DOI:
10.1039/C7CS00399D
(Review Article)
Chem. Soc. Rev., 2018,
47, 4198-4232
Carbon-based hybrid nanogels: a synergistic nanoplatform for combined biosensing, bioimaging, and responsive drug delivery
Received
30th December 2017
First published on 18th April 2018
Abstract
Nanosized crosslinked polymer networks, named as nanogels, are playing an increasingly important role in a diverse range of applications by virtue of their porous structures, large surface area, good biocompatibility and responsiveness to internal and/or external chemico-physical stimuli. Recently, a variety of carbon nanomaterials, such as carbon quantum dots, graphene/graphene oxide nanosheets, fullerenes, carbon nanotubes, and nanodiamonds, have been embedded into responsive polymer nanogels, in order to integrate the unique electro-optical properties of carbon nanomaterials with the merits of nanogels into a single hybrid nanogel system for improvement of their applications in nanomedicine. A vast number of studies have been pursued to explore the applications of carbon-based hybrid nanogels in biomedical areas for biosensing, bioimaging, and smart drug carriers with combinatorial therapies and/or theranostic ability. New synthetic methods and structures have been developed to prepare carbon-based hybrid nanogels with versatile properties and functions. In this review, we summarize the latest developments and applications and address the future perspectives of these carbon-based hybrid nanogels in the biomedical field.
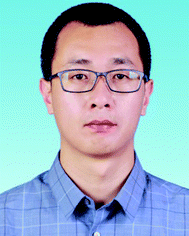
Hui Wang
| Hui Wang received a BS in chemistry (2006) from Anhui University and a PhD in inorganic chemistry (2011) from the University of Science and Technology of China (USTC), P. R. China, respectively. Since then, he has been a postdoctoral research associate in the Department of Chemistry at the College of Staten Island, The City University of New York and Department of Materials Science & Engineering, University of Washington, respectively. He is currently a professor of the High Magnetic Field Laboratory, Hefei Institutes of Physical Science, Chinese Academy of Sciences. His current research interests focus on the synthesis of carbon-based functional nanomaterials and exploring their applications in biomedicine including responsive drug carriers and cancer diagnosis and therapy. |
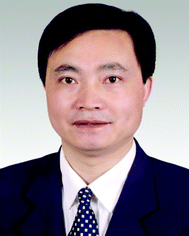
Qianwang Chen
| Professor Qianwang Chen received his PhD from the University of Science and Technology of China (USTC) in 1995 and was appointed to the faculty of USTC. He worked as a postdoc in the Institute of Hydrothermal Chemistry, Kochi, Japan, Alexander von Humboldt Research Fellow in the Solar Energy Institute of Hannover, Germany and as a visiting researcher in the Hong Kong Polytechnic University. He was appointed as a professor in 2000 at the USTC and was awarded the Cheung Kong Professorship in 2002 by the Ministry of Education of China. His research interests include the synthesis and application of nanoscale materials. He has co-authored over 290 publications in the research field. |
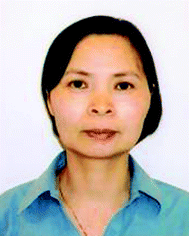
Shuiqin Zhou
| Shuiqin Zhou received a BS (1988) and a MS (1991) from Xiamen University, China, and a PhD from The Chinese University of Hong Kong in 1996. During 1996–2000 she worked at the State University of New York at Stony Brook as a postdoctoral research associate. She then worked at Union Carbide/The Dow Chemical Company as a senior chemist until 2002 when she moved to The City University of New York at the College of Staten Island as an associate professor. She is currently a professor of chemistry working in the field of micro/nanogels, supramolecular assembly, nanomaterials, and biomaterials. She has 115 journal publications and several book chapters. |
1. Introduction
Nanogels are three-dimensional (3D) hydrogel particles in the nanoscale size range (typically from tens to hundreds of nanometers) that can be fabricated by either physical or chemical cross-linking of polymers under controlled conditions.1–3 Physically crosslinked hydrogels have transient junctions between polymer chains that arise from either polymer chain entanglements and/or secondary forces such as ionic interactions, hydrogen bonds, or hydrophobic interactions. Chemically crosslinked hydrogels have permanent junctions between the polymer chains via covalent bonding linkages. While physically crosslinked hydrogels have the advantages of forming gels with no need of adding crosslinking agents or doing chemical modification, it is generally difficult to control variables such as gelation time, network pore size, mechanical strength, and chemical functionalization. In contrast, chemically crosslinked gels have a very stable network structure with a relatively high mechanical strength owing to the covalent bonding junctions and controllable pore size depending on the crosslinking degree, nature of crosslinking agents, and solvent quality for the polymer network chains. Chemically crosslinked gels can be synthesized by radical polymerization of small molecular vinyl monomers or polymers functionalized with polymerizable groups (macromonomers) in the presence of a crosslinking agent or by covalent linkages between polymer chains having reactive pendant functional groups such as –OH, –COOH, and –NH2. However, the residual monomers, initiators, and crosslinking agents have to be extracted from the gel networks before their application because of their toxicity. The stable chemical or physical bonds and high water content in the nanogels prevent the nanogels from dissolving but give rise to fluid-like transport properties for the molecules significantly smaller than the mesh size (2–25 nm on average in typical swollen nanogels,4 depending on the crosslinking degree and nature of the crosslinking agent) of the polymer networks.5,6 The unique physical properties resulting from this unusual state of matter make nanogels ideal candidates as eco-friendly and biocompatible nanocarriers for drugs and biomolecules widely used in disease diagnosis, sensing, and therapy, with the added benefit of high patient compliance and safety.7–10 Nanogels are considered advantageous over other drug delivery systems for a number of reasons. They are inert in the blood stream and internal aqueous environment and do not induce immunological responses in the body, because nanogels have soft structural and compositional resemblances to the extracellular matrix, suitable particle size, amenability to surface functionalization for minimal protein binding.11 They can avoid the rapid renal exclusion and clearance by phagocytic cells and the uptake by the reticuloendothelial system (RES) because of their suitable particle size typically in the range of 20 to 200 nm, leading to a prolonged blood circulation time.12 They provide possibilities for both passive and active site targeting to dose drugs with reduced side effects.13,14 Specifically, the physico-chemical properties including size, water content, refractive index, permeability, and hydrophilicity–hydrophobicity of nanogels can be varied in response to biomedical parameter changes such as pH, temperature, and level of biomolecules.15–17 The stimuli-responsive behavior of nanogels enables the nanogel-based drug delivery systems to become an active participant, rather than a passive carrier, in the optimization of disease therapy.18
In the past decade, a lot of effort has been made to integrate the stimuli-responsive nanogels with imaging contrast agents to combine the biosensing and/or bioimaging ability with the controlled drug delivery function.19–21 Such responsive hybrid nanogels have two key attributes. One is the endogenous and exogenous probing ability that enables the continuous monitoring of the biochemical and biophysical parameters over time and space to understand the intricate biological processes and develop novel diagnoses.22–28 Another is to realize simultaneous bioimaging and responsive drug delivery, which has great advantages over traditional chemotherapy since the imaging function can help to precisely identify the location and size of the tumor site and monitor the treatment progress of chemotherapy.29–32 It is expected that the combination of imaging contrast agents with the responsive nanogel carriers into a single system can address the disease from multiple angles, so that the weaknesses of one therapeutic or diagnostic agent can be remedied with the strengths of another and vice versa. Until now, various kinds of imaging contrast agents with specific size and shape have been used to synthesize multifunctional hybrid nanogels, including paramagnetic ions, organic dyes, semiconducting quantum dots (QDs), polymer dots, noble metal nanoparticles (NPs), metal oxides and rare earth metal (REM) NPs.33–45 Several recent reviews have summarized the preparation and theranostic applications of these multifunctional hybrid nanogels.19,20,42,46–49 While these hybrid nanogels have demonstrated excellent medical imaging functions in magnetic resonance imaging (MRI),50 fluorescence optical imaging (FOI),51 X-ray computed tomography (CT) imaging,52 positron-emission tomography (PET) imaging,53 and ultrasound (UA) imaging,54 several disadvantages are associated with these contrast agents embedded in the hybrid nanogels. For example, the toxicity of potentially leaked heavy metal species (e.g., Gd, Cd and Pb), insufficient brightness, passivated surface and stochastic blinking of metals or QDs used in the hybrid nanogels hinder their use for in vivo sensing and therapeutic applications.55,56 Organic dyes and polymer dots in hybrid nanogels often show poor photostability and potential toxicity when used as biosensing and bioimaging agents.57–59 The noble metal-based sensors often possess potential toxicity, low sensitivity, and unstable optical properties in the local chemical environment/surrounding medium, which limits their signal output as a sensor.60,61
On the other hand, carbon nanomaterials (fullerene, graphene, carbon nanotubes (CNTs), carbon NPs, carbon dots (CDs) and nanodiamond (ND)) are increasingly being used in various fields including energy, catalysis and water treatment owing to their unique physicochemical properties.62–68 Because of their excellent biocompatibility, large specific surface area, non-specific binding sites, easy surface modification and potential biodegradation, carbon nanomaterials have been explored as a novel tool for biomedical applications.69–72 For example, carbon nanomaterials with photoluminescent (PL) properties or functionalized with an optical imaging agent have been widely exploited for optical sensing of pH, temperature, glucose, ions, and other species.73–78 Carbon-based drug delivery systems often demonstrate high loading capacity for chemotherapeutics through π-stacking or hydrophobic interactions between the drug molecules and carbonaceous framework.79–82 In addition, owing to the aromatic and π-conjugated chemical bonds in the carbonaceous framework, carbon-based drug carriers can effectively absorb and convert near infrared (NIR) light to heat, and thus enable the NIR-responsive drug release for combined chemo-photothermal therapy.83–86 Furthermore, carbon nanostructures with electron donors and electron acceptors could be potential candidates for photodynamic therapy in which the light energy is converted to produce reactive oxygen species (ROS) to kill nearby cancer cells.87–90 With all these unique properties of carbon nanomaterials, the combination of carbon nano-objects with different polymer matrices has been widely pursued to develop multifunctional nanocomposites for a broad range of applications.91–105 A recent review has overviewed the synthesis, properties, and applications of various gel-nanocomposites assembled from nanocarbons with polymeric organogels and hydrogels.106 The soft-nanocomposites resultant from the incorporation of fullerenes, graphenes, and CNTs into gel matrices have been applied in solar cell devices, oil/dye absorption and recovery, NIR induced photothermal and programmable devices, NIR-driven drug release, improved catalytic activity and antimicrobial activity, highly conductive films, and mechanically high strength materials. In contrast to these carbon-based bulky gel nanocomposites, there is a lack of such a comprehensive overview for the small sized carbon-based hybrid nanogel materials, although a very brief section summarizing a few early developed carbon-based hybrid nanogel systems was included in a review on responsive nanogel composites.16 It is expected that the incorporation of carbon nanomaterials into the responsive polymer nanogels will combine the excellent properties and functions from each building block for many useful applications. Indeed, the development of carbon-based hybrid nanogels for biomedical applications has emerged as a rapid developing field in the past five years. The research indicates that the carbon-based hybrid nanogels could not only maintain the individual properties of each constituent, but also display cooperative properties. For example, the carbon-based hybrid nanogels as drug carriers can display (1) good biocompatibility because both carbon nano-objects and many nanogels formed by natural polymers (e.g. collagen, gelatin, polysaccharides etc.) or synthetic polymers (e.g., polyesters, polyethylene glycol etc.) have low toxicity and good biocompatibility,69,70,107 (2) stimulus-triggered drug release, (3) synergistic therapy, and (4) efficient imaging diagnosis ability, which is desirable to develop a new generation of drug delivery systems with improved delivery behavior and on-time monitoring ability of therapeutic efficacy.15,108,109 As schematically depicted in Fig. 1, with rational design of the compositions and properties of each building block, the carbon-based hybrid nanogels allow the integration of multiple important functions into a single nanocarrier system for biomedical applications such as biosensing, bioimaging and responsive drug delivery to improve treatment outcomes in a synergistic manner.
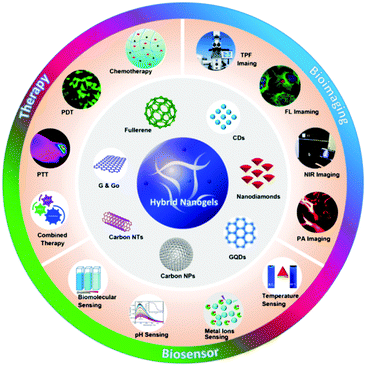 |
| Fig. 1 Carbon-based hybrid nanogels and their applications in nanomedicine including biosensors, bioimaging and therapy. | |
In this review, we aim to provide a comprehensive view on the synthesis of carbon-based hybrid nanogels functionalized with different carbon nanostructures and their applications for biosensing, bioimaging, responsive drug release and synergistic therapy. The general carbon–polymer nanocomposite particles with no crosslinking of polymer chains or carbon nano-objects embedded in a bulky polymer gel are not in the scope of this review. The focus on the carbon-based hybrid nanogels with carbon nano-objects embedded in the small polymer nanogels is important as these nanostructures bridge the gap between carbon nanoscience and the soft gel NPs and have attracted growing interests in the developments toward emerging applications for in situ diagnosis and therapy from low-toxic carbon and polymer nanogels. It is believed that this review will be of great interest to investigators and practitioners in materials science, biochemistry, biomedical engineering, nanotechnology, and nanomedicine.
2. Classification of responsive carbon-based hybrid nanogels
A key attribute of multifunctional hybrid nanogels as a drug delivery system is their ability to regulate the drug release under specific environments or stimuli, which can significantly improve the therapeutic efficacy at pathological sites but reduce the systemic side effects. Both endogenous and exogenous activations can regulate the drug release from the preloaded hybrid nanogels. The endogenous activation can be realized by the variation of specific physiochemical characteristics of the pathological microenvironment. For example, many pathological processes in tumor tissue and intracellular endosomes/lysosomes decrease the local pH by 1–2.5 pH units or increase the local temperature by 1–5 °C in comparison with that of blood and normal tissues.110–112 Some specific enzymes can be overexpressed on pathological sites that can degrade the hybrid nanogels to trigger the drug release.113–115 On the other hand, exogenous activation that can remotely control the drug release at a desired site and time using physical stimuli such as NIR light and magnetic field is considered crucial to boost the drug efficacy in cancer treatment while minimizing side effects.116–118 For carbon-based hybrid nanogels, the type of chemical/biochemical and physical stimuli that can be applied to trigger the release of preloaded drugs depends on the compositions of the polymer nanogels and carbon nanostructures. In this section, we classify the carbon-based hybrid nanogels in terms of their responsiveness to different types of external stimuli based on the chemical nature of both the polymer nanogels and carbon nano-objects.
2.1. pH-responsive carbon-based hybrid nanogels
The pH sensitivity of the carbon-based hybrid nanogels is generally originated from the pH-sensitive polymer network chains bearing acid or basic groups. The pKa values of these acid/base groups on the polymer network chains determine the sensitive pH-range of the resultant hybrid nanogels. When the pH is changed to cause the dissociation of these acid/base groups, the nanogel network chains become charged, which can build up a Donnan potential to swell the gel network.119 In such a case, the pH change can induce a swelling/de-swelling transition of the carbon-based hybrid nanogels. Many pH-sensitive polymers have been used to synthesize the carbon-based hybrid nanogels. Typically, monomers containing carboxylic acid and amine/amino groups are used to synthesize the polymeric hybrid nanogels. For example, acrylic acid (AA) with a –COOH group was copolymerized with vinylimidazole (VIM) in the presence of functionalized CNTs to form a pH-responsive CNT-based hybrid nanogel.120 2-(Diethylamino)ethyl methacrylate (DEA) with an amino group was used to synthesize pH-responsive pDEA–fullerene hybrid nanogels.121 A newly developed amino acid-derived zwitterionic monomer of ornithine methacrylamide (OrnAA) with both carboxylic and amine groups has been used to synthesize a pH-sensitive p(CD-OrnAA) hybrid nanogel.122 For natural polymers, the pH-sensitive polysaccharides are the most commonly used to prepare pH-responsive carbon-based hybrid nanogels. For example, chitosan containing amine groups has been used to synthesize pH-sensitive chitosan–CD hybrid nanogels.123 Alginate containing –COOH groups has been used to prepare pH-sensitive CD–alginate hybrid microgels,124,125 CNT–alginate hybrid microgels,126 and graphene oxide (GO)–alginate hybrid nanogels.127 Hyaluronic acid (HA) with –COOH groups has been used to crosslink with functionalized CDs to prepare pH-sensitive HA–graphene and HA–CDs hybrid nanogels.128 In some cases, the pH-sensitivity of the carbon-based hybrid nanogels can be also induced by the pH-sensitive surface functional groups on the carbon nano-objects like CDs or GO. For example, the CDs synthesized via a hydrothermal synthesis involving Agaricus bisporus as a carbon source and ethylenediamine as a nitrogen source possess both pH-sensitive –COOH and –NH2 groups on their surface. These CDs display pH-sensitive emission intensity at 550 nm when excited using 500 nm light. When these pH-sensitive CDs were incorporated into pH-insensitive poly(ethylene glycol) (PEG) microgels, the resultant CDs–PEG hybrid microgels exhibited excellent pH-sensitivity.129
2.2. Glucose-responsive carbon-based hybrid nanogels
Glucose-responsive carbon-based hybrid nanogels are designed from the incorporation of carbon nanomaterials into glucose-responsive nanogels. Typically, the glucose-responsiveness of the carbon-based hybrid nanogels is originated from the glucose-responsive nanogels. Two strategies have been applied to prepare glucose-sensitive nanogels. One is to encapsulate the enzyme of glucose oxidase into polymer nanogels because the glucose oxidase can oxidize glucose into gluconic acid and produce hydrogen peroxide.130–132 Another is to introduce boronic acid moieties onto the polymer nanogel network chains.133,134 The complexation of boronic acids to sugars or other compounds containing cis-diols has been used for glucose-sensing or glucose-responsive drug delivery for decades.135 Our group and Ravaine's group pioneered the synthesis and study of glucose-responsive nanogels almost at the same time via coupling phenyl boronic acid (PBA) groups onto the polymer nanogels.136,137 The PBA groups on the nanogel network chains are in equilibrium between the undissociated (trigonal, uncharged) and the dissociated (tetrahedral, charged) forms in aqueous solution. Both forms react reversibly with 1,2-cis-diols such as glucose. The complexation of the uncharged form with glucose is unstable because it is highly susceptible to hydrolysis, but the binding with glucose causes the thermodynamically more favorable charged form. As a result, the binding of glucose to the PBA groups on the nanogel network chains increases the degree of ionization and builds up a Donnan potential for the gel to swell. Compared to the enzyme-embedded polymer nanogels, the PBA-based polymer nanogels are highly stable and can bind the glucose reversibly for continuous glucose-sensing, while the enzymes are very sensitive to the environment and susceptible to denaturation. So far, several types of carbon nano-objects have been used to prepare glucose-responsive carbon-based hybrid nanogels. For example, GO nanosheets functionalized with alkenyl groups have been copolymerized and crosslinked with the 4-vinylphenylboronic acid (VPBA) monomers, producing the glucose-responsive graphene–P(4-VPBA) hybrid nanogels.138 Our group has recently immobilized fluorescent CDs into PBA-containing copolymer nanogels by rational design of the complexation interactions between the surface functional groups of CDs and the monomers, resulting in a type of glucose-responsive CDs–polymer hybrid nanogel.139 Kim's group has deposited glucose-responsive boronic acid-conjugated HA–cholesterol nanogels onto multiwall CNTs, resulting in a CNT–polymer nanogel hybrid for glucose sensing.140
2.3. Thermo-responsive carbon-based hybrid nanogels
The thermo-responsive properties of carbon-based hybrid nanogels are typically produced by the thermos-sensitive polymer nanogels in which the carbon nano-objects are hosted. Thermo-sensitive polymers exhibit a lower critical solution temperature (LCST) in water in a commonly detectable range. The polymer chain conformation undergoes a “coil–globule” transition from an expanded coil conformation in a good solvent (hydrated polymer chains) at temperatures below the LCST to a condensed globule state in a poor solvent (dehydrated polymer chains) when the temperature is above the LCST. When these polymer chains are crosslinked, the resultant nanogel undergoes a volume change around the volume phase transition temperature (VPTT). The VPTT of polymer nanogels can be tuned by changing the hydrophilic and hydrophobic moieties on the polymer network chains.141 Numerous thermos-sensitive polymers, such as poly(2-(2-methoxyethoxy)ethyl methacrylate) (PDEGMA),142 poly(N-vinyl caprolactam) (PNVCL),143 and poly(N-isopropylacrylamide) (PNIPAM),144 can be synthesized into nanogels based on a simple one-step precipitation polymerization in the water phase. Briefly, the monomers, crosslinkers, and initiator are completely soluble in water, but upon initiated polymerization, the formed polymers become insoluble in water at the reaction temperatures above the LCST of these thermo-sensitive polymers. These initially formed polymers precipitate out from the water phase and form nucleus seeds that can be stabilized by surfactant molecules. The polymerization proceeds by absorption of the remaining monomers/crosslinkers/initiators into the initially formed seed particles until the polymerization reaction is completed. When the temperature is cooled to temperatures below the LCST, water becomes a good solvent again for these polymers, thus the crosslinked polymer nanoparticles absorb water and swell to form nanogels. However, the most widely studied thermos-sensitive nanogels are PNIPAM-based nanogels with VPTT (∼34 °C) close to body temperature in water.145–148 In recent years, thermo-sensitive carbon-based hybrid nanogels were synthesized by incorporating carbon nanomaterials into PNIPAM nanogels to combine the merits of nanocarbons with the thermos-sensitivity of PNIPAM. For example, vinyl group-modified CDs were copolymerized into the PNIPAM nanogels via emulsion polymerization to form a thermo-sensitive CDs–PNIPAM hybrid nanogel.95 Different amounts of reduced GO (rGO) nanosheets have been loaded into PNIPAM nanogels to produce the thermo-sensitive GO–PNIPAM hybrid nanogels.149
For in vivo biomedical applications, there are some concerns regarding the potential cytotoxicity of the thermos-sensitive PNIPAM or PNVCL polymers, although the cytotoxicity of these polymers is much lower than that of their corresponding monomers of NIPAM and NVCL.143 It is desirable to use biocompatible thermos-responsive polymer nanogels to host carbon nanomaterials for in vivo applications. It is known that a PEG polymer has excellent biocompatibility and has been widely applied in the pharmaceutical industry with approval by the Food and Drug Administration (FDA).150 The PEGylation (the process of attaching PEG chains) on nanocarriers can sterically repel blood opsonin molecules and thus drastically increase the bioavailability of the nanocarriers with in vivo longevity and enhanced permeability and retention effect.15,151 About ten years ago, a new thermo-sensitive polymer called nonlinear PEG, composed of short oligo(ethylene glycol) (OEG) side chains grafted on the carbon backbone chain, was found to exhibit an interesting phase behavior in water with a tunable LCST determined by the length of the OEG side chains.152–155 Ever since, a variety of nonlinear PEG-based thermo-sensitive polymer nanogels have been investigated because of their biocompatibility and tunable VPTT.156–161 We previously incorporated Ag–Au bimetallic NPs and ZnO QDs into nonlinear PEG based nanogels to construct thermo-responsive hybrid nanogels for multifunctional drug carriers.23,36,37 Recently, we have incorporated CDs into nonlinear PEG-based nanogels to develop a biocompatible thermos-responsive carbon-based hybrid nanogel.162
2.4. NIR light- and electro-responsive carbon-based hybrid nanogels
The light- or electro-responsiveness of carbon-based hybrid nanogels normally originates from the light-absorbing properties and electric conductivity of carbon nanomaterials. Light-responsive carbon-based hybrid nanogels use light as an external stimulus to control the release of the preloaded active molecules, which is a very attractive activation strategy because a light source can be externally applied with high spatial and temporal precision.163 Among all light sources, NIR light in the wavelength range of 700–1000 nm is considered to be more favored than visible light due to its simple operation, low energy absorption, maximum penetration, and minimum side effects for human tissue and organs.164,165 In general, the nanocarbons embedded in the polymer nanogels can translate the absorbed photons into heat, which can enhance the local temperature in the hybrid nanogels. Numerous carbon-based hybrid nanogels have been developed with incorporation of CDs, GO nanosheets, and CNTs into polymer nanogels for photothermal agents and multifunctional drug carriers.149,166–168 On the other hand, the incorporation of electro-conducting multiwall CNTs into a non-responsive gelatin microgel can produce an electro-responsive drug delivery system.169
2.5. Multi-responsive carbon-based hybrid nanogels
The above described four categories of hybrid nanogels were mostly produced by incorporation of carbon-only nanomaterials into non-responsive or single-responsive polymer nanogels, which usually can only display responsiveness to a single stimulus or dual stimuli at most with each from nanocarbons and nanogels, respectively. In order to combine multiple stimuli-responsive activation strategies or functions into a single carbon-based hybrid nanogel drug delivery system, two types of combinations have been currently applied to develop multi-responsive carbon-based hybrid nanogels.
