guest 1a and catenane 4. CCDC 2079026 and 2079027. For ESI and crystallographic data in CIF or other electronic format see DOI: 10.1039/d1qo00910a |
|
This journal is © the Partner Organisations 2021 |