One is the incorporation of carbon nano-objects into dual-responsive polymer nanogels. The dual-responsive polymer nanogels can be synthesized by the copolymerization of different types of monomers, which can display responsiveness to different stimuli. For example, temperature- and pH-dual responsive poly(N-isopropylacrylamide-co-acrylic acid) [P(NIPAM–AA)] can be synthesized from the copolymerization of NIPAM and AA monomers. So far, GO nanosheets and CNTs have been respectively incorporated into the P(NIPAM-co-AA) nanogels, which resulted in pH-, thermo-, and NIR light-triple responsive carbon-based hybrid nanogels.170–172 Dual-responsive polymer nanogels can also be obtained through the synthesis of multicomponent polymer networks such as interpenetrated polymer networks (IPNs) in the nanogels. IPNs are defined by the International Union of Pure and Applied Chemistry (IUPAC) as ‘‘two or more networks that are at least partially interlaced on a molecular scale but not covalently bonded to each other and cannot be separated unless chemical bonds are broken’’.173 IPNs can be classified into full IPNs with the interpenetrated polymer chemically crosslinked inside the first set of polymer networks174 or semi-IPNs (sIPNs) with linear polymer chains embedded within the first set of polymer networks.48 Several dual-responsive IPN or sIPN structured polymer nanogels have been prepared for smart drug delivery systems,175 including thermo and pH dual responsive P(NIPAM–AA) IPNs,176 thermo and pH dual responsive PNIPAM–chitosan semi-IPNs,177 as well as pH and glutathione responsive disulfide crosslinked dextran–polyacrylic acid (PAA) IPNs.178 Recently, chitosan-coated CNTs have been embedded into the thermos-sensitive PNIPAM nanogel network, resulting in the incorporation of CNTs in the pH- and thermo-dual responsive chitosan–PNIPAM semi-IPNs. Such formed chitosan/PNIPAM@CNT hybrid nanogels exhibit triple responsiveness to the change of pH, temperature, and NIR irradiation.179 Our group has previously developed a biocompatible pH and temperature dual responsive semi-IPN structured nanogel, composed of linear chitosan chains interpenetrated into a thermos-sensitive nonlinear PEG chain network, for smart anti-cancer drug delivery.180 After incorporating the CDs into the semi-IPN structured chitosan–PEG nanogels, we obtained a pH, temperature, and NIR light triple-responsive chitosan–PEG@CDs hybrid nanogel for simultaneous biosensing, bioimaging, and synergistic therapy.162 Another type of dual responsive nanogel is formed by chemical-sensitive crosslinking bonds. For example, the disulfide bond (SS) is redox-responsive. Based on this method, drug-conjugated GO nanosheets have been incorporated into pH and glutathione dual responsive disulfide-bond linked HA nanogels. The resultant GO–SS–HA hybrid nanogels enable pH, glutathione, and NIR light triple responsive drug release.167 The design based on the chemical sensitive crosslinking-bond in nanogels will add more stimuli into the nanogels. For example, a polymer nanogel crosslinked with a trypsin-degradable peptide linker is responsive to the presence of enzyme,114 thus it is possible to develop multi-responsive carbon-based hybrid nanogels including enzyme stimulus.
Another way to prepare multi-responsive carbon-based hybrid nanogels is to incorporate hybrid carbon NPs sensitive to two or more external stimuli into the responsive polymer nanogels. A variety of dual responsive carbon-based hybrid NPs combining light absorbing and magnetic properties have been developed for multimodal imaging and magnetic field/NIR light responsive drug release.71 However, few of them have been incorporated into nanogels. The incorporation of magnetic NPs into responsive nanogels cannot only prevent the agglomeration of magnetic NPs, but also provide magnetic field-guided site targeting for remotely enhancing drug accumulation in the tumor site.181 Magnetic NPs can also generate heat when exposed to an appropriate alternating current (AC) magnetic field, which can be used for hyperthermia treatment to kill tumor cells.182,183 We have recently developed magnetic field and NIR light dual responsive carbon hybrid NPs (Fe3O4@PC–CDs) composed of magnetic iron oxide (Fe3O4) nanocrystals and NIR absorbing CDs embedded in a porous carbon matrix.86 After coating these dual responsive Fe3O4@PC–CD carbon hybrid NPs with a thermo-sensitive polymer nanogel, we were able to obtain NIR light, magnetic field, and thermos-triple responsive carbon-based hybrid nanogels.184 In addition to the incorporation of pre-synthesized dual responsive carbon hybrid NPs, multi-responsive carbon-based hybrid nanogels can be also fabricated by embedding two different types of functional NPs into a responsive nanogel. For example, Au NPs and CDs have been respectively incorporated into two sides of a pH-sensitive nanogel called Janus NPs,185 which provide a new strategy to synthesize multi-responsive carbon-based hybrid nanogels.
3. Synthesis of hybrid nanogels functionalized with different carbon nanomaterials
The carbon-based polymer hybrid nanogels are not produced from the simple mixing of carbon nano-objects with the post-synthesized polymer nanogels. Instead, each component needs rational design of their chemical moieties before the gelation to promote the intimate interactions or chemical bonding between the carbon nano-objects and the pre-polymer or pre-gel molecules. In such a way, the resulting hybrid nanogels will have stable nanostructures and properties to provide multiple functions, which will not only depend on each component’ nature, but also on the synergy between each component. Without any doubt, these new generations of carbon–polymer hybrid materials will open up new possibilities for applications in different areas of nanomedicine. In this section, the design and synthesis of carbon-based hybrid nanogels prepared from different carbon nano-objects, including CDs, GO or rGO nanosheets, fullerenes, CNTs, carbon NPs, and carbon ND, will be described.
3.1. Synthesis of CD-based hybrid nanogels
As a new member of the carbon material family, CDs with a size usually below 15 nm, have outshone other members of the carbon family and attracted tremendous attention in the past decade because of their outstanding properties of fluorescence, cyto-compatibility, photostability, electronic, mechanical and other chemical properties.186–194 These properties give CDs a great advantage over other fluorescent contrast agents (organic dyes, QDs, plasmonic NPs, and REM NPs).195–200 For example, CDs with abundant hydrophilic surface functional groups anchored on an aromatic π-conjugated carbon matrix display excellent solution dispersity with no need for further surface modification.201–203 A lot of chemicals and materials, such as glucose, citric acids, ethylenediamine, amino acids, polysaccharides, polyethyleneimine, and fruit peels have been used as carbon sources to synthesize the CDs, typically via solvothermal/hydrothermal or microwave-assisted pyrolysis. These synthetic methods endow several types of reactive functional groups produced on the surface of CDs, such as –COOH, –OH and –NH2 groups, depending on the carbon source. Consequently, the past few years have seen the emergence of different designs for the synthesis of CD-based hybrid nanogels in order to integrate the merits of CDs with stimuli-responsive polymer nanogels for a new type of multifunctional nanoplatform. The key for the successful synthesis of CD-based hybrid nanogels is to induce the NP formation of the CD-included pregel mixture in a continuous phase. Below we summarize a few typical synthetic routes to prepare responsive CD-based hybrid nanogels.
3.1.1. Precipitation polymerization.
With rational design of the compositions of pregel monomers and the surface functional groups on the CDs, responsive CD-based hybrid nanogels can be synthesized by precipitation polymerization. Precipitation polymerization is a heterogeneous polymerization process that begins with a homogeneous phase in water, where the monomers and initiator are completely soluble, but upon initiation the formed polymer is insoluble and thus precipitates to form nucleus seeds. The polymerization proceeds by absorption of monomers and initiator into the initially formed polymer seed particles until the completion of the reaction. Based on this principle, our group firstly developed a synthetic strategy to incorporate a type of fluorescent carbon NP (FCNPs or CDs, ∼13 nm) into thermo-sensitive copolymer nanogels using NIPAM and acrylamide (AAm) as comonomers and N,N′-methylenebisacrylamide (BIS) as a crosslinker in the presence of FCNPs bearing –COOH and –OH functional groups.166 The AAm monomer was designed to complex with the FCNPs via hydrogen bonding interactions with the surface –COOH and –OH groups on FCNPs. NIPAM was designed to enable the precipitation polymerization because the PNIPAM-dominant copolymer would become insoluble at a reaction temperature of 70 °C (above the LCST). Before initiation of polymerization, NIPAM, AAm–FCNP complexes, and BIS are all soluble in a homogeneous aqueous phase. Upon the addition of initiator, NIPAM and AAm (complexed with FCNPs) monomers copolymerize randomly to form insoluble copolymers at the reaction temperature, which “precipitate” as initial nucleus seed particles in water. The polymerization proceeds by absorption of the remaining monomer/crosslinker molecules into these initial seed particles until the reaction is completed, resulting in the immobilization of FCNPs in the thermos-responsive P(NIPAM–AAm) copolymer nanogel. Following the same strategy, we were able to immobilize the CDs bearing surface –COOH and –OH groups into a glucose sensitive copolymer nanogel.139 In this case, AAm was designed to complex with the CDs via hydrogen bonding interactions and NIPAM has the same function to form the insoluble copolymer at reaction temperature. In addition, a glucose-sensitive comonomer of VPBA was added into the reaction mixture. All the pregel components including NIPAM, AAm–CDs complexes, VPBA, and BIS are soluble in a clear aqueous phase, but their copolymers are insoluble in water at the reaction temperature. After initiating the copolymerization and giving enough time for the reaction to complete, glucose-sensitive hybrid nanogels with CDs immobilized in the P(NIPAM–AAm–VPBA) copolymer nanogel network were obtained. It has been proved that the coating of PEG chains on the surface of CDs can enhance the chemical and photoluminescence stabilities of CDs.187 Such PEG passivated CDs can also be successfully incorporated into the PNIPAM nanogel via a precipitation polymerization method (Fig. 2) to achieve high quantum yields (QYs) of the resultant PNIPAM–CDs@PEG hybrid nanogels.204
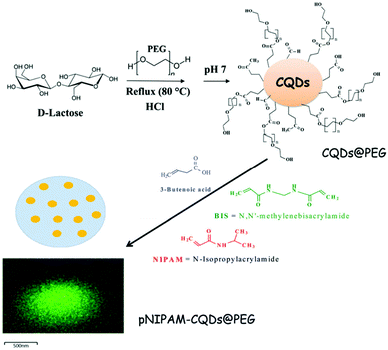 |
| Fig. 2 Schematic illustration of the synthetic route for the preparation of luminescent PNIPAM–CDs@PEG hybrid nanogels. Adapted from ref. 204 with permission (2017) of the Royal Society of Chemistry. | |
The precipitation polymerization method is not just good for the preparation of a PNIPAM-containing hybrid nanogel. In principle, any thermo-sensitive polymer with an LCST below the polymerization reaction temperature can be applied to prepare hybrid nanogels. Considering the tunable thermo-sensitivity of the recently developed nonlinear PEG polymers, we later synthesized a new type of CD-based hybrid nanogel from a crosslinked nonlinear PEG network interpenetrated with pH-sensitive chitosan biopolymer (PEG–chitosan@CDs) using the one-pot precipitation polymerization method.162 As shown in Fig. 3, the PEG macromonomers of (2-(2-methoxyethoxy)ethyl methacrylate) (MEO2MA) and oligo(ethylene glycol) methyl ether methacrylate (MEO5MA), the PEGdimethacrylate (PEGDMA) crosslinker, the chitosan chains, and the CDs bearing surface –COOH and –OH groups are all water soluble in a homogeneous phase. The chitosan chains and CDs are complexed with the MEO2MA and MEO5MA comonomers through the hydrogen bonding associations among the –OH/–NH2 groups of chitosan, the –COOH and –OH groups on the CDs, and the ether oxygens of the MEO2MA and MEO5MA monomers and PEGDMA crosslinker. When these PEG macromonomer/crosslinker molecules are polymerized at 70 °C, the copolymers are insoluble in water. The complexed chitosan chains and CDs are in situ trapped in the resulting nonlinear PEG networks.
 |
| Fig. 3 Schematic illustration of the formation of PEG–chitosan@CD hybrid nanogels. | |
The precipitation polymerization method has enabled the successful synthesis of other CD-based polymer hybrid nanogel systems using differently functionalized CDs or CD-containing hybrid NPs. We summarize the recent examples of carbon-based hybrid nanogels synthesized from different methods using CDs or other nanocarbons (GO, fullerenes, CNTs, and NDs) with different surface functional groups and different pregel components in Table 1.
Table 1 Examples of reported nanocarbon-based hybrid nanogels synthesized from different methods
Nano carbons |
Major surface functional groups |
Pregel monomers or polymers |
Crosslinker |
Synthetic method |
Nano structure |
Ref. |
CDs |
–COOH; –OH |
NIPAM; AAm |
BIS |
Precipitation polymerization |
Random distribution |
166
|
CDs |
–COOH; –OH |
NIPAM; AAm; VPBA |
BIS |
Precipitation polymerization |
Random distribution |
139
|
CDs |
PEG; 3-butenoic acid |
NIPAM |
BIS |
Precipitation polymerization |
Random distribution |
204
|
CDs |
–COOH; –OH |
MEO2MA; MEO5MA; chitosan |
PEGDMA |
Precipitation polymerization |
Random distribution |
162
|
CDs/SiO2 hybrid NP |
–COOH; –OH; –NH2 |
NIPAM; MAA |
BIS |
Precipitation polymerization |
Core–shell |
205
|
Fe3O4–CDs–PC hybrid NP |
–COOH; –OH |
NIPAM; AAm |
BIS |
Precipitation polymerization |
Core–shell |
184
|
CDs |
–COOH; –OH |
VPBA; AAm |
BIS |
Dispersion polymerization |
Random distribution |
307
|
CDs |
Vinyl |
NIPAM |
Vinyl-CD |
Emulsion polymerization |
Core–shell |
95
|
CDs |
Vinyl |
OrnAA (amino acid) |
Vinyl-CD |
Inverse microemulsion polymerization |
Random distribution |
122
|
CDs |
–COOH; –OH |
Chitosan |
Glutaraldehyde |
Non-solvent induced phase separation |
Random distribution |
123
|
CDs |
–NH2 |
PEGylated oxidized alginate |
NH2–CD–NH2 |
Hydrogen bonding association followed by amide crosslinking |
Random distribution |
124
|
CDs |
–NH2 |
PEGylated hyaluronic acid |
NH2–CD–NH2 |
Hydrogen bonding association followed by amide crosslinking |
Random distribution |
128
|
CDs |
–NH2 |
Alginate |
Ca2+ |
Micropipette dropping and crosslinking |
Core–shell |
125
|
CD |
–NH2 |
PVP; PAA-SH |
Disulfide |
LBL and in situ crosslinking |
LbL |
206
|
CDs |
–COOH; –OH; –NH2 |
PEG diacrylate |
PEG diacrylate |
Droplet-based microfluidics |
Random distribution |
129
|
CDs |
–COOH; –OH; –NH2 |
Alginate and carboxymethyl cellulose |
Ca2+ |
Centrifugal microfluidic chips |
Random distribution or Janus NPs |
187
|
rGO |
–COOH; –OH |
NIPAM, MAA |
BIS |
Mixing and adsorption |
Core–shell |
149
|
GO–DOX conjugates |
–COOH; –OH |
HA |
Cystamine (disulfide) |
Mixing and adsorption |
Core–shell |
167
|
rGO |
Acrylated chitosan |
NIPAM |
PEG diacrylate |
Precipitation polymerization |
Random distribution |
207
|
GO |
Salep |
NIPAM, AA |
BIS |
Precipitation polymerization |
Random distribution |
171
|
GO |
Alkenyl |
VPBA for core |
BIS |
Dispersion/precipitation polymerization |
Random distribution |
208
|
NIPAM for shell |
GO |
–COOH; –OH |
Alginate |
Cystamine (disulfide) |
Water–oil–water double emulsion |
Random distribution |
127
|
GO |
–COOH; –OH |
PHEA-DVS; HA-EDA |
Divinyl sulfone |
Formation of bulk hybrid gel followed by high pressure homogenizing |
Random distribution |
168
|
Fullerene |
HA |
HA grafted with fullerenes |
Fullerenes |
Self-assembly |
Random distribution |
209
|
Fullerene |
Glycol chitosan |
Glycol chitosan grafted with C60 and dimethylmaleic acid |
Fullerenes & electrostatic attraction |
Self-assembly |
Random distribution of C60 clusters |
210
|
Fullerene |
γ-Cyclodextrin (γ-CDx) |
PDEA nanogel |
BIS |
Incubation of C60–γ-CDx complexes with swollen nanogels |
Random distribution |
121
|
SWCNTs |
Chitosan |
NIPAM |
BIS, PEG diacrylate |
Precipitation polymerization |
Random distribution |
179
|
MWCNTs |
Gelatin |
Sodium methacrylate |
BIS |
Water–oil emulsion polymerization |
Random distribution |
169
|
MWCNTs |
–COOH; –OH |
NIPAM; AA |
Hectorite |
Microfluidic reactor |
Random distribution |
172
|
NDs |
–COOH; –OH |
NIPAM |
BIS |
Precipitation polymerization |
Random distribution |
211
|
NDs |
PEI |
N-Acetylated chitosan |
Amide |
Hydrogen bonding association followed by amide crosslinking |
Random distribution |
212
|
3.1.2. Emulsion/inverse emulsion polymerization.
Emulsion or inverse emulsion polymerization is another route to synthesize polymeric nanogels. Recently, Kim and Lee functionalized CDs with vinyl groups on their surface. The vinyl group modified CDs and fluorescein O-methacrylate in organic solvent were dropped into a water phase containing the surfactant of sodium dodecyl sulfate (SDS), NIPAM monomers, and BIS crosslinkers. The initiation of polymerization and crosslinking produced core–shell structured CD-based hybrid nanogels with the thermos-sensitive PNIPAM nanogel coated on the CD core.95
Inverse emulsion polymerization is a technique based on the hydrophilic monomers dissolved in aqueous solution emulsified by a water-in-oil emulsifier in a continuous oil phase followed by initiation of polymerization. For very hydrophilic polymers that do not display an LCST under normal reaction conditions, inverse microemulsion polymerization is a typical method to synthesize their polymer nanogels. As shown in Fig. 4, Liu's group was able to synthesize a type of zwitterionic polymer–CD hybrid nanogel from amino acid monomers using the inverse microemulsion polymerization method.122 The CDs were firstly functionalized with vinyl groups to form polymerizable/crosslinkable CDs. In the continuous oil phase (hexane), emulsifiers of Tween 80 and Span 80 as well as an oil-phase initiator of V-70 were firstly dissolved. In a separate water phase, the OrnAA (a newly developed amino acid) monomer molecules and the vinyl modified CDs were dissolved in phosphate buffer saline (PBS) solution. Under vigorous stirring, the water phase was added dropwise and dispersed into the continuous oil phase to form pregel droplets with further sonication. After the initiation of the polymerization reaction, a pOrnAA–CDs hybrid nanogel with CDs serving as chemical crosslinkers and embedded in the nanogel networks was produced. Interestingly, the content of the vinyl modified CDs in the reaction droplet could not only control the crosslinking density or swelling degree of the hybrid nanogels, but also control the overall size of the hybrid nanogels. Furthermore, the functional amino groups on the hybrid nanogels from the OrnAA units can be conjugated with targeting ligands such as folic acid.
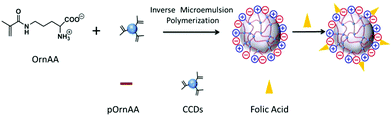 |
| Fig. 4 Schematic illustration of the preparation of pOrnAA–CDs hybrid nanogels conjugated with folic acid on the surface. Reproduced from ref. 122 with permission (2016) of Elsevier. | |
3.1.3. Non-solvent induced phase separation and CD-induced association of polysaccharides.
Polysaccharide-based nanogels have attracted attention as drug nanocarriers due to their unique multi-functional groups in addition to their biocompatibility, biodegradability, large surface area for multivalent bioconjugation, tunable size, and interior network for the incorporation of different pharmaceutical agents.213 Several types of responsive CD-based polysaccharide hybrid nanogels have been recently synthesized for multifunctional drug carriers. The simplest method is to immerse the alginate microgel beads into the CD aqueous solution. The surface –NH2 groups on the CDs and the –COOH/–OH groups on the alginate chains can form hydrogen bonding association and thus the CDs can be coated onto the surface of the alginate microgel particles.125 We have developed a type of chitosan-CD hybrid nanogel with size ∼65 nm based on a nonsolvent-induced phase separation method.123 CDs and chitosan dissolved in water can form complexes through the hydrogen bonding association between the –OH/–NH2 groups on the chitosan chains and the –COOH and –OH groups. When a small amount of ethanol, a nonsolvent to chitosan, was added into the clear CD–chitosan mixture solution under vigorous stirring, the phase separation of the chitosan–CD complexes from water induces the formation of small colloidal NPs assisted by ethylenediaminetetraacetic acid (EDTA) molecules. The addition of glutaraldehyde can crosslink the chitosan chains in the complex NPs, producing stable chitosan–CD hybrid nanogels. Liu's group developed another strategy to synthesize polysaccharide–CD hybrid nanogels.124,128 They firstly synthesized CDs containing abundant –NH2 groups on their surface from one-step microwave pyrolysis of citric acid/ethylenediamine mixture solution. In one system, they grafted the methoxypolyethylene glycol amine (mPEG-NH2) to the oxidized alginate (OAL) chains via the coupling reaction between the terminal –NH2 group of mPEG and the –COOH groups of OAL in the presence of 1-(3-(dimethylamino)propyl)-3-ethylcarbodiimide (EDC) and N-hydroxysuccinimide (NHS), resulting in mPEG–OAL polysaccharide backbone chains grafted with some PEG chains. The addition of the –NH2 group capped CDs into the mPEG–OAL aqueous solution can induce the formation of mPEG–OAL–CD NPs through the hydrogen bonding associations between the mPEG–OAL chains and –NH2 capped CDs. The subsequent EDC catalyzed coupling reaction between the –NH2 groups on the CDs and the –COOH groups on the OAL chains produced the CD-crosslinked mPEG–OAL–CDs hybrid nanogels.124 In another system, they synthesized PEGylated HA–CD hybrid nanogels bearing targeting ligands of folic acid (FA) based on a similar strategy.128 As shown in Fig. 5, the FA–PEG–NH2 chains can be grafted onto the HA chains via an EDC coupling reaction between the terminal –NH2 group of the FA–PEG chains and the –COOH groups of the HA chains, resulting in FA–PEG–HA chains. The addition of the –NH2 capped CDs into the FA–PEG–HA aqueous solution induces the formation of FA–PEG–HA–CD hybrid NPs through hydrogen bonding associations. The subsequent amidation between the –NH2 groups on the CDs and the –COOH groups on the HA chains in the NPs produces FA–PEG–HA–CDs hybrid nanogels. The attached FA moieties on the grafted PEG chains on the surface of the hybrid nanogels can serve as a targeting ligand for specific types of cancer cells.
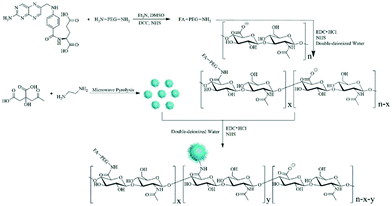 |
| Fig. 5 Schematic illustration of the synthesis of the FA–PEG–HA–CD hybrid nanogels. Reproduced from ref. 128 with permission (2016) of Elsevier. | |
3.1.4. Microfluidic technology.
Microfluidic technology is another method to prepare polymer microgels embedded with functional CDs. In general, microfluidic devices allow the production of uniform small-sized droplets of fluid from single or multiple channels, which enable the synthesis of microgels with different compositions and architectures. For example, droplet based microfluidics has been recently used to synthesize PEG–CD hybrid microgels.129 The microfluidic device consists of a simple arrangement of channels (tubes) and needles to generate a coaxial inner stream of pregel solution surrounded by an outer oil phase (light paraffin liquid with 4% span 80). The inner stream of pregel solution, consisting of PEG–diacrylate (Mw = 10 kDa), CDs, and Irgacure 2959 (photo-initiator) in PBS, breaks into micrometer sized droplets due to the shearing force of the outer fast moving oil phase. The droplets generated in this way can then be polymerized by an exposure to UV light (365 nm) for 30 second, producing crosslinked PEG–CD hybrid microgels. This droplet microfluidic method also allows the simultaneous loading of cells into the PEG–CD hybrid microgels by simply adding the cells into the pregel solution before injection into the inner stream channel for droplet formation.
In another example, Yue et al. designed a centrifugal microfluidic device that enables the synthesis of single-phase or Janus hybrid microgel particles (Fig. 6).185 The pregel solution, consisting of a mixture of sodium alginate and carboxymethyl cellulose (CMC) dissolved in an aqueous dispersion of colloidal Au NPs or CDs can be injected into the eight radial channels on the microfluidic chip. The centrifugation of the microfluidic chip mounted on a spin coater can produce micrometer-sized droplets of the pregel solution, which can then be crosslinked by the CaCl2 solution placed in the circumjacent chambers opposite to the channel outlets, resulting in single phase alginate–CMC–Au NP or alginate–CMC–CD hybrid microgels. One advantage of this method is that the composition of the hybrid microgels can be tailored by adding different types of functional NPs such as Au NPs or CDs into the pregel solution. The microfluidic method also allows the fabrication of Janus hybrid microgels by simply injecting the alginate–CMC–AuNP pregel solution and the alginate–CMC–CD pregel solution separately into a pair of adjacent channels and then centrifuging. In such a way, the two pregel solutions containing different types of NPs centrifuged out from the adjacent channels merge into the same droplet at the outlet of the channels, resulting in two different hybrid microgels occupying each of the hemispheres of the droplet.
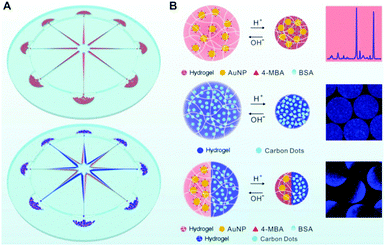 |
| Fig. 6 (A) Schematic illustration of the synthesis of single-phase (upper left) and Janus (lower left) hybrid nanogels via centrifugal microfluidic chips. (B) pH-induced swell and shrink of single phase alginate–AuNP hybrid nanogels (upper right), single-phase alginate–CD hybrid nanogels (middle right), and Janus alginate nanogels encapsulating AuNPs and CDs in dual hemispheres (lower right). Reproduced from ref. 185 with permission (2017) of the American Chemical Society. | |
3.2. Synthesis of GO-based hybrid nanogels
Graphene, a two-dimensional (2D) carbon lattice with a closely packed honeycomb structure, has a huge family including single- and multi-layered graphene, GO, rGO and doped graphene.106,214 Due to a wealth of fascinating properties such as giant electron mobility, high Young's modulus, optoelectronic properties, large specific surface area, and biocompatibility, graphene has aroused tremendous interest in various areas including energy, catalysis and devices,214–217 especially in biomedical areas.215,217,218 For example, the strong NIR-absorption and excellent photothermal conversion ability of GO and rGO make them powerful agents for photothermal therapy (PTT).219,220 In addition, the 2D planar aromatic structure combined with the –OH/–COOH polar groups on GO and rGO nanosheets enable them to interact with many drugs or biomolecules via π–π stacking, van der Waals interaction, ionic interactions, or hydrogen bonding, imparting abilities for drug or gene delivery218,221–225 and fabrication of biosensors.226 Despite these merits mentioned, graphene or its derivatives could encounter agglomeration issues in buffers and serum and thus require further surface functionalization.227 The ever increasing demand for more functionality in biomedicine inspired researchers to combine the beneficial characteristics of graphene derivatives and polymers for a synergetic effect.168,228,229 In particular, different synthetic strategies have been explored to incorporate GO nanosheets into stimuli-responsive polymer nanogels to develop new multifunctional nanoplatforms.127,149,167,168,171,207,208 Below we summarize the currently developed synthetic methods to prepare GO-based hybrid nanogels.
3.2.1. Mixing of polymer nanogels with GO derivatives.
Lu et al. prepared a type of core–shell like GO-based hybrid nanogel using a facile solution mixing method.149 Specifically, they synthesized a core–shell structured nanogel with the homo-PNIPAM nanogel as the core and the poly(NIPAM-co-methacrylic acid) [P(NIPAM–MAA)] copolymer nanogel as shell. Afterwards, these core–shell structured PNIPAM@P[(NIPAM–MAA)] nanogel dispersions at certain concentrations were respectively added to a fixed amount of previously synthesized rGO dispersion under stirring to achieve different ratios of rGO to PNIPAM nanogels. The continuous gentle agitation of these mixtures for 24 h produced a series of rGO–PNIPAM hybrid nanogels with the rGO nanosheets mainly adsorbed on the outer layer of the P(NIPAM–MAA) nanogel, forming a core–shell GO-based hybrid nanogel with the homo-PNIPAM nanogel in the core and the rGO–P(NIPAM–MAA) hybrid nanogel as a dense shell. Interestingly, the content of rGO in the hybrid nanogels can significantly change the photo- and thermos-sensitivity of the PNIPAM@rGO–P(NIPAM–MAA) hybrid nanogels. Different from the coating of small rGO nanosheets onto the PNIPAM@P(NIPAM–MAA) nanogel template in this system, Lee's group synthesized a new type of hybrid nanogel by coating pH-sensitive HA nanogels onto a GO-based nanoparticle core template (Fig. 7).167 They firstly functionalized the GO nanosheets with different amounts of the anticancer drug doxorubicin (DOX), resulting in a series of GO–DOX conjugated NPs with different particle sizes ranging from 21 nm to 70 nm. In a separate synthesis, they prepared disulfide crosslinked HA nanogels. Coating of the GO–DOX conjugate NPs with the crosslinked HA nanogels can be obtained by simple physical agitation. Specifically, the GO–DOX NPs dispersed in DMSO were added dropwise to the cross-linked HA nanogels in the water phase under stirring. The continuous stirring of the mixture for 2 h produced a uniform GO–DOX–HA hybrid nanogel. The hybrid nanogel dispersed in the dimethyl sulfoxide (DMSO)/water mixture can be dialyzed against water for sufficient time to remove DMSO. The overall size of the hybrid nanogels depends on the size of the GO–DOX core template NPs. The larger the GO–DOX core NPs, the more the HA nanogels coated onto the core NPs, resulting in a larger size of the final GO–DOX–HA hybrid nanogel particles.
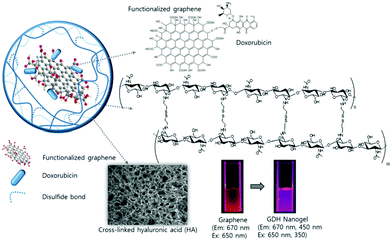 |
| Fig. 7 Schematic illustration of the light responsive GO–DOX–HA (GDH) hybrid nanogels. Reproduced from ref. 167 with permission (2015) of the Royal Society of Chemistry. | |
It should be mentioned that although the method based on physical mixing from the post-synthesized nanogels and GO derivative is simple, it is possible that a small amount of the rGO or GO–DOX nanosheets embedded in the PNIPAM or HA nanogels may diffuse out after the nanogels undergo frequent swelling/shrinking transitions under certain conditions. Thus, such prepared hybrid nanogels may not be a good candidate for biosensor applications, where the optical signal must be fully reversible under different sensing conditions. In situ immobilization of GO nanosheets in polymer nanogels should be a better synthetic strategy to achieve highly stable GO-based hybrid nanogels.
3.2.2. Precipitation polymerization.
Precipitation polymerization, starting from the functionalized GO nanosheets dispersed in water-soluble monomer aqueous solution, is a good method to immobilize the GO nanosheets in situ in the polymer nanogels. Several GO-based hybrid nanogel systems have been synthesized from the precipitation polymerization method in the presence of NIPAM as co-monomers. For example, Wang et al. have synthesized a thermos and NIR light-sensitive PNIPAM–chitosan–rGO hybrid nanogel (Fig. 8).207 They firstly functionalized the rGO with chitosan chains and then further grafted acrylate groups onto the chitosan chains using the EDC coupling reaction between –COOH and –NH groups. This modification of the rGO not only increases the water dispersity of rGO, but also provides polymerizable vinyl groups on the rGO. These rGO nanosheets modified with the acrylated chitosan chains can then complex with the NIPAM monomers and PEG diacrylate crosslinker through hydrogen bonding and hydrophobic associations in the clear pregel solution. The copolymerization reaction initiated at 70 °C of NIPAM, PEG diacrylate, and acrylated chitosan modified rGO comonomers produced nonsoluble initial PNIAPM–chitosan–rGO hybrid nanogel seed particles, which can be stabilized by the hydrophilic chitosan and PEG components. The polymerization proceeds by absorption of the remaining monomers into the initially formed nanogel seed particles until the completion of the reaction. The resultant PNIPAM–chitosan–rGO hybrid nanogels become soluble at temperatures below their VPTT of ∼38 °C. Such prepared hybrid nanogels are very stable because the rGO nanosheets are chemically immobilized in the interior of the nanogel network. Based on a similar principle, Bardajee et al. synthesized a type of P(NIPAM–AA)–salep–GO hybrid nanogel.171 In this system, the GO nanosheets were firstly mixed with the salep solution under ultrasonic agitation to form a homogeneous dispersion of salep-modified GO in water. The NIPAM/AA comonomers and BIS crosslinkers were then added into the salep-modified GO dispersion solution to form complexes. The initiation of the polymerization reaction at 70 °C resulted in P(NIPAM–AA)–salep–GO hybrid nanogels with GO nanosheets randomly immobilized in the P(NIPAM–AA) nanogel network. On another system, Zhou et al. synthesized a glucose-responsive GO-based hybrid nanogel.208 The GO nanosheets were firstly modified with alkenyl groups to become polymerizable. The resultant alkenyl-GO nanosheets were then directly copolymerized and crosslinked with the VPBA monomers in the presence of surfactant SDS as a dispersing agent, producing glucose-responsive GO–P(4-VPBA) hybrid nanogels. Additional neutral thermo-sensitive PNIPAM nanogel shells were added onto these GO–P(4-VPBA) hybrid nanogel particles by using the precipitation polymerization method, resulting in the final stable GO–P(4-VPBA)@PNIPAM core–shell structured hybrid nanogels.
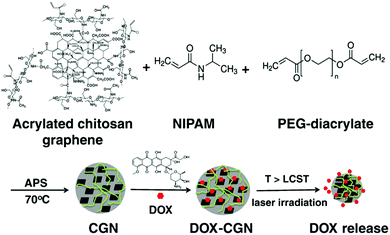 |
| Fig. 8 A schematic illustration of the synthesis of the PNIPAM–chitosan–rGO hybrid nanogels and the DOX loading and releasing from the hybrid nanogels. Reproduced from ref. 207 with permission (2013) of Elsevier. | |
3.2.3. Double emulsion followed by in situ crosslinking.
Xu et al. recently synthesized GO–alginate hybrid nanogels based on a water–oil–water double emulsion method followed by in situ crosslinking.127 The –COOH groups on the alginate chains and GO nanoplatelets were firstly activated by EDC. After that, the mixture of alginate and GO in a water phase was emulsified into dichloromethane by using dioctyl sodium sulfosuccinate as a surfactant, forming water–oil droplets. These water–oil droplets were further emulsified into an aqueous solution of poly(vinyl alcohol) (PVA), forming water–oil–water double emulsion droplets. The in situ crosslinking of the GO–alginate mixture in the minidroplets by disulfide-containing cystamine produces GO–alginate hybrid nanogels. After the removal of dichloromethane under vaporization overnight, the hybrid nanogels can be purified by centrifugation and repeated washes with water.
3.2.4. Formation of a bulk hybrid gel followed by high pressure homogenizing.
Unlike the existing strategies for preparation of polymer nanogels, Fiorica et al. recently developed a new strategy to fabricate GO-based hybrid nanogels.168 This new method permits the fine control of particle size, composition, and dispersity of the GO-based hybrid nanogels. For example, they have prepared a double network-structured GO–polymer hybrid nanogel using this two-step top down method (Fig. 9). Specifically, they synthesized two different polymers bearing different functional groups. One is hyaluronic-((2-aminoethyl)-carbamate)acid (HA-EDA) and another is α,β-poly(N-2-hydroxyethyl)-D,L-aspartamide-divinyl sulfone (PHEA-DVS). At step one, a bulk hybrid hydrogel was first obtained by Michael-type addition of the amino-containing HA-EDA with the PHEA-DVS–GO mixture solution at pH 10.0. The amine functions of HA-EDA were exactly balanced by the DVS functions of PHEA-DVS, thus forming a rigid double network without excess both double bond and amine groups. Then the second step was applied to produce hybrid nanogels from the bulk hybrid gel by using an ultraturrax and high pressure homogenizer (30 kpsi). After three cycles of homogenization, small sized HA-EDA–PHEA-DVS–GO hybrid nanogels were obtained.
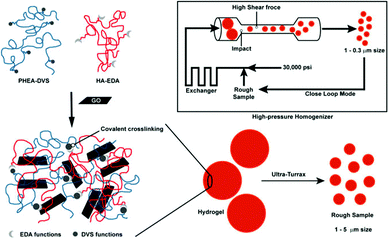 |
| Fig. 9 A schematic illustration of the process involved in the preparation of HA-EDA–PHEA-DVS–GO double network structured hybrid nanogels. Reproduced from ref. 168 with permission (2017) of the American Chemical Society. | |
3.3. Synthesis of fullerene-based hybrid nanogels
Fullerenes, molecules of carbon in the form of a hollow sphere, have attracted much attention because of their unique physical and chemical properties that differ from other traditionally used carbon materials since their discovery in 1985.230,231 For example, the high electroconductivity, good thermal conductivity, high electrochemical stability and special mechanical properties of fullerenes enable their wide applications in adsorption electrodes, screen printed systems, solar cells and other devices.232,233 The special well-ordered structure of fullerenes with hollow carbon clusters endows them with the possibility to be used as robust containers for other species. Until now, although the research on fullerenes in energy-related industries has achieved great progress, the development of fullerenes in biomedical areas is relatively slow due to their poor water-solubility.234 The functionalization of fullerenes is a good strategy to overcome the poor water-solubility. Fullerene derivatives have showed great potential for biomedical applications including anti-HIV,235–237 antibacterial and antiviral activity,237–239 antioxidation,237,240 and especially cancer therapy and diagnosis.237,241,242 For example, polyhydroxylated fullerenes have demonstrated promise for photoacoustic (PA) imaging and PTT.243 Notably, fullerenes can efficiently transfer triplet excited energy to oxygen molecules, resulting in the generation of ROS in a high yield under light illumination, which makes them a potential photosensitizer for photodynamic therapy (PDT).244,245 With all these advantages and promises, however, fullerene derivatives are still faced with poor tumor-targeting ability and responsive drug delivery.246 A hybrid system with stimuli-responsive nanogels may be a solution that cannot only enhance biocompatibility and tumor-targeting ability, but also bring in new features that the nanogel possesses, resulting in a multifunctional platform for cancer theranostics.209,210,247,248 Here, we summarize two available synthetic strategies for the formation of fullerene-based hybrid nanogels.
Lee's group firstly did chemical conjugation of fullerenes (C60) to the HA chains (HA-g-C60). The π–π stacking between C60 fullerenes plus the hydrogen bonding associations between the HA chains induce the self-assembly of the HA-g-C60 chains, resulting in spherical hybrid nanogel particles with C60 aggregates serving as crosslinking points.209 Using a similar association method from the C60-grafted polysaccharide chains, they later incorporated the C60 fullerene clusters into glycol chitosan (GC) nanogels.210 Specifically, they grafted the glycol chitosan chains in two steps with 2,3-dimethylmaleic acid (DMA) and C60 fullerenes respectively. These grafted polysaccharide chains of GC-g-DMA-g-C60 self-assembled to hybrid nanogels in aqueous solution due to (1) π–π stacking between the C60 fullerenes and (2) the electrostatic interactions between pendant DMA groups and the residual free amine group of GC at pH 7.4. The aggregated C60 clusters are randomly embedded in the polymer gel matrix. The size of the GC-g-DMA-g-C60 hybrid nanogels can be controlled by pH change. For example, the cleavage of the DMA moieties from the GC chains at pH = 5.0 can reduce the inter-chain electrostatic interactions, thus the relatively large hybrid nanogel aggregates can be separated into small hybrid nanogels, which provides potential of the GC-g-DMA-g-C60 hybrid nanogel aggregates for targeting endosomal pH (∼5.0) in tumor cells.
Sugikawa et al. recently developed a different protocol to incorporate fullerenes into pH-responsive nanogels (Fig. 10).121 Specifically, the fullerenes (C60/70) are solubilized by γ-cyclodextrin, forming water-soluble C60/70–γ-CDx complexes. On the other hand, the pH-responsive nanogels are synthesized from the emulsion polymerization of DEA in the presence of 1.0 wt% bifunctional crosslinker. The resultant poly(2-(diethylamino)ethyl methacrylate) (PDEA) nanogels undergo a swelling/shrinking volume phase transition with the critical transition pH = 7.8, because of the protonation and deprotonation of the diethylamino groups. At pH < 7.8, the PDEA nanogels are swollen with large mesh size, thus the incubation of the C60–γ-CDx complexes with the swollen nanogels in buffer solution (pH = 5.9) leads to the successful complex-to-nanogel transfer of the fullerene molecules. However, at pH > 7.8, the transfer of the fullerenes to the nanogels is unfavored due to the shrunken nanospace of the nanogel. Interestingly, they found that the fullerene-incorporated hybrid nanogels are very stable. No fullerene release from the nanogels was detected even when the pH was adjusted up to pH 7.8. The presence of the C60 in the nanogels also did not change the physical properties of the nanogels, including the pH-dependent swelling/shrinking transition curve.
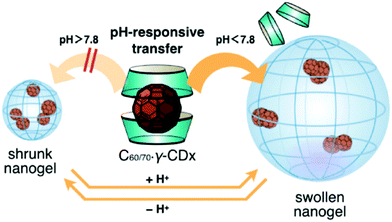 |
| Fig. 10 Schematic illustration of the pH-responsive transfer of fullerenes from a γ-CDx-based complex to a nanogel by the exchange method and the reversible pH-responsive phase transition of the fullerene-incorporated nanogel. Reproduced from ref. 121 with permission (2016) of the Chemical Society of Japan. | |
3.4. Synthesis of CNT-based hybrid nanogels
As one-dimensional (1D) carbon nanomaterials, carbon nanotubes can be composed of a single graphitic layer or multiple coaxial layers, resulting in single-walled carbon nanotubes (SWCNTs) and multiple-walled carbon nanotubes (MWCNTs).249–252 CNTs have attracted a lot of attention due to their unique mechanical, chemical, electrical, and optical properties, which provides them with great potential for applications in a broad range of fields from optoelectronics to biomedicine.253–259 For example, CNTs have been utilized as photothermal agents and photosensitizers for PTT and PDT in cancer treatment in vitro and in vivo.260–262 CNTs have been used as fluorophores for labeling in living systems for deep tissue fluorescence imaging.263–265 However, the difficult surface functionalization and intrinsic poor solubility of CNTs have hindered further biomedical applications.266 To overcome these issues, CNTs have been hybridized with many biopolymers and hydrogels for a variety of biomedical applications.267 For example, CNTs have been functionalized and incorporated into hydrogels to produce mechanically and electrically enhanced 3D porous networks as potential scaffolds for tissue construction.268 The CNT–polymer composite hydrogels have also been extensively studied for drug delivery, with particular attention to the controlled release by external stimuli.269–272 However, the majority of the reported CNT–polymer hybrid hydrogels are bulk gels although sometimes they were called nanogels.120 Only a limited number of examples have been reported for the preparation of CNT-based hybrid nanogels/microgels. In general, the small sized CNT-based hybrid nanogels/microgels were prepared by precipitation polymerization, water-in-oil emulsion polymerization, and microfluidic technology. Since the principles of these synthetic methods have been discussed in the synthesis of CD-based or GO-based hybrid nanogels, below we will just briefly summarize the synthetic routes of the CNT-based hybrid nanogels from different functionalizations of CNTs and polymers.
Qin et al. have synthesized a type of stimuli-responsive CNT-based hybrid nanogel using the precipitation polymerization method in the presence of NIPAM monomers.179 The SWCNTs were firstly acidified and truncated and then treated with sodium cholate. Such treated CNTs were then incubated with the oleic acid grafted chitosan solution to produce water-dispersible chitosan-wrapped CNTs. To prepare the CNT-based hybrid nanogels, an appropriate amount of NIPAM monomers with PEG diacrylate and BIS crosslinkers were then added dropwise into the aqueous dispersion solution of the chitosan-wrapped CNTs. Then the polymerization was initiated at 70 °C under protection of Ar, resulting in the CNTs–chitosan–PNIPAM hybrid nanogels with the chitosan-wrapped CNTs randomly embedded in the PNIPAM nanogels. Spizzirri et al. have prepared spherical CNT–gelatin hybrid microgels by using the water-in-oil emulsion polymerization method.169 In this system, MWCNTs were firstly dispersed into the gelatin aqueous solution by ultrasonication. These gelatin-wrapped CNTs were dispersed in water at different concentrations. Then the sodium methacrylate (NaMA) co-monomer and BIS crosslinker were dissolved into the gelatin-wrapped CNT solutions, forming the dispersion aqueous phase in the emulsion polymerization process (Fig. 11). A mixture of n-hexane and chloroform was used as an oil phase. Span 85 and tween 85 were used as emulsifiers. After the initiation of the polymerization, spherical CNT–gelatin–PNaMA hybrid microgels were produced. Another method to prepare the CNT-based hybrid nanogels is to use a microfluidic reactor. Hou et al. have synthesized CNTs–hectorite–P(NIPAM–AA) hybrid microgels using a microfluidic reactor.168 The MWCNTs were oxidized in concentrated H2SO4 and HNO3 to form water dispersible CNTs. These oxidized CNTs together with the NIPAM monomer, AA monomer, hectorite, and potassium persulfate were then dissolved in water as the aqueous phase solution. N,N,N′,N′-tetramethyldiamine dispersed in soybean was used as the oil phase solution. The two solutions were then injected into microcapillaries by two syringe pumps. Aqueous droplets were generated in the oil phase solution and then polymerized.
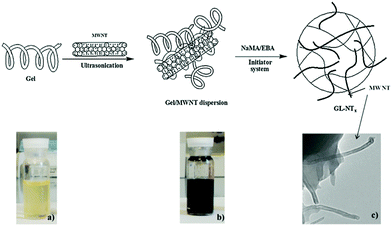 |
| Fig. 11 Schematic representation of the synthesis of CNTs–gelatin–PNaMA hybrid nanogels. (a) Gelatin solution in water; (b) MWNTs dispersion in gelatin solution; (c) morphology of the hybrid nanogels. Reproduced from ref. 169 with permission (2013) of Elsevier. | |
3.5. Synthesis of ND-based hybrid nanogels
As a type of carbon family material, NDs are three-dimensional (3D) sp3-bonded carbons with a distinctive faceted surface architecture where, depending on the size and morphology, the sp3-surface of the NDs is stabilized through either termination with functional groups or reconstruction of sp3 carbon into sp2 carbon.273 The presence of such surface functional groups on the NDs allows them (i) to coordinate water molecules around the surface and ensure good dispersion in an aqueous medium/matrix and (ii) to interact chemically with specific molecules or drugs in a biocompatible environment.274–277 Due to their excellent biocompatibility in many in vitro and in vivo studies,276,278,279 NDs have been widely utilized for biomedical imaging, sensing and therapeutic delivery.280–285 In order to further widen and enhance the applications in biomedical areas, NDs have previously been incorporated into polymer matrices or hydrogels to improve their mechanical properties and/or elute therapeutic compounds.286–291 In addition to these bulk composites or hybrid gels, small NDs–polymer hybrid NPs were also recently prepared by chemical coating of NDs with hyperbranched polyglycerol and polyamidoamine (PDA) for biomolecule labeling, anticancer drug delivery, and gene delivery.292–295 However, only a few studies have reported the synthesis of ND-based hybrid nanogels.211,212
Girard et al. encapsulated NDs into thermos-sensitive PNIPAM nanogels for the first time using the precipitation polymerization method. The carboxylic group functionalized NDs suspended in a colloidal solution were directly added into a reaction mixture containing NIPAM monomer and BIS crosslinker. The initiation of the polymerization at 70 °C resulted in NDs–PNIPAM hybrid nanogels.211 Kim et al. applied a different strategy to synthesize the ND-based hybrid nanogels.212 Pristine NDs were first air oxidized at a high temperature to form carboxylated NDs and then dissolved into anhydrous DMSO solvent with the aid of ultrasonication. Afterwards, polyethylenimine (PEI) chains were coupled onto the surface of NDs via EDC coupling reaction between the amine groups on the PEI chains and the surface –COOH groups on the NDs. The resulting ND–PEIs only show a slight increase in size compared to the carboxylated NDs, indicating that the ND–PEI NPs stay as monomers in the aqueous phase. These ND–PEI NPs were then further conjugated with excess succinic acid to yield carboxylated ND–PEIs. When these carboxylated ND–PEI NPs were mixed with N-acetylated chitosan (50% degree of acetylation) chains in aqueous solution, ND–PEI–chitosan complex NPs were formed through the hydrogen bonding associations between the chitosan chains and PEI chains. The EDC coupling reaction between the –COOH groups on the carboxylated ND–PEIs and the amine groups on the chitosan chains in the complex NPs can then in situ crosslink the PEI–chitosan chains, forming stable ND–PEI–chitosan hybrid nanogels. When drug molecules (e.g., timolol) were presented during the complexation process between ND–PEIs and N-acetylated chitosan in solution, the in situ crosslinking of the complex NPs could naturally trap the drug molecules into the hybrid nanogels (Fig. 12). Such prepared drug-loaded ND–PEI–chitosan hybrid nanogels can be further embedded into a bulk hydrogel contact lens as an effective ocular drug delivery platform.
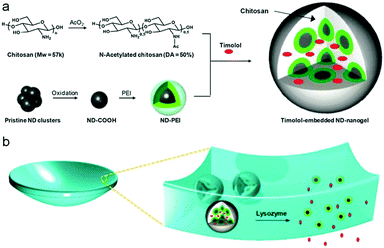 |
| Fig. 12 Schematic illustration of (a) the synthetic strategy of drug-loaded ND–PEI–chitosan hybrid nanogels and (b) the lysozyme-activated drug eluting contact lens embedded with the drug-loaded ND–PEI–chitosan hybrid nanogels. The lysozyme can cleave the N-acetylated chitosan and degrade the embedded hybrid nanogels, leading to drug release while leaving the lens intact. Reproduced from ref. 212 with permission (2014) of the American Chemical Society. | |
4. Nanostructures of carbon-based hybrid nanogels
The morphologies and nanostructures are important parameters for particles used in biological applications. For instance, it has been demonstrated that particles with high aspect ratios can successfully evade phagocytosis and exhibit prolonged blood circulation time in comparison with spherical particles of similar diameter.296,297 Polygonal NPs usually outperform nanospheres in terms of extravasation into tumors, since NP extravasation into tumors is proportional to their bioavailability.298 On the other hand, the nanostructures of hybrid nanogels associated with the distribution of carbon nano-objects, interactions of the carbon nano-objects with surrounding materials, and introduction of other functional materials could determine the multi-functions for their end use in nanomedicine. Depending on the designed synthetic strategies, the shape and nanostructures of the carbon-based hybrid nanogels can be very different. We classify the nanostructures in terms of the distribution of carbon nano-objects in the hybrid nanogels as (a) random distribution and (b) core–shell structure. The nanostructures of the recently developed carbon-based hybrid nanogels are summarized in Table 1.
4.1. Hybrid nanogels with carbon nano-objects randomly distributed
So far, the majority of the synthetic methods for hybrid nanogels are based on the droplet formation of pregel solutions containing different amounts of carbon nano-objects such as fullerenes, CDs, GO nanosheets, and CNTs. Hybrid nanogels prepared in such a way, including precipitation polymerization, emulsion polymerization, microfluidic polymerization, and phase separation in an aqueous phase, are all spherical in shape. Because the carbon nano-objects are designed either polymerizable in the pregel solution or to interact with one or more polymerizable components in the pregel solution via secondary forces (e.g., hydrogen bonding, van der Waals forces, or electrostatic interactions), the resultant hybrid nanogels typically have the carbon nano-objects randomly distributed through the whole spherical particle. To confirm this type of nanostructure, transmission electron microscopy (TEM), especially high resolution TEM (HRTEM), scanning electron microscopy (SEM), and atomic force microscopy (AFM) are very powerful techniques to identify the location and distribution of the carbon nano-objects in the gel matrix.
Fig. 13 shows a few typical examples of carbon-based hybrid nanogels with carbon nano-objects randomly distributed in the polymer nanogel matrix. First, although the three hybrid nanogels are composed of different polymers and carbon nano-objects with CDs embedded in the PEG–chitosan nanogels (A), rGO nanosheets embedded in the PNIPAM–chitosan nanogels (B), and NDs encapsulated in the PNIPAM nanogels (C), respectively, all three types of hybrid nanogels display a spherical shape. The reason is that all these hybrid nanogels were prepared from the precipitation polymerization of a pregel solution containing the carbon nano-objects. Second, the magnified images confirm that all the hybrid nanogels have carbon nano-objects randomly distributed in the gel matrix. In the PEG–chitosan@CD hybrid nanogels, the dark CDs of irregular size and shape randomly embedded in the nanogel can be clearly seen (Fig. 13A and B).162 Relative to the PEG–chitosan@CD hybrid nanogels, the PNIPAM–chitosan–rGO hybrid nanogels have much higher loading of the dark carbon nano-objects. It seems that the dark rGO nanosheets appear in small clusters, which are randomly distributed through the whole nanogel particle (Fig. 13C and D).207 Different from the TEM images of PEG–chitosan@CDs and PNIPAM–chitosan–rGO hybrid nanogels, the SEM images of the PNIPAM–NDs hybrid nanogels show some bright spots, which can be attributed to NDs (Fig. 13E and F).211 The NDs are scattered within the nanogel spheres but not everywhere on the substrate. It is also notable that the NDs are not observable in all the nanogel particles. The reason might be that the SEM only focuses on the surface of the hybrid nanogels and cannot penetrate through the particle. Overall, it can be seen that the loading content of the carbon nano-objects in the nanogel matrix can be very different even though they all are randomly distributed. The feeding concentration, size, and surface functionalization of the carbon nano-objects in the pregel solution should be key factors to control their loading content in the hybrid nanogels.
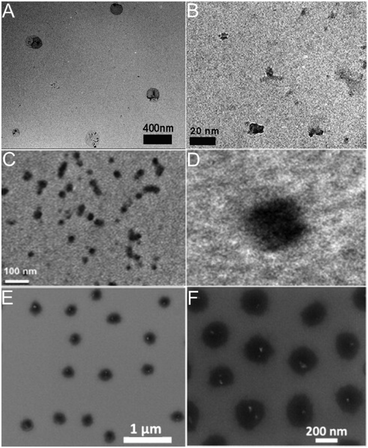 |
| Fig. 13 (A and B) TEM images of the PEG–chitosan@CD hybrid nanogels with the highly magnified section on the right. Reproduced from ref. 162 with permission (2015) of Wiley. (C and D) TEM images of PNIPAM–chitosan–rGO hybrid nanogels with the highly magnified single particle on the right image. Reproduced from ref. 207 with permission (2013) of Elsevier. (E and F) SEM images of the PNIPAM–ND hybrid nanogels with high magnification image on the right. Reproduced from ref. 211 with permission (2012) of Elsevier. | |
Besides the spherical shape, polygonal carbon-based hybrid nanogels were reported recently. Both the coupling SEM image and AFM image indicate that the double network structured HA-EDA–PHEA-DVS–GO hybrid nanogels, produced from the bulk hybrid gel by homogenization using an ultraturrax and high pressure homogenizer, display a polygonal shape no matter whether in a dried state or swollen state (Fig. 14).168 No free GO flakes were observed, implying that GO was completely incorporated into the double polymer networks. In contrast, the AFM micrographs highlighted the presence of GO nanosheets with a lateral size of about 250 nm inside the nanogel network. The AFM phase imaging also shows that the local elastic modulus at the surface of the GO–polymer hybrid nanogel was quite heterogeneous, owing to the composite nature of the hybrid nanogels, varying approximately from 0.5 to 10 MPa.
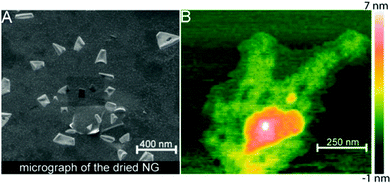 |
| Fig. 14 Morphology analysis of the HA-EDA–PHEA-DVS–GO hybrid nanogels: (A) SEM micrograph of the dried sample and (B) AFM image of the swollen sample. Reproduced from ref. 168 with permission (2017) of the American Chemical Society. | |
4.2. Hybrid nanogels with a core–shell structure
The core–shell (york–shell) structured hybrid nanogels have been of great interest in the biomedical field.22,23,299,300 Typically, core–shell structured hybrid nanogels are synthesized using the core template method with the polymer gel shell deposited onto the core NPs by covalent bonding or adsorption relying on other secondary forces.301,302 The core and shell can provide different multifunctions as well as synergistic effects in biomedical applications, depending on the design. For example, inorganic metal NP cores may provide binding sites and optical properties, while the nanogel shell cannot only protect the inorganic NPs from aggregation, but also protect and stabilize the loaded drugs and afford functionalities including biocompatibility and targeting. To synthesize core–shell structured carbon-based hybrid nanogels, it is normally difficult to use the small-sized carbon nano-objects (e.g., fullerenes, CDs, GO nanosheets, and CNTs) directly as a core template. Lee's group functionalized GO nanosheets with different amounts of DOX drug molecules to form relatively large GO–DOX conjugate NPs for the purpose of a core template. Then, the crosslinked HA nanogels can be deposited onto the GO–DOX conjugate NP core template to form core–shell structured GO–DOX–HA hybrid nanogels.167 As shown in Fig. 15, it is clear that the GO–DOX conjugate NPs have a larger size (∼20–25 nm) than the GO nanosheets. After coating the GO–DOX conjugate NPs with an HA nanogel shell, the size of the hybrid nanogels increases to about 100 nm. Unfortunately, the regular TEM image on the dried hybrid nanogels did not display a clear core–shell interface, due to the low electron density contrast difference between the core and shell materials.
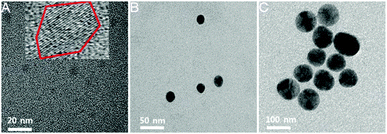 |
| Fig. 15 TEM images of the GO nanosheets (A), GO–DOX conjugate NPs (B), and GO–DOX–HA hybrid nanogels (C), respectively. Reproduced from ref. 167 with permission (2015) of the Royal Society of Chemistry. | |
Recently, a few core–shell structured CD-based hybrid nanogels have been prepared using CD doped inorganic NPs as a core template or mesoporous silica NPs as a core template. The introduction of additional inorganic NPs into the carbon-based hybrid nanogels can provide additional functionalities for biomedical applications. For example, our group synthesized a class of multifunctional hybrid NPs (∼100 nm) that combine magnetic Fe3O4 nanocrystals and fluorescent CDs into a single porous carbon NP (Fe3O4–CDs–PC) using a simple one-pot solvothermal method.59,86 The hydrophilic –COOH and –OH surface functional groups endow excellent water dispersibility to these Fe3O4–CD–PC hybrid NPs, which can thus serve as an excellent core template for the synthesis of CD-based hybrid nanogels. With the Fe3O4–CDs–PC hybrid NPs as a core template, we have successfully deposited a thermo-responsive P(NIPAM–AAm) copolymer nanogel shell onto the core using the precipitation polymerization method. The resultant Fe3O4–CDs–PC@P(NIPAM–AAm) hybrid nanogels not only display a clear core–shell structure (Fig. 16), but also combine the magnetic responsive property with the optical property of the CDs in the core.184
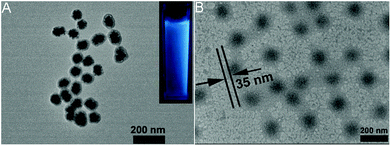 |
| Fig. 16 TEM images of (A) the Fe3O4–CDs–PC hybrid NPs and (B) the Fe3O4–CDs–PC@P(NIPAM–AAm) hybrid nanogels. The inset in (A) is a photograph of the Fe3O4–CDs–PC hybrid NPs exposed under UV light (365 nm). Reproduced from ref. 184 with permission (2014) of the Royal Society of Chemistry. | |
Other widely used core template NPs for the synthesis of core–shell hybrid nanogels are silica NPs. Li et al. synthesized a new core–shell structured CD-based hybrid nanogel for protein sensing.205 They first synthesized CD-doped silica NPs as the core template. The optically transparent silica could not only protect the luminescent CDs, but also provide the core NPs with water dispersibility, biocompatibility and surface functionality. With these CD-doped silica NPs as the core template, they successfully synthesized a new type of core–shell structured CD-based molecularly imprinted polymer (MIP) hybrid nanogel (CDs–SiO2@MIP) through the precipitation polymerization coating of a molecularly imprinted thermo-sensitive P(NIPAM–MAA) nanogel shell onto the CDs–silica NPs. Liu et al. developed a novel method to synthesize CD-based hybrid nanogels.206 Using mesoporous silica NPs as the core template, they performed layer-by-layer (LbL) sequential desposition of poly(N-vinylpyrrolidone) (PVP, Mw = 10 kDa), thiolated PAASH (Mw = 240 kDa with a thiol functionalization degree of 10.3%), CDs and PAASH onto these silica NPs. The subsequent controlled oxidation of thiol groups in the PAASH chains results in the disulfide cross-linking of PAA (Fig. 17A), producing the PVP–PAA–CDs–PAA hybrid nanogel shell coated on the silica NP core template. The TEM image reveals that the mesoporous silica NPs (MSNs) have a uniform, discrete spherical shape with an average diameter of ≈200 nm (Fig. 17B). At higher magnification, the well-ordered mesopores of the silica NPs can be observed (Fig. 17C). In contrast, the core–shell structured CD composite NP (CDCN) hybrid nanogel shows a relatively smooth particle surface, indicating the homogeneous deposition of the LbL multilayer composite nanoshells on the core template (Fig. 17D).
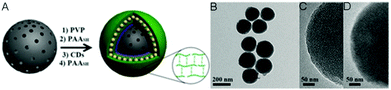 |
| Fig. 17 (A) Schematic illustration of the formation of a CDCN core–shell hybrid nanogel via LbL assembly. (B–D) TEM image of mesoporous silica NPs (B), HRTEM image of silica NP (C), and HRTEM image of the CDCN core–shell hybrid nanogel (D), respectively. Reproduced from ref. 202 with permission (2015) of Wiley. | |
It should be mentioned that the templating method can also be used to synthesize hollow nanogels with a large inner cavity. Typically, the hollow nanogels are synthesized by first the synthesis of core–shell structured nanogels followed by the removal of the core template. So far, a variety of smart hollow nanogels have been synthesized using different core templates, including silica NPs,303,304 Au NPs,305 hydroxypropylcellulose,306 and degradable nanogel.133 It is expected that the hollow nanogels will have larger loading capacity for drug molecules than the nanogels without a cavity. However, to the best of our knowledge, the hollow structured carbon-based hybrid nanogels have not been studied.
5. Biomedical applications of carbon-based hybrid nanogels
The possibility of combining the properties of responsive polymer nanogels and carbon nanomaterials into a single hybrid particle widens their applications or enhances a single property level in different areas of nanomedicine, for example, biosensing, bioimaging, on-demand drug release, and hyperthermia therapy. Until now, many carbon-based hybrid nanogels have been designed and explored for biomedical applications, based on different compositions and properties of each building block from both responsive polymer gels and carbon nano-objects. In this section, some example biomedical applications of different carbon-based hybrid nanogels will be discussed, in terms of biosensing, responsive drug delivery, and multifunctional responsive drug carriers combined with biosensing or bioimaging. A comprehensive summary of the biomedical applications of carbon-based hybrid nanogels developed so far is given in Table 2.
Table 2 Biomedical applications of carbon-based hybrid nanogels
Code of hybrid nanogels |
Major component in hybrid nanogels |
Responsive stimuli |
Drug |
Applications |
Ref. |
P(NIPAM–AAm)–FCNP |
FCNPs (or CDs), P(NIPAM–AAm) |
Temperature |
Curcumin |
Temperature sensing, cell imaging, drug delivery |
166
|
NIR light |
P(NIPAM–AAm–VPBA)–CDs |
Imprinted P(NIPAM–AAm–VPBA), CDs |
Glucose molecule |
|
Glucose sensing |
139
|
Temperature |
PEG–chitosan–CDs |
Nonlinear PEG, chitosan, CDs |
pH |
DOX |
pH sensing, bi-photon cell imaging |
162
|
NIR light |
pH/NIR responsive drug delivery |
CDs–SiO2@MIP |
CDs–SiO2, imprinted P(NIPAM–MAA) |
Temperature |
|
Temperature sensing, cytochrome c sensing |
205
|
Cytochrome c |
Fe3O4–CDs–PC@ P(NIPAM–AAm) |
Fe3O4–CDs–PC, P(NIPAM–AAm) |
Temperature, NIR light, magnetic field |
Curcumin |
Temperature sensing, cell imaging, Thermo/NIR/magnetic-responsive drug release, chemotherapy |
184
|
P(VPBA–AAm)–CDs |
P(VPBA–AAm), CDs |
Glucose molecule |
Insulin |
Glucose-/NIR-responsive insulin release, glucose sensing |
307
|
CDs–FPNIPAM |
CDs, PNIPAM, P(fluorescein–MAA) |
Temperature |
|
Temperature sensing |
95
|
FA–p(OrnAA-CDs) |
CDs, pOrnAA, FA |
pH |
FITC-dextran |
Cancer cell imaging, targeted drug release |
122
|
CCHNs |
Chitosan, CDs |
pH, NIR light |
DOX |
pH-Responsive drug release, combined photothermal and chemo therapy, NIR tumor imaging, TPF imaging |
123
|
mPEG–OAL–CDs |
mPEG, oxidized alginate, CDs |
pH |
DOX |
pH-Responsive drug release, cellular imaging, chemo therapy |
124
|
FA–PEG–HA–CDs |
FA, PEG, hyaluronic acid, CDs |
pH |
DOX |
pH-Responsive drug release, cellular targeting, cellular imaging, chemo therapy |
128
|
CA–CD |
Alginate, CDs |
pH |
Garlic extract |
pH-Responsive drug delivery |
125
|
CDCN |
PVP, PAASH, CDs, SiO2 |
Glutathione (GSH) |
|
GSH sensing, cell imaging |
206
|
PEGDA–CDs |
PEGDA, CDs |
pH |
|
Cell microenvironment pH sensing |
129
|
Alg–CMC–Au–CDs |
Au NPs, alginate, carboxymethyl cellulose, CDs |
pH |
|
Surface-enhanced Raman scattering (SERS)-fluorescence dual-mode pH sensing, extracellular pH sensing |
185
|
rGO–PNIPAM |
rGO, PNIPAM, P(NIPAM–MAA) |
White light |
Calcein |
Thermo- and photo-responsive drug release |
149
|
Temperature |
GDH |
GO–DOX, thiolated hyaluronic acid |
pH, GSH, NIR light |
DOX |
pH/GSH/light-responsive drug release, combined photothermal and chemo therapy, NIR tumor imaging, cellular targeting |
167
|
CGN |
Chitosan, PNIPAM, PEGDA, GO |
NIR light |
DOX |
Thermo-/NIR light-responsive drug release, combined photothermal and chemo therapy, cellular imaging |
207
|
Temperature |
P(NIPAM–AA)–salep–GO |
Salep, GO, P(NIPAM–AA) |
pH, temperature |
DOX |
Thermo-/pH-responsive drug delivery, chemo therapy |
171
|
GS@pPBAs |
GO, PVPBA |
Glucose |
|
Glucose sensing |
208
|
AG |
GO, cystamine-conjugated alginate |
pH |
DOX |
pH/redox responsive drug release, chemo therapy |
127
|
GSH |
HA-EDA–PHEA-DVS–GO |
GO, PHEA-DVS, HA-EDA |
NIR light |
Irinotecan |
Chemo therapy, PTT on tumor |
168
|
HA–C60 |
Fullerene, hyaluronic acid |
NIR light |
|
NIR tumor imaging, photodynamic tumor therapy |
209
|
GCDF |
Dimethylmaleic acid-modified glycol chitosan, fullerene |
NIR light, pH |
|
NIR tumor imaging, endosomal pH targeting, photodynamic tumor therapy |
210
|
CS/PNIPAAm@CNT |
Chitosan, PNIPAM, SCNTs, PEGDA |
pH, temperature, NIR light |
DOX |
Thermo-/pH-/NIR light-responsive drug release, combined photothermal and chemo therapy, cellular imaging |
179
|
Gelatin–CNT |
Gelatin, PMAA, CNT |
Electric field |
Diclofenac sodium salt |
Electric-responsive drug release |
169
|
ND–PEI–chitosan |
PEI, chitosan, nanodiamond |
Lysozyme |
Timolol maleate |
Lysozyme responsive drug release in contact lens |
212
|
5.1. Biosensing
Many pathological processes in various tissues and organs are accompanied by a change of biosignal including pH, temperature, ion concentration, redox state, biomolecules and so on.111,308–313 Thus, the development of hybrid nanogel-based probes for signal tracing can contribute to the explanation of intricate biological processes and the development of advanced diagnoses. In general, both the molecular recognition abilities and optical codes are needed for rapid target identification. By integrating molecular recognition and optical coding components, each hybrid nanogel could be considered as a ‘chemical lab’ that detects and analyzes a unique sequence or compound in a complex mixture.
5.1.1. Glucose sensing.
Two PBA-based glucose responsive carbon-based hybrid nanogels have been developed for glucose sensing.139,208 Our group has recently immobilized fluorescent CDs into glucose imprinted P(NIPAM–AAm–VPBA) copolymer nanogels for glucose detection at physiological pH (Fig. 18).139 In order to enhance the selective and sensitive recognition of the target analyte, molecular imprinting, which was established in 1972 by Wulff and Sarhan, is one good approach to generate artificial receptors.314–316 A MIP gel is normally generated by polymerizing pregel molecules in the presence of template analyte molecules. After removing the template molecules, the polymer network will reproduce a cavity with a fixed size and shape.317 Due to a better fit in shape and surface chemistry, these cavities will later preferentially recognize the analyte molecules over structure analogues. In our P(NIPAM–AAm–VPBA)–CDs hybrid nanogels, glucose molecules are selected as template molecules that can bind with VPBA reversibly. At the rationally designed pH value (pH 8.8) of the reaction medium, the glucose molecules and the VPBA monomers form a stable negatively charged glucose–VPBA complex. After the precipitation polymerization of the pregel components, including, NIPAM, AAm–CDs complexes, and glucose–VPBA complexes, the CDs remain binding with AAm units and the glucose molecules remain binding on the PBA moieties in the resulting P(NIPAM–AAm–VPBA)–CDs hybrid nanogels. The glucose–PBA binding is not favorable in neutral water. Thus, the glucose molecules originally binding on the PBA moieties can be freed and diffuse out from the gel network in deionized water. After dialysis removal of these glucose molecules, the glucose-imprinted boron centers will serve as favorable glucose recognition sites and thus provide high selectivity and sensitivity for glucose detection. The results show that such glucose-imprinted poly(NIPAM–AAm–VPBA)–CDs hybrid nanogels undergo a reversible volume phase transition in response to the variation of glucose concentration. The addition of glucose molecules can selectively bind with the PBA moieties to form a negatively charged mono-dentate complex, thus increasing the ionization degree and inducing gel swelling. The swollen gel network chains produce stress on the surface of the immobilized CDs and quench the fluorescence intensity of CDs, hence converting the biochemical signals into fluorescence signals with excellent reproducibility (Fig. 19).
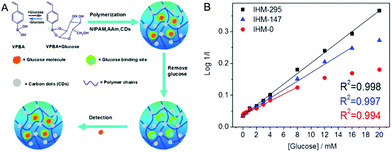 |
| Fig. 18 (A) Schematic synthetic illustration of the glucose-imprinted P(NIPAM–AAm–VPBA)–CDs hybrid nanogels with specific glucose-binding sites for highly sensitive and selective glucose detection. (B) The linear fitting plots of the glucose dependent PL quenching in terms of log(1/I) versus glucose concentration. Reproduced from ref. 139 with permission (2015) of the American Chemical Society. | |
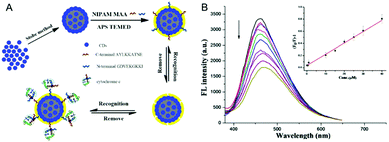 |
| Fig. 19 (A) Schematic illustration of the molecular imprinting of a CDs–SiO2@MIP core–shell hybrid nanogel for highly sensitive and selective detection of cyto c. (B) The normalized fluorescence spectra of CDs–SiO2@MIP hybrid nanogels with the addition of the indicated concentrations of cyto c. Inset is the linear plot of (F0/F) − 1 against the concentration of cyto c. Reproduced from ref. 205 with the permission (2015) of Elsevier. | |
Zhou et al. developed a selectively glucose-responsive biosensor based on the GO–P(4-VPBA) hybrid nanogels, which enables “turn-on” photoluminescence detection of glucose in blood serum.208 In this system, the GO serves as the optical code and the PBA moieties are still the glucose-recognizing targets. Interestingly, the increase in glucose concentration enhances the fluorescence intensity of the hybrid nanogels, which is opposite to the optical signal response observed in the P(NIPAM–AAM–VPBA)–CDs hybrid nanogels. Moreover, the GO–P(4-VPBA) hybrid nanogels displayed excellent selectivity to glucose over other monosaccharides or even polysaccharides/glycoproteins, although no molecular imprinting process was adopted. The reason is that the GO–P(4-VPBA) hybrid nanogels are composed of a large amount of boronates (major component), which would react with both 1,2-cis- and 5,6-cis-diols of glucose to form glucose–bis(boronate) complexes. The formation of such glucose–bis(boronate) complexes creates additional cross links within the gel networks and, thus, provokes shrinkage upon adding glucose. The shrinkage of the gel network chains reduces the surface stress produced from the stretching polymer network chains on the GO sheets, and thus enhance the fluorescence intensity. In contrast, the hybrid nanogels containing a relatively small amount of boronates (e.g., <15 mol%) will only bind with glucose to form negatively charged mono-dentate complexes, thus inducing the gel swelling and causing the fluorescence quenching of the carbon nano-objects in the hybrid nanogels.
5.1.2. Cytochrome complex sensing.
Li et al. developed a new core–shell structured molecularly imprinted CDs–SiO2@MIP hybrid nanogel for highly sensitive and selective detection of cytochrome c (cyto c). In this hybrid nanogel system, the CDs buried in SiO2 NPs serve as the fluorescence code and the peptide imprinted nanogel shell provides the recognition ability towards the cyto c molecules.205 The N- and C-terminal nonapeptides of cyto c were chosen as the common template when synthesizing the P(NIPAM–MAA) nanogel shell. The thermo-sensitive PNIPAM element allows the reversible swelling and shrinking in response to temperature changes to realize recognition and release of target protein cyto c. The resultant CDs–SiO2@MIP hybrid nanogel could recognize the target cyto c with a linear fluorescence quenching via the special interaction between the cyto c molecules and the recognition cavities, over a concentration range of 0.1–40 μM of cyto c. The detection limit was determined to be 89 nM. Such a high sensitivity is attributed to the high imprinting factor of the double templates of N- and C-terminal nonapeptides on the nanogel shell with the following reasons: (1) N- and C-terminal nonapeptides were chosen as the common template, and they could be preassembled with NIPAM and MAA via double hydrogen bond interaction effects; (2) the two-terminals imprinting strategy allowed the selective rebinding of two terminals of the targets into the cavities of CDs–SiO2@MIP, respectively. When N- or C-terminal nonapeptide was used to imprint the nanogel shell, the detection sensitivity of the hybrid nanogel for cyto c is lowered.
5.1.3. Intracellular redox state (or GSH) sensing.
The intracellular redox state serves as a significant regulator of cell microenvironmental reactions.308 The alternation of intracellular redox state can affect signal transduction, protein synthesis, DNA and RNA synthesis, enzyme activation and even regulation of the cell cycles.309 It is known that glutathione (GSH) is considered to be the major contributing molecule to the intracellular redox state.318–320 Therefore, detection of the GSH level has been used to estimate the intracellular redox state in the past decades.321
Liu et al. have developed novel and sensitive CDCNs for detection of the GSH level, which could be utilized to evaluate the intracellular redox state.206 The CDCNs composed of a silica NP core and a disulfide cross-linked PVP–PAA–CD multilayer nanogel shell exhibit extremely weak luminescence due to the luminescent quenching effect caused by the aggregation of CDs. However, upon the addition of a certain amount of GSH, the PL intensity of the CDCNs can be significantly enhanced due to the deconstruction of the nanogel shell through the GSH triggered cleavage of disulfide linkages and subsequent release of the assembled CDs (Fig. 20A and B). Meanwhile, through tuning the cross-linking density of CDCNs (thiol functionalization degree of the polymer shell), the responsive concentration of GSH varied, making it available for detecting intracellular GSH concentrations in various cells (Fig. 20C and D). For example, CDCN-10 hybrid nanogels composed of a thiol functionalization degree of 10.3% on the PAA chains in the nanogel shell possess a responsive GSH concentration of 10 × 10−3 M. Importantly, the encapsulation of the rhodamine B (RhB) dye in the mesoporous silica core NP of the hybrid nanogels could not only monitor the intracellular deconstruction process of RhB-loaded CDCNs (RCDCNs) in real time, but also construct a ratiometric nanosensor for GSH concentration measurement. This new approach for detection of GSH concentrations in cancer cells and normal cells has a great significance on cancer diagnostics and therapy.
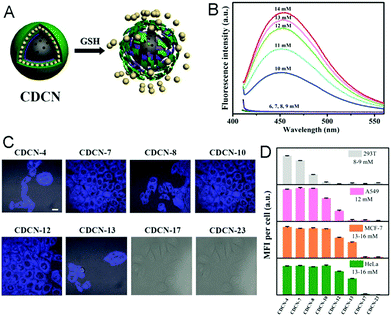 |
| Fig. 20 (A) Schematic illustration of the deconstruction of CDCN and release of CDs in the presence of GSH. (B) GSH concentration dependent PL emission spectra of CDCN-10 hybrid nanogels in buffer (pH 7.4, 0.15 M NaCl) solution at 37 °C for 24 h. (C) The overlay of bright-field and fluorescent images of HeLa cells after 24 h incubation with CDCN-4, CDCN-7, CDCN-8, CDCN-10, CDCN-12, CDCN-13, CDCN-17, and CDCN-23, respectively. The scale bar is 25 μm and corresponds to all images. (D) Mean fluorescence intensity per cell of 293T cells, A549 cells, MCF-7 cells, and HeLa cells incubated with various CDCNs for 24 h. Reproduced from ref. 206 with the permission (2015) of Wiley. | |
5.1.4. pH sensing in cell microenvironments.
Chandra and Singh recently fabricated a type of poly(ethylene glycol)diacrylate–CD (PEGDA–CD) hybrid nanogel using pH-sensitive CDs bearing –COOH, –OH, and –NH2 for cell microenvironmental pH sensing.129 In this PEGDA–CD hybrid nanogel system, the polymer is neutral, but the CDs serve as both the optical code and recognition elements to the proton ions. Since the surface of the CDs has many ionizable functional groups that are vulnerable to pH changes, they can ultimately affect the surface states and give rise to pH sensitive fluorescence emissions. After being immobilized into the PEGDA nanogels, the fluorescence of the CDs is still very stable and pH-sensitive, even under the high ionic strength of 1 M (well above the physiological ionic strength of ∼0.13 M). The hybrid nanogels incubated in PBS of different pH values can be imaged using a fluorescence microscope. The results indicate that the green fluorescence intensity of the PEGDA–CDs hybrid nanogels increases as the pH of the solution decreased (Fig. 21A). The average fluorescence intensity from the hybrid nanogels, calculated using ImageJ software, when plotted against pH values, showed a linear relationship between fluorescence emission and pH (Fig. 21B), indicating the suitability of the PEGDA–CDs hybrid nanogels for pH sensing. To probe the pH change in a cell microenvironment, mammalian cells are fabricated into the hybrid nanogels using a microfluidic method. The results demonstrate that the decrease in pH as the mammalian cells grow inside the biocompatible PEGDA–CD hybrid nanogels can be easily monitored via fluorescence microscopy. These results suggest that the newly developed PEGDA–CD hybrid nanogels can be used to study time dependent changes in cellular microenvironment pH and monitor the cellular growth or disease progression.
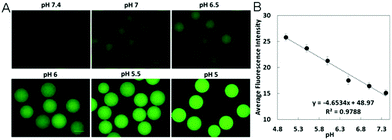 |
| Fig. 21 (A) Green emission (λex = 480 nm) from the PEGDA–CDs hybrid nanogels dispersed in solution with different pH values. Image shows increased fluorescence as the pH decreases from 7.4 to 5. Scale bar is 200 μm. (B) Plot showing change in average fluorescence intensity per nanogel against the respective pH values. Reproduced from ref. 129 with permission (2017) of the American Chemical Society. | |
Yue et al. developed another type of alginate–CMC–Au–CD hybrid nanogel for extracellular pH sensing.185 In this system, the pH-sensitive alginate and carboxymethyl cellulose gel network chains serve as the recognition elements for proton ions. Interestingly, both the gold NPs and CDs serve as the optical codes for pH sensing. Using the versatile microfluidic fabrication method, the Au NPs and CDs can be distributed evenly in each half of the nanogel spheres, which enables the surface-enhanced Raman scattering (SERS) and fluorescence dual-mode extracellular pH sensing.
5.2. Responsive drug delivery
Carbon-based hybrid nanogels are ideal candidates for highly effective stimuli-responsive drug delivery carriers with super-high drug loading capacities, because of their porous structure, large surface area and favorable drug interactions of carbon nano-objects, and high solution stability. So far, the external stimuli, mainly composed of endogenous and exogenous activations, have been widely used to control the release behavior of drugs for disease treatment in vivo.116,322
5.2.1. Endogenous activation-responsive drug delivery.
The endogenous activation can be realized by the variation of specific physiochemical characteristics of the pathological microenvironment including pH, temperature, biomolecules and so on.323 Several carbon-based hybrid nanogels have been developed for responsive drug delivery. Majumdar et al. fabricated a type of alginate–CD hybrid nanogel for pH-responsive drug delivery systems that can release drugs depending upon the amount of pathogen (MRSA) present in the target.125 Interestingly, the incorporation of CDs onto the alginate nanogels dramatically enhances the loading capacity from 19% to 78% for the garlic extract model drug containing allicin. The alginate–CD hybrid nanogels display pH-dependent controlled drug release, which results in increased therapeutic efficiency. More importantly, the hybrid nanogels are not only stimuli responsive but also a controlled drug release system as it releases the drug according to the pathogen concentration (MRSA), because cell divisions produce secondary metabolites that lead to the decrease in pH of the medium. The drop in the pH value triggers drug release from the hybrid nanogels, resulting in MRSA cell death. Bardajee et al. developed a new class of thermo and pH dual-responsive biodegradable hybrid nanogels from the copolymerization and crosslinking of NIPAM and AA monomers in the presence of salep modified GO.171 The resultant P(NIPAM–AA)–salep–GO hybrid nanogels display high loading capacity for DOX drug and thermo/pH dependent releasing behavior: slow drug release at neutral pH and lower temperature but increased significantly in acidic pH and higher temperature, without any burst release. While the GO-based hybrid nanogels exhibited no cytotoxicity in the tested concentration range up to 410 μg mL−1, the dual responsive sustained drug release from the hybrid nanogels enhanced toxicity to HeLa cells when compared to the equivalent dose of the free drug.
Kim et al. reported a rational design of therapeutic contact lenses through the incorporation of NDs into enzyme responsive nanogel matrices as effective ocular drug delivery platforms (Fig. 12).212 The timolol maleate-loaded ND–PEI–chitosan hybrid nanogel could be embedded within a hydrogel matrix and cast into contact lenses. The ND–PEI–chitosan hybrid nanogel can retain the timolol by short distance chemical interactions to avoid the premature elution of the drug, but keeping it physically active for its further release under degradation of the chitosan-based nanogel via lysozyme cleavage. In addition to the improved timolol-controlled release, the incorporation of a small amount of NDs into the lens matrix enhances the tensile strength and boosts the elastic modulus by the reinforcement of the polymer matrix. Overall, the obtained contact lens embedded with lysozyme degradable and timolol-loaded ND–PEI–chitosan hybrid nanogel not only had excellent water and oxygen permeability, optical clarity, and improved mechanical properties, but also most importantly displayed sustainable drug release under lysozyme activation. This novel ND-based system provided an interesting platform for the development of advanced enzyme triggered drug release devices for sustained therapy applications.
5.2.2. Exogenous activation-responsive drug delivery.
The exogenous activation of drug release has attracted considerable attention because it does not rely on changes in specific physical or chemical properties of the environmental medium, which may cause severe side-effects to normal cells and tissues in in vivo applications due to a harsh environmental change.324,325 A promising exogenous activation strategy is to apply electric field/magnetic field/NIR light, which can trigger the drug release from hybrid nanogel carriers at the desired site and time and thus enhance site-specific accumulation. Such a spatial or temporal control is expected to significantly improve therapeutic efficacy in cancer treatment. Lu et al. incorporated the rGO nanosheets into the thermos-sensitive PNIPAM nanogel, producing a photo-/temperature dual responsive drug delivery system.149 The content of rGO in the hybrid nanogels can modulate the thermal and photic sensitivity. With a lower rGO content (<47.5 wt%), the rGO–PNIPAM hybrid nanogels exhibit a temperature responsive drug release with more drug release as the temperature increases. However, with a higher rGO content (>64.5 wt%), the hybrid nanogels have no thermos-responsive drug release, and instead, display photo-responsive drug release. Spizzirri et al. developed a biocompatible gelatin–CNT hybrid nanogel, which demonstrated electric field responsive drug release.169 The stimulation of an electric field (9 V) on the hybrid nanogel system can increase the drug release by 20%. The hybrid nanogels containing a higher contents of CNTs release more drug molecules in response to the stimulus of the same electric field strength. Such electro-responsive CNT-based hybrid nanogels could be inserted into a suitable topical drug delivery device in order to release therapeutics as a consequence of an applied external voltage.
5.2.3. Combined endogenous/exogenous activation-responsive drug delivery.
The combination of endogenous and exogenous activation-responsive drug delivery is a desirable approach to further improve the therapeutic efficacy of cancer in a synergistic manner.116 Such combined therapy is expected to lower the drug dosage requirements while still achieving comparable cytotoxicity to the corresponding drug dose without exogenous activation, thus minimizing systemic side-effects of chemotherapeutic agents. Xu et al. developed a type of GO hybridized biodegradable alginate nanogel that exhibits combined anticancer therapeutic effects from both endogenous and exogenous activations.127 The DOX-encapsulated AGD hybrid nanogels maintained a limited DOX drug release under normal physiological conditions, but released DOX drug in an accelerated way under both acidic and reducible conditions mimicking extracellular tumor microenvironments and intracellular compartments. The dual-stimulative release properties of the GO-based hybrid nanogels enable an effective delivery of anticancer drug in tumor cells and high intracellular drug accumulation for a long time, exerting a good antitumor cytotoxicity, which can be further enhanced under NIR laser photothermal treatment. These GO-based hybrid nanogels provide a promising potential for a new combinative anticancer treatment. In another example, pH and NIR light responsive HA-EDA–PHEA-DVS–GO double-network hybrid nanogels could also combine the endogenous and exogenous activation responsive drug delivery for treatment of colorectal carcinoma cells.168 Thanks to the huge aromatic surface area of GO, the hybrid nanogels can easily load a high amount of the poorly water-soluble anticancer drug irinotecan (33.0 wt%). Both the pH of the external medium and the NIR irradiation process can control the release of the antitumor drug. The irinotecan-loaded GO-based hybrid nanogels can easily enter into cancer cells and synergize the hyperthermic and drug cytotoxic effects in the presence of NIR irradiation, providing high therapeutic efficacy with combined photothermal and chemotherapy.
Qin et al. prepared another type of carbon-based CS/PNIPAM@CNT hybrid nanogel with chitosan-coated CNTs randomly embedded in the PNIPAM–PEG nanogels that can also combine the endogenous and exogenous activation responsive drug release to enhance the anti-cancer therapeutic efficacy (Fig. 22A).179 The pH/thermo dual responsive CS/PNIPAm@CNT hybrid nanogels released DOX faster at 40 °C than at 25 °C (same pHs) and faster at pH 5.0 than at pH 7.4 (same temperatures) (Fig. 22B). Moreover, the DOX-loaded CS/PNIPAm@CNT hybrid nanogels upon NIR irradiation showed significantly greater cytotoxicity in HeLa cells owing to a NIR-triggered increase in temperature and enhanced DOX release (Fig. 22C). More examples of the improved therapeutic efficacy of the carbon-based hybrid nanogels through the combination of the endogenous and exogenous activation responsive drug delivery are summarized in the next section for multifunctional applications.123,162,166,167,184,207
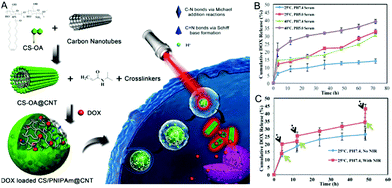 |
| Fig. 22 (A) Schematic representation of DOX loaded CS/PNIPAM@CNT hybrid nanogels and the pH-/NIR light responsive drug release in cells. (B) Temperature/pH-responsive DOX release and (C) NIR-responsive DOX release from the DOX-loaded CS/PNIPAM@CNT hybrid nanogels. Reproduced from ref. 179 with permission (2015) of Elsevier. | |
5.3. Multifunctional applications with combined biosensing, bioimaging and responsive drug delivery
Current treatments of diseases typically require a first diagnosis followed by application of a known therapeutic method. This process is time consuming and often associated with severe side effects due to the lack of site targeting ability, orthogonal treatment, or real time monitoring of the treatment progress to adjust drug dose. For example, although much progress has been achieved for cancer treatment by chemotherapy, patients treated by conventional chemotherapy commonly suffer from severe side effects. The combination of medical therapy with biosensing and/or bioimaging, holds great promise for the investigation and treatment of various diseases with reduced side effects because they can potentially provide simultaneous diagnosis, therapy, and monitoring of the response to treatment. This section will exemplify the multifunctional applications of carbon-based hybrid nanogel drug carriers.
5.3.1. Biosensing combined with responsive drug release.
We recently reported a P(VPBA–AAm)–CD hybrid nanogel that can realize the simultaneous optical detection of glucose using NIR light and glucose responsive insulin delivery.307 The reversible glucose-responsive swelling/deswelling transition of the P(VPBA–AAm) network chains could change the surface states of the hydrogen bonded CDs embedded in the networks and thus affect the fluorescence properties of the hybrid nanogels (Fig. 23A). The glucose-induced PL intensity change exhibits a linear correlation to the increase in the glucose concentration in a clinically relevant range at physiological pH (Fig. 23B). As glucose molecules are removed from the hybrid nanogels, the dissociation equilibrium of the PBA groups on the nanogel network chains shifts back from boronate ester to boronic acid and thus the nanogels reversibly shrink back. The return of the environmental nature surrounding the CDs embedded in the nanogel networks leads to a recovery of the PL spectra (Fig. 23C). The porous structure of the P(VPBA–AAm)–CD hybrid nanogels provides a high loading capacity for insulin molecules and the insulin release rate from the hybrid nanogels can be triggered by the glucose concentration in the dispersion medium (Fig. 23D).
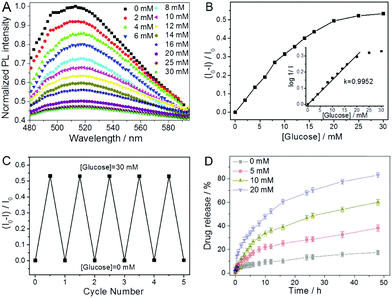 |
| Fig. 23 (A) PL spectra and (B) the quenched PL intensity of the P(VPBA–AAm)–CDs hybrid nanogels as a function of glucose concentration. The inset in (B) is the linear fitting plot of the glucose dependent PL quenching in terms of log(1/I) versus glucose concentration. (C) Reversible PL decay and recovery cycles upon the repeated addition (30.0 mM) and dialysis removal of glucose (0 mM) in the dispersion medium of the hybrid nanogels. All measurements were performed in PBS of pH = 7.4. Excitation wavelength = 900 nm. (D) Release profiles of insulin from the hybrid nanogels dispersed in PBS of pH = 7.4 at different glucose concentrations (0 mM, 5 mM, 10 mM and 20 mM) and a constant temperature of 37 °C. Reproduced from ref. 307 with permission (2016) from the Royal Society of Chemistry. | |
In general, two factors should be responsible for the glucose-induced PL quenching of the hybrid nanogels. First, the swelling/deswelling of the nanogel networks will change the local refractive index of the surrounding medium of the CDs and thus change the Rayleigh scattering intensity.326,327 Second, the swelling/deswelling of the nanogel networks can change the number of surface defects on the CDs that are immobilized in the polymer networks.
5.3.2. Bioimaging combined with responsive drug release.
The fluorescence optical imaging ability of carbon-based hybrid nanogels can be provided by the quasi semi-conducting nanocarbons (like CDs, graphene or SWCNTs).328 Its popularity comes largely from the fact that nanocarbons utilize a nonionizing portion of the electromagnetic spectrum and thus provide a safety profile that is unattainable by other popular imaging modalities such as X-ray and conventional CT that utilize ionizing radiation. The FOI in the NIR light wavelength range is particularly favorable for disease detection and analysis of functional biomolecules in vivo,329,330 because the wavelength window of NIR imaging falls in the regions of low energy absorption by water, oxyhemoglobin, and deoxyhemoglobin and thus provides deep penetration into tissues. Benefiting from the strong absorbance and fluorescence emission in the NIR region, the CDs/fullerenes/CNT-based hybrid nanogels have widely been used to combine the bioimaging function with responsive drug delivery for therapeutic purposes.123,209,331 The combined application containing bioimaging function and responsive drug delivery for chemotherapy capability have great advantages over traditional chemotherapy because the imaging function can help to precisely identify the location of the tumor region and monitor the treatment progress.
One example is the chitosan@CD hybrid nanogels developed in our group with many CDs randomly embedded in the chitosan nanogels (Fig. 24A), which provides the hybrid nanogels with enhanced drug loading capacity, bright fluorescence for multimodal imaging, and excellent photothermal conversion ability.123 The chitosan@CDs hybrid nanogels can enter into cells and light up the cells in multicolor form due to the excitation-wavelength tunable fluorescence emission of CDs embedded in the hybrid nanogels (Fig. 24B–D). In addition, no significant autofluorescence was observed on cell imaging even being irradiated up to 30 min under a strong laser due to the excellent photostability of CDs. Thanks to the upconverted fluorescence property of CDs, the CDs-based hybrid nanogels could serve as an excellent two-photon imaging contrast agent under the excitation of NIR light (Fig. 24E), which demonstrates minimal photodamage and deep tissue penetration in tissues. The DOX-loaded chitosan@CD hybrid nanogels demonstrated intelligent drug release in response to both NIR light and change in pH (Fig. 24F and G) and could permeate into implanted tumors on mice and release drug molecules efficiently on site to inhibit tumor growth. More importantly, the NIR imaging function of the chitosan@CD hybrid nanogels could be used to monitor the therapeutic effect of cancer by imaging-guided chemotherapy in vivo (Fig. 24H). The therapeutic outcome achieved by chemo-photothermal combinatory therapy is much better than the additive result from separated photothermal therapy and chemotherapy based on the tumor growth-reduction ratio (Fig. 24I and J), suggesting a synergistic effect by the combinatory chemo-photothermal therapy.
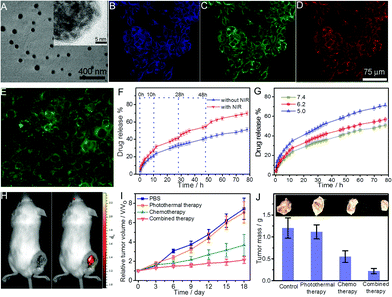 |
| Fig. 24 (A) TEM image of chitosan@CD hybrid nanogels with the inset showing the magnified section of the hybrid nanogel. (B–D) Confocal images of the 4T1 cells treated with chitosan@CDs hybrid nanogels excited by different lasers of (B) 405, (C) 488, and (D) 546 nm. (E) TPF imaging of the 4T1 cells treated with chitosan@CDs hybrid nanogels excited by an NIR laser of 820 nm. (F) DOX-release kinetics from the chitosan@CD hybrid nanogels in PBS (pH = 7.4) at 37 °C under 5 min exposure to NIR irradiation (808 nm, 1.5 W cm−2) at the indicated time points. (G) pH-responsive DOX-release from the chitosan@CD hybrid nanogels in buffers of different pH values at 37 °C. (H) NIR image of the tumor site from a mouse intravenously treated with DOX-loaded chitosan@CD hybrid nanogels at day 18 post-treatment. (I) Tumor growth curves of mice after receiving different treatments including control, photothermal therapy, chemotherapy, and combined photothermal-chemo therapy. (J) Mass and pictures of tumors obtained from mice on day 18 after receiving different treatments. Reproduced from ref. 203 with permission (2017) of the American Chemical Society. | |
Liu's group developed two series of biocompatible polysaccharide-based hybrid nanogels with fluorescent CDs being used as crosslinkers for theranostic applications.124,128 The resultant mPEG–OAL–CDs and FA–PEG–HA–CDs hybrid nanogels with highly integrated CDs in the nanogel networks could be used for imaging-guided drug delivery in the tumor therapy. The theranostic hybrid nanogels demonstrated extraordinary fluorescence property, and therefore, provide great potential for the real-time and noninvasive location tracking to cancer cells based on the FOI function. Furthermore, the pH-triggered site-specific release and tumor-targeted delivery of DOX from the two types of hybrid nanogels in the mimicked tumor microenvironment enhanced the anticancer efficiency of DOX, with less drug leakage in the simulated physiological media. Another example is the zwitterionic FA–p(OrnAA-CDs) hybrid nanogel that can be used for targeted drug delivery and simultaneous bioimaging.122 The CD crosslinked hybrid nanogels not only exhibit a controlled drug release manner, but also provide stable fluorescence signals for real-time tracking in the drug delivery process. Furthermore, the FA-modified hybrid nanogels can selectively target the cancer cells overexpressed with folate receptor.
Wang et al. developed a thermo- and NIR light dual responsive CGN hybrid nanogel drug delivery platform based on incorporation of chitosan-modified rGO into a thermosensitive nanogel.207 The CGN not only shows a high DOX loading capacity (48 wt%), but also displays a temperature and NIR-light dual responsive drug release behavior in the physiologically important temperature range (37–42 °C). Upon irradiation with NIR light (808 nm), the CGN hybrid nanogels can release DOX drug rapidly and repetitively, demonstrating a promising application for on-demand drug release by NIR light. More importantly, the fluorescence imaging ability of the CGN can monitor the drug expression location in the cancer cells at different temperatures. Furthermore, the CGN can combine the NIR photothermal therapy with the DOX chemotherapy and significantly enhance the therapeutic efficacy to kill cancer cells.
The GO–DOX–HA (GDH) hybrid nanogels developed by Lee's group could even combine more effects into a single hybrid nanogel drug carrier through the incorporation of NIR light responsive DOX-conjugated GO nanosheets into the pH and GSH dual sensitive disulfide-crosslinked HA nanogel.167 For example, the GDH hybrid nanogels demonstrated excellent NIR imaging function both in vitro and in vivo (Fig. 25A–D). Such NIR fluorescence images of tumor-bearing mice treated with the GDH hybrid nanogel could be used to verify the size and location of the tumor because the nanogel preferentially accumulates in tumors passively and actively through both an EPR effect and guidance of the receptor-mediated HA receptor (Fig. 25D). The GDH hybrid nanogels also display pH, GSH, and NIR light responsive controlled drug release behavior. In addition, the fluorescent GO in the GDH nanogel can be considered an optical imaging contrast agent as well as a heat source when excited by laser irradiation. Thus, the hybrid nanogel can synergize the NIR photothermal treatment with the DOX treatment in killing tumor cells, demonstrating a much higher therapeutic efficacy compared to other treatments (Fig. 25E). Additionally, the change in the body weight of treated nude mice proved insignificant and their vital behaviors appeared equivalent to the mice receiving other types of treatments (Fig. 25F).
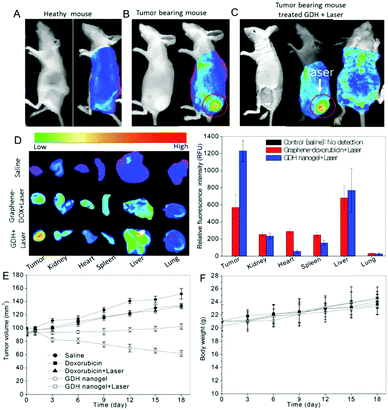 |
| Fig. 25
In vivo optical imaging and combined thermo-chemotherapy using the GDH hybrid nanogel. (A and B) Optical imaging of healthy mice and tumor bearing mice, respectively. (C) Light responsive imaging. The GDH nanogels were intravenously injected and a 670 nm laser was applied to the tumors for 30 min. (D) Ex vivo imaging and fluorescence intensities of tumors and normal tissues. Organs were arranged in the following order: tumor (T), kidney (K), heart (H), spleen (S), liver (Li), and lung (Lu). (E) Tumor volume change after being treated with DOX and the GDH hybrid nanogels with and without laser irradiation. (F) Body weight changes in mice after treatment with the nanogels. The data were plotted as mean ± SEM (n = 5). Reproduced from ref. 167 with permission (2015) of the Royal Society of Chemistry. | |
5.3.3. Bioimaging combined with PDT/PTT.
PDT/PTT employs photosensitizing agents taken up by cells to generate highly reactive oxygen species or heat from light absorption respectively, leading to photoablation of the cancer cells and subsequent cell death.332–334 To avoid nonspecific heating of healthy cells, photosensitizers must show high absorption in the NIR region and selective uptake in cancerous cells in tumors over normal cells in healthy tissues. Until now, some carbon-based hybrid nanogels have been used as photosensitizing agents for PDT/PTT due to the strong NIR absorbance of nanocarbons in the NIR region.167,168,209,210 For example, the HA–C60 conjugates allowed high-resolution NIR fluorescence imaging of tumor sites in vivo without any label from fluorophores or isotopes.209 The HA–C60 conjugates can effectively accumulate into the tumor tissue, primarily owing to passive targeting via the EPR effect by their prolonged circulation in the blood stream and active targeting via specific binding to the CD44 receptor on tumor cells. Therefore, the HA–C60 conjugates systemically administered into the tumor-bearing nude mice demonstrated a high PDT efficacy when NIR light was applied on the solid tumor site after 24 h post-injection.
Kim et al. also developed a glycol chitosan–dimethylmaleic acid–fullerene (GCDF) hybrid nanogel for combined NIR imaging with PDT upon NIR light illumination.210 The fluorescence intensity of the GCDF hybrid nanogels containing different amounts of C60 was visualized with a Kodak image station (Fig. 26A). Furthermore, the GCDF injected intravenously through the tail vein into KB tumor-bearing nude mice can serve as a fluorescence label for in vivo NIR fluorescence imaging (Fig. 26B and C). The GCDF hybrid nanogels provided high-resolution fluorescence intensity at the tumor site after 8 h post-injection, which could be attributed to the extravasation of hybrid nanogels from the tumor vasculature due to the EPR effect. The C60-based GCF and GCDF hybrid nanogels demonstrated high therapeutic efficacy based on the NIR light PDT because of the effective generation of singlet oxygen species (Fig. 26D). In contrast, the same hybrid nanogels have no cytotoxicity to the KB cells without application of NIR light even at much higher doses (Fig. 26E).
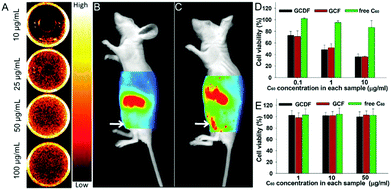 |
| Fig. 26 (A) NIR fluorescence (excitation wavelength = 635 nm, emission wavelength = 720 nm) images from wells containing GCDF hybrid nanogels (equivalent to 10–100 mg mL−1 of C60) in 150 mM PBS (pH 7.4). (B and C) In vivo non-invasive fluorescence imaging of nude mice harboring KB tumors. A GCDF (equivalent to 10 mg C60 per kg mice body) was intravenously injected into nude mice and fluorescence images were obtained at 2 h (B, left) or 8 h (C, right) post-injection. The tumor site is indicated by a white arrow. (D and E) Cell viabilities of KB cells treated with GCDF, GCF nanogels and free C60 (n = 7) at relatively low C60 concentration (0.1–10 mg mL−1 C60) for 12 h with light illumination (D) and at relatively high C60 concentration (1–50 mg mL−1 C60) for 24 h without light illumination (E). Reproduced from ref. 210 with permission (2013) of the Elsevier. | |
5.3.4. Combined biosensing, bioimaging, and responsive drug delivery.
Our group has developed several CD-based hybrid nanogels that can combine multiple functions including optical sensing, bioimaging and responsive drug release for cancer treatment.162,166,184 For example, the biocompatible PEG–chitosan@CD hybrid nanogels can not only be used as a fluorescent pH sensor to probe the physiological environment change (pH range: from 2.99 to 8.0, Fig. 27A), but also serve as an excellent laser confocal and TPF imaging contrast agent (Fig. 27B–F).162 The hybrid nanogels emit a bright fluorescence and can light up DU145 cells. In addition, the confocal images of DU145 cells did not show a fluorescence signal change after a continuous irradiation with the excitation laser at 405 nm for 30 min benefiting from the excellent photostability of the hybrid nanogels (Fig. 27B and C). The chitosan in hybrid nanogels can induce a pH-sensitive swelling/deswelling of the hybrid nanogels, which not only changes the fluorescence intensity of embedded CDs for pH sensing, but also controls the DOX release over the physiologically important pH range of 5.0–7.4. The thermosensitive nonlinear PEG network can promote the DOX release through the local heat produced by the embedded CDs under NIR irradiation. Therefore, the hybrid nanogels exhibit pH- and NIR light dual responsive drug release (Fig. 27G). The in vitro cytotoxicity test results indicate that the DOX-loaded hybrid nanogels demonstrated significantly higher therapeutic efficacy against the DU145 prostate cancer cells through the synergistic effect of combined photothermal and chemotherapy, when compared to the additive therapeutic efficacy of chemo- and photothermal therapy alone (Fig. 27H and I). The additive therapeutic efficacies of independent chemo- and photothermal treatments were estimated using the relation of Tadditive = 100 − (fchemo × fphotothermal) × 100, where f is the fraction of surviving cells after each treatment.
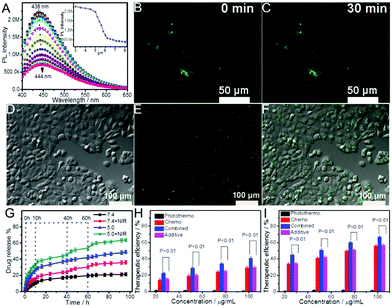 |
| Fig. 27 (A and inset) The pH-dependent PL spectra and relative maximum PL intensity of the PEG–chitosan@CD hybrid nanogels obtained at λex = 360 nm for pH sensing. (B and C) Laser scanning confocal microscopy images of DU145 human prostate cancer cells incubated with the PEG–chitosan@CDs hybrid nanogels (5 μg mL−1) under an excitation wavelength of 405 nm for different exposure times. (D) Transmission, (E) TPF, and (F) overlaid images of DU145 human prostate cancer cells incubated with PEG–chitosan@CD hybrid nanogels. The excitation laser wavelength is 900 nm. (G) The release profiles of DOX from the hybrid nanogels in PBS of pH = 7.4 and 5.0 at 37 °C, respectively, irradiated with/without 1.5 W cm−1 NIR for 5 min at the indicated time points. (H and I) Therapeutic efficacies of PEG–chitosan@CD hybrid nanogels as a drug carrier and photothermal therapy agent at pH = 7.4 (H) and pH = 5.0 (I), respectively, for chemo-, photothermal, and their combined treatments. A t-test was used to compare the therapeutic efficacies of combined treatment with the additive efficacies of chemo- and photothermal treatments alone. All p-values are lower than 0.01. Reproduced from ref. 162 with permission (2015) of Wiley. | |
When the fluorescent carbon NPs (or CDs) were incorporated into the thermo-sensitive P(NIPAM–AAm) nanogels, the resultant hybrid nanogels can combine multiple functions for simultaneous optical temperature sensing, confocal cellular imaging, endogenous thermo-activation and exogenous NIR irradiation-controlled curcumin release by changing the mesh size of the gel networks in response to local temperature changes.166,184 Compared to the corresponding P(NIPAM–AAm) nanogels, the hybrid nanogel drug carrier shows much higher therapeutic efficacy against the mouse melanoma B16F10 cells due the improved drug loading capacity for hydrophobic curcumin molecules and the combined NIR photothermal treatment with chemotherapy. The versatility of the CD-based hybrid nanogels can be further enhanced when a core–shell nanostructure was designed. For example, we have prepared a core–shell hybrid nanogel using the magnetic and fluorescent bifunctional carbon NP (Fe3O4–CDs–PC) as a core and the thermo-responsive P(NIPAM–AAm) nanogel as a shell.184 The resultant Fe3O4–CDs–PC@P(NIPAM–AAm) hybrid nanogels can combine multiple functions for temperature sensing, cell imaging, thermo-/NIR light/magnetic triple responsive curcumin release, and chemotherapy (Fig. 28A). The increase in temperature can deswell the nanogel and enhance the PL intensity of the CDs immobilized in the hybrid nanogel, providing the fluorescent temperature sensing ability (Fig. 28B and C). The core–shell hybrid nanogels can also enter into and light up the B16F10 cells under laser excitation (Fig. 28D). Furthermore, the core–shell hybrid nanogels exhibit thermo-/NIR light/magnetic field triple-stimuli responsive drug release profiles (Fig. 28E–G). Upon NIR-irradiation, the CDs in the hybrid nanogels can convert the NIR light to heat. The increase in temperature can act as a stimulus to activate the temperature-induced shrinking of the P(NIPAM–AAm) gel shell, leading to accelerated drug release. Similarly, upon an external alternating magnetic field, the Fe3O4 nanocrystal in the inner core can generate localized heat which can also induce a phase change in the polymer gel to trigger the release of the loaded curcumin drug. In addition, the magnetic responsive property of the hybrid nanogels may provide a noninvasive site targeting drug delivery ability to reduce the systemic side effects. The in vitro cytotoxic test results indicated that the core–shell hybrid nanogels can combine the NIR photothermal treatment with the curcumin drug treatment and thus significantly enhanced the therapeutic efficacy (Fig. 28H).
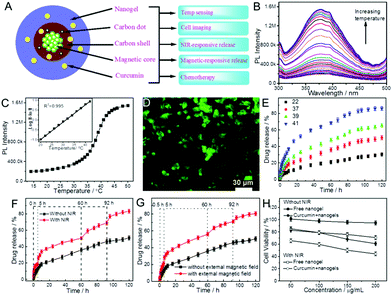 |
| Fig. 28 (A) Schematic illustration of the multifunctional core–shell hybrid nanogel. (B) Typical PL profiles of the hybrid nanogels under different temperatures, taken at 2.0 °C intervals from low to high. (C and inset) The temperature dependent PL intensity of the hybrid nanogels with a linear correlation established over the temperature range of 20–42 °C. (D) Confocal microscopy images of B16F10 cells incubated with the hybrid nanogels under a 20 min continuous exposure to an excitation laser with wavelength = 405 nm. (E) Temperature responsive curcumin release from the core–shell hybrid nanogels. (F) Release profiles of curcumin from the hybrid nanogels at 37 °C with or without 1.5 W cm−2 NIR irradiation for 5 min at the indicated time points. (G) Magnetic field-responsive release profiles of curcumin from the hybrid nanogels at 37 °C with/without application of an external magnetic field for 30 min at the scheduled time points. (H) In vitro cytotoxicity of hybrid nanogels and curcumin-loaded hybrid nanogels without/with 1.5 W cm−2 NIR irradiation for 5 min on B16F10 cells, respectively. Reproduced from ref. 184 with permission (2014) of the Royal Society of Chemistry. | |
6. Conclusions and perspectives
In summary, we have reviewed the preparation methods and structures of different types of carbon-based hybrid nanogels and their applicability in biomedical areas for biosensing, bioimaging, and responsive drug delivery in either alone or combined multifunctions. The carbon-based hybrid nanogels are rapidly emerging as novel nanoplatforms that can not only monitor the biochemical and biophysical parameters in vitro and in vivo, but also deliver drugs intelligently in response to environmental stimuli for combinatorial therapies and imaging-guided therapies to provide multimodal diagnosis, superior therapeutic efficacy, and minimized side effects in different disease treatments. The versatility of the carbon-based hybrid nanogels can be designed from both responsive nanogels and nanocarbons. The combination of nanocarbons with nanogels indeed improves the biocompatibility and biostability of the nanocarbons under physiological conditions, prolongs the nanocarbon circulation time in blood, and improves the penetration across the biological barriers. In addition to the responsive properties of polymer nanogels to the biological endogenous signal change such as acidic pH, increased temperature, increased glucose level, reductive potential, and overexpressed enzymes, the incorporation of nanocarbons into nanogels can add a new exogenous activation process with external stimuli such as NIR light, an electric field, and a magnetic field for diagnosis and therapy. The unique electro-optical properties, highly conjugated carbon frames, and functional surface groups of nanocarbons provide the hybrid nanogels with imaging and sensing functions for real time monitoring of treatment progress, enhanced drug loading capacity, synergistic endogenous/exogenous activation responsive drug delivery, and combinatorial medical therapies with PDT/PTT.
Despite the significant advances that have been achieved, the clinical translation of carbon-based hybrid nanogels for reliable biosensors, smart drug carriers, and theranostics remains to be realized. A number of parameters affect the efficacy of this imaging and delivery system and require further optimization. One is that the current fluorescent wavelength range (400 nm to 950 nm) of nanocarbons limits the sensing and imaging sensitivity of carbon-based hybrid nanogels when used in clinical application. The present imaging range is strongly susceptive to the autofluorescence from endogenous chromophores in the body, which produces unwanted background signal that interferes with the imaging of endogenous tissues and impairs detection sensitivity during functional imaging.335–337 In addition, the intense scattering of short wavelength light by tissues can reduce the penetration depth and detection sensitivity.338,339 Limitations arising from the endogenous autofluorescence, reduced photon adsorption, and light scattering by tissues should be overcome. Novel nanocarbons that have NIR second window imaging ability (1000–1700 nm) need be developed because NIR-II fluorescence provides better contrast and higher spatial resolution than the current fluorescence range.340–342 Doping nanocarbons with other plasmonic or magnetic components into the hybrid nanogels to provide multimodal sensing and imaging ability is a good strategy.184,185 Using the metal-free nanocarbons with T1 positive MRI ability for fabrication of carbon-based hybrid nanogels is another good choice because MRI is a noninvasive, sensitive, and preferred imaging modality in the clinic due to its high spatial resolution and virtually unlimited tissue penetration.343,344
Another issue is low accumulation of hybrid nanogels in solid tumors. A recent survey of nanocarrier studies over the past 10 years indicated that no more than 5% of the administered nanocarriers, including inorganic, organic, and polymer nanoparticles (e.g., nanogels), actually reach the target site.345 The accumulation efficacy of a hybrid nanogel in the tumor site should largely depend on its physicochemical properties, including size, shape, charge, composition and surface properties, which dictate its pharmacokinetics and biodistribution.346–349 For example, both experimental results and theoretical simulations have shown that the shape of a nanocarrier can affect not only its cellular internalization but also biodistribution, circulation, and tumor penetration.350–353 Biodistribution studies demonstrated that particles with high aspect ratios could successfully evade phagocytosis and were endowed with prolonged blood circulation when compared with spherical particles of similar diameter.296,297,354 Hence, nanogels with high aspect ratios are preferred to improve the extravasation into tumors. Application of new engineering techniques such as microfluidic technology and an ultraturrax high pressure homogenizer for fabrication of carbon-based hybrid nanogels with special components and shape is one way to increase the accumulation of nanogels at tumor sites.168,185 The surface conjugation with targeting ligands of the hybrid nanogels is another way to improve the accumulation efficacy of nanocarriers through high specificity towards diseased cells.
The development of carbon-based hybrid nanogels for clinical use is still in its infancy. We believe that the current progress on carbon-based hybrid nanogels will expand the prospects of their applications for non-invasive diagnosis, combinatorial therapies, and theranostics to improve the treatment efficiency of different diseases. However, many preclinical/clinical studies related to safety and efficacy in vivo need to be carried out, including specific binding to various types of diseased cells, biodistributions, metabolism, pharmacodynamics, and pharmacokinetics. The complexity of the system and intricate structural properties demand more fundamental understanding on host–guest interactions and careful engineering of the carbon-based hybrid nanogels in order to achieve the desired effects including the deep penetration and high accumulation on tumors. The scalable production and batch-to-batch reproducibility need to be addressed as well. We hope that this review highlighting the latest development of carbon-based hybrid nanogels, from the broad range of synthetic methods to the wide range of applications in the biomedical field, will inspire more significant studies toward the clinical use of such promising systems.
Abbreviations
1D | One-dimensional |
2D | Two-dimensional |
3D | Three-dimensional |
AA | Acrylic acid |
AAm | Acrylamide |
AC | Alternating current |
AFM | Atomic force microscopy |
BBB | Blood–brain barrier |
BIS |
N,N′-Methylenebisacrylamide |
C60 | Fullerenes |
CD | Carbon dot |
CDCNs | CD composite NPs |
CMC | Carboxymethyl cellulose |
CNT | Carbon nanotube |
CS/PNIPAAm@CNT | Chitosan/poly(N-isopropylacrylamide)/CNT |
CT | Computed tomography |
Cyto c | Cytochrome c |
DEA | 2-(Diethylamino)ethyl methacrylate |
DMA | 2,3-Dimethylmaleic acid |
DMSO | Dimethyl sulfoxide |
DOX | Doxorubicin |
EDC | 1-Ethyl-3-(3-dimethylaminopropyl)carbodiimide |
EDTA | Ethylenediaminetetraacetic acid |
EPR | Enhanced permeability and retention |
FA | Folic acid |
FCNPs | Fluorescent carbon nanoparticles |
FDA | Food and Drug Administration |
Fe3O4 | Iron oxide |
FOI | Fluorescence optical imaging |
GC | Glycol chitosan |
GCDF | Glycol-chitosan–2,3-dimethylmaleic acid–fullerene |
GDH | Graphene oxide–doxorubicin–hyaluronic acid |
GO | Graphene oxide |
GSH | Glutathione |
HA | Hyaluronic acid |
HA-EDA | Hyaluronic-((2-aminoethyl)-carbamate)acid |
HRTEM | High-resolution transmission electron microscopy |
IPNs | Interpenetrating polymer networks |
IUPAC | International Union of Pure and Applied Chemistry |
LbL | Layer-by-layer |
LCST | Lower critical solution temperature |
MEO2MA | 2-(2-Methoxyethoxy)ethyl methacrylate |
MEO5MA | Methyl ether methacrylate |
MIP | Molecularly imprinted polymer |
mPEG-NH2 | Methoxypolyethylene glycol amine |
MRI | Magnetic resonance imaging |
MSNs | Mesoporous silica NPs |
MWCNTs | Multiple-walled carbon nanotubes |
NaMA | Sodium methacrylate |
ND | Nanodiamond |
NHS |
N-Hydroxysuccinimide |
NIR | Near-infrared |
NPs | Nanoparticles |
OAL | Oxidized alginate |
OEG | Oligo(ethylene glycol) |
OrnAA | Ornithine methacrylamide |
PAA | Polyacrylic acid |
PA | Photoacoustic |
PBA | Phenylboronic acid |
PBS | Phosphate buffered saline |
PC | Porous carbon |
PDA | Polydopamine |
PDEA | Poly(2-(diethylamino)ethyl methacrylate) |
PDEGMA | Poly(2-(2-methoxyethoxy)ethyl methacrylate) |
PDT | Photodynamic therapy |
PEG | Polyethyleneglycol |
PEGDA | Poly(ethylene glycol)diacrylate |
PEGDMA | PEGdimethacrylate |
PEI | Polyethylenimine |
PET | Positron-emission tomography |
PHEA-DVS | α,β-Poly(N-2-hydroxyethyl)-D,L-aspartamide-divinyl sulfone |
PL | Photoluminescent |
PNaMA | Poly(sodium methacrylate) |
PNIPAM | Poly(N-isopropylacrylamide) |
P(NIPAM–AA) | Poly(N-isopropylacrylamide-co-acrylic acid) |
P(NIPAM–AAm) | Poly(N-isopropylacrylamide-co-acrylamide) |
P(NIPAM–AAm–VPBA) | Poly(N-isopropylacrylamide-co-acrylamide-co-4-vinylphenyl boronic acid) |
P(NIPAM–MAA) | Poly(NIPAM-co-methacrylic acid) |
PNVCL | Poly(N-vinyl caprolactam) |
pOrnAA | Poly(ornithine methacrylamide) |
PTT | Photothermal therapy |
PVA | Poly(vinyl alcohol) |
PVP | Poly(N-vinylpyrrolidone) |
P(4-VPBA) | Poly(4-vinylphenyl boronic acid) |
P(VPBA–AAm) | Poly(4-vinylphenyl boronic acid-co-acrylamide) |
QDs | Quantum dots |
QYs | Quantum yields |
RCDCNs | RhB-loaded CDCNs |
RhB | Rhodamine B |
rGO | Reduced graphene oxide |
REM | Rare earth metal |
RES | Reticuloendothelial system |
ROS | Reactive oxygen species |
SDS | Sodium dodecyl sulfate |
SEM | Scanning electron microscopy |
SERS | Surface-enhanced Raman scattering |
sIPNs | Semi interpenetrating polymer networks |
SWCNTs | Single-walled carbon nanotubes |
SS | Disulfide bond |
TEM | Transmission electron microscopy |
TPF | Two-photon fluorescence |
UA | Ultrasound |
VIM | Vinylimidazole |
VPTT | Volume phase transition temperature |
VPBA | 4-Vinylphenyl boronic acid |
γ-CDx | γ-Cyclodextrin |
Conflicts of interest
There are no conflicts to declare.
Acknowledgements
We gratefully acknowledge the financial support from the start-up fund of Chinese Academy of Sciences (1600000012B2Q), the American Diabetes Association (Basic Science Award 1-12-BS-243), the PSC-CUNY Research Award (67155-00 45, 60281-00 48), the National Natural Science Foundation (NSFC, 21271163 and U1232211), CAS/SAFEA international partnership program for creative research teams and CAS Hefei Science Center (2016HSC-IU011) and Anhui Province Key Laboratory of Medical Physics and Technology (Grant No. LMPT201701).
References
- M. Hamidi, A. Azadi and P. Rafiei, Adv. Drug Delivery Rev., 2008, 60, 1638–1649 CrossRef CAS PubMed.
- E. M. Ahmed, J. Adv. Res., 2015, 6, 105–121 CrossRef CAS PubMed.
- K. J. De France, T. Hoare and E. D. Cranston, Chem. Mater., 2017, 29, 4609–4631 CrossRef CAS.
- S. Bazban-Shotorbani, E. Dashtimoghadam, A. Karkhaneh, M. M. Hasani-Sadrabadi and K. I. Jacob, Langmuir, 2016, 32, 4996–5003 CrossRef CAS PubMed.
- I. Neamtu, A. G. Rusu, A. Diaconu, L. E. Nita and A. P. Chiriac, Drug Delivery, 2017, 24, 539–557 CrossRef CAS PubMed.
- M. Simphiwe, M. Thashree, E. C. Yahya, K. Pradeep, C. d. T. Lisa and P. Viness, Curr. Pharm. Des., 2015, 21, 2801–2813 CrossRef.
- H. Q. Wu and C. C. Wang, Langmuir, 2016, 32, 6211–6225 CrossRef CAS PubMed.
- R. T. Chacko, J. Ventura, J. Zhuang and S. Thayumanavan, Adv. Drug Delivery Rev., 2012, 64, 836–851 CrossRef CAS PubMed.
- Y. Li, D. Maciel, J. Rodrigues, X. Shi and H. Tomás, Chem. Rev., 2015, 115, 8564–8608 CrossRef CAS PubMed.
- J. Li and D. J. Mooney, Nat. Rev. Mater., 2016, 1, 16071 CrossRef CAS PubMed.
- K. S. Soni, S. S. Desale and T. K. Bronich, J. Controlled Release, 2016, 240, 109–126 CrossRef CAS PubMed.
- F. Alexis, E. Pridgen, L. K. Molnar and O. C. Farokhzad, Mol. Pharmaceutics, 2008, 5, 505–515 CrossRef CAS PubMed.
- C. Gonçalves, P. Pereira and M. Gama, Materials, 2010, 3, 1420 CrossRef.
- N. S. Zarekar, V. J. Lingayat and V. V. Pande, Nanosci. Nanotechnol. Res., 2017, 4, 25–31 CAS.
- S. Mura, J. Nicolas and P. Couvreur, Nat. Mater., 2013, 12, 991–1003 CrossRef CAS PubMed.
- O. Kuksenok, D. Deb, P. Dayal and A. C. Balazs, Annu. Rev. Chem. Biomol. Eng., 2014, 5, 35–54 CrossRef CAS PubMed.
- H. Yang, Q. Wang, S. Huang, A. Xiao, F. Li, L. Gan and X. Yang, ACS Appl. Mater. Interfaces, 2016, 8, 7729–7738 CAS.
- L. Zha, B. Banik and F. Alexis, Soft Matter, 2011, 7, 5908–5916 RSC.
- M. Molina, M. Asadian-Birjand, J. Balach, J. Bergueiro, E. Miceli and M. Calderon, Chem. Soc. Rev., 2015, 44, 6161–6186 RSC.
- B. Sierra-Martin and A. Fernandez-Barbero, Soft Matter, 2015, 11, 8205–8216 RSC.
- W. Wu and S. Zhou, Nano Rev., 2010, 1, 5730 CrossRef PubMed.
- W. Wu, N. Mitra, E. C. Y. Yan and S. Zhou, ACS Nano, 2010, 4, 4831–4839 CrossRef CAS PubMed.
- W. Wu, J. Shen, P. Banerjee and S. Zhou, Biomaterials, 2010, 31, 7555–7566 CrossRef CAS PubMed.
- H. Zhu, Y. Li, R. Qiu, L. Shi, W. Wu and S. Zhou, Biomaterials, 2012, 33, 3058–3069 CrossRef CAS PubMed.
- O. S. Wolfbeis, Chem. Soc. Rev., 2015, 44, 4743–4768 RSC.
- Q. M. Zhang, W. Xu and M. J. Serpe, Angew. Chem., Int. Ed., 2014, 53, 4827–4831 CrossRef CAS PubMed.
- H.-s. Peng, J. A. Stolwijk, L.-N. Sun, J. Wegener and O. S. Wolfbeis, Angew. Chem., Int. Ed., 2010, 49, 4246–4249 CrossRef CAS PubMed.
- K. W. Y. Chan, G. Liu, X. Song, H. Kim, T. Yu, D. R. Arifin, A. A. Gilad, J. Hanes, P. Walczak, P. C. M. van Zijl, J. W. M. Bulte and M. T. McMahon, Nat. Mater., 2013, 12, 268–275 CrossRef CAS PubMed.
- L. Feng, M. Gao, D. Tao, Q. Chen, H. Wang, Z. Dong, M. Chen and Z. Liu, Adv. Funct. Mater., 2016, 26, 2207–2217 CrossRef CAS.
- X.-R. Song, X. Wang, S.-X. Yu, J. Cao, S.-H. Li, J. Li, G. Liu, H.-H. Yang and X. Chen, Adv. Mater., 2015, 27, 3285–3291 CrossRef CAS PubMed.
- A. Gianella, P. A. Jarzyna, V. Mani, S. Ramachandran, C. Calcagno, J. Tang, B. Kann, W. J. R. Dijk, V. L. Thijssen, A. W. Griffioen, G. Storm, Z. A. Fayad and W. J. M. Mulder, ACS Nano, 2011, 5, 4422–4433 CrossRef CAS PubMed.
- P. Haigron, J. L. Dillenseger, L. Luo and J. L. Coatrieux, IEEE Eng. Med. Biol., 2010, 29, 100–104 CrossRef PubMed.
- C.-K. Lim, A. Singh, J. Heo, D. Kim, K. E. Lee, H. Jeon, J. Koh, I.-C. Kwon and S. Kim, Biomaterials, 2013, 34, 6846–6852 CrossRef CAS PubMed.
- Y. Li and S. Zhou, Chem. Commun., 2013, 49, 5553–5555 RSC.
- W. Wu, M. Aiello, T. Zhou, A. Berliner, P. Banerjee and S. Zhou, Biomaterials, 2010, 31, 3023–3031 CrossRef CAS PubMed.
- W. Wu, J. Shen, P. Banerjee and S. Zhou, Biomaterials, 2011, 32, 598–609 CrossRef CAS PubMed.
- W. Wu, J. Shen, P. Banerjee and S. Zhou, Adv. Funct. Mater., 2011, 21, 2830–2839 CrossRef CAS.
- A. H. Milani, J. M. Saunders, N. T. Nguyen, L. P. D. Ratcliffe, D. J. Adlam, A. J. Freemont, J. A. Hoyland, S. P. Armes and B. R. Saunders, Soft Matter, 2017, 13, 1554–1560 RSC.
- Y.-W. Noh, S.-H. Kong, D.-Y. Choi, H. S. Park, H.-K. Yang, H.-J. Lee, H. C. Kim, K. W. Kang, M.-H. Sung and Y. T. Lim, ACS Nano, 2012, 6, 7820–7831 CrossRef CAS PubMed.
- H. Kang, A. C. Trondoli, G. Zhu, Y. Chen, Y.-J. Chang, H. Liu, Y.-F. Huang, X. Zhang and W. Tan, ACS Nano, 2011, 5, 5094–5099 CrossRef CAS PubMed.
- T. Li, W. Zhou, Q. Song and W. Fang, J. Photochem. Photobiol., A, 2015, 302, 51–58 CrossRef CAS.
- M. Chan and A. Almutairi, Mater. Horiz., 2016, 3, 21–40 RSC.
- Z. Meng, F. Wei, R. Wang, M. Xia, Z. Chen, H. Wang and M. Zhu, Adv. Mater., 2016, 28, 245–253 CrossRef CAS PubMed.
- Y. Qu, B. Y. Chu, J. R. Peng, J. F. Liao, T. T. Qi, K. Shi, X. N. Zhang, Y. Q. Wei and Z. Y. Qian, NPG Asia Mater., 2015, 7, e207 CrossRef CAS.
- M. S. Strozyk, S. Carregal-Romero, M. Henriksen-Lacey, M. Brust and L. M. Liz-Marzán, Chem. Mater., 2017, 29, 2303–2313 CrossRef CAS.
- L. Fangyuan, L. Zeyu and L. Daishun, Curr. Med. Chem., 2017, 24, 1 Search PubMed.
- H.-Q. Wu and C.-C. Wang, Langmuir, 2016, 32, 6211–6225 CrossRef CAS PubMed.
-
W. Wu and S. Zhou, Nanomater. Drug Delivery, Imaging, Tissue Eng., John Wiley & Sons, Inc., 2013, pp. 269–319 Search PubMed.
- K. S. Soni, S. S. Desale and T. K. Bronich, J. Controlled Release, 2016, 240, 109–126 CrossRef CAS PubMed.
- X. Wang, D. Niu, Q. Wu, S. Bao, T. Su, X. Liu, S. Zhang and Q. Wang, Biomaterials, 2015, 53, 349–357 CrossRef CAS PubMed.
- M. Müllner, A. Schallon, A. Walther, R. Freitag and A. H. E. Müller, Biomacromolecules, 2010, 11, 390–396 CrossRef PubMed.
- H. Deng, Y. Zhong, M. Du, Q. Liu, Z. Fan, F. Dai and X. Zhang, Theranostics, 2014, 4, 904–918 CrossRef CAS PubMed.
- D. Chen, D. Yang, C. A. Dougherty, W. Lu, H. Wu, X. He, T. Cai, M. E. Van Dort, B. D. Ross and H. Hong, ACS Nano, 2017, 11, 4315–4327 CrossRef CAS PubMed.
- X. Wang, D. Niu, P. Li, Q. Wu, X. Bo, B. Liu, S. Bao, T. Su, H. Xu and Q. Wang, ACS Nano, 2015, 9, 5646–5656 CrossRef CAS PubMed.
- K.-T. Yong, W.-C. Law, R. Hu, L. Ye, L. Liu, M. T. Swihart and P. N. Prasad, Chem. Soc. Rev., 2013, 42, 1236–1250 RSC.
- A. M. Derfus, W. C. W. Chan and S. N. Bhatia, Nano Lett., 2004, 4, 11–18 CrossRef CAS PubMed.
- J. Mérian, J. Gravier, F. Navarro and I. Texier, Molecules, 2012, 17, 5564 CrossRef PubMed.
- S. G. Liu, T. Liu, N. Li, S. Geng, J. L. Lei, N. B. Li and H. Q. Luo, J. Phys. Chem. C, 2017, 121, 6874–6883 CAS.
- H. Wang, A. Mararenko, G. Cao, Z. Gai, K. Hong, P. Banerjee and S. Zhou, ACS Appl. Mater. Interfaces, 2014, 6, 15309–15317 CAS.
- Y. Pan, S. Neuss, A. Leifert, M. Fischler, F. Wen, U. Simon, G. Schmid, W. Brandau and W. Jahnen-Dechent, Small, 2007, 3, 1941–1949 CrossRef CAS PubMed.
- K. Kawata, M. Osawa and S. Okabe, Environ.
Sci. Technol., 2009, 43, 6046–6051 CrossRef CAS PubMed.
- S. Ravi and S. Vadukumpully, J. Environ. Chem. Eng., 2016, 4, 835–856 CrossRef CAS.
- Y. Gong, Q. Fu and C. Pan, MRS Adv., 2017, 1–7 Search PubMed.
- D. Jariwala, V. K. Sangwan, L. J. Lauhon, T. J. Marks and M. C. Hersam, Chem. Soc. Rev., 2013, 42, 2824–2860 RSC.
- X. Liu and L. Dai, Nat. Rev. Mater., 2016, 1, 16064 CrossRef CAS.
- H. Wang, J. Zhuang, D. Velado, Z. Wei, H. Matsui and S. Zhou, ACS Appl. Mater. Interfaces, 2015, 7, 27703–27712 CAS.
- H. Wang, Q. W. Chen, J. Chen, B. X. Yu and X. Y. Hu, Nanoscale, 2011, 3, 4600–4603 RSC.
- H. Wang, N. Yan, Y. Li, X. Zhou, J. Chen, B. Yu, M. Gong and Q. Chen, J. Mater. Chem., 2012, 22, 9230 RSC.
- G. Hong, S. Diao, A. L. Antaris and H. Dai, Chem. Rev., 2015, 115, 10816–10906 CrossRef CAS PubMed.
- N. Karousis, I. Suarez-Martinez, C. P. Ewels and N. Tagmatarchis, Chem. Rev., 2016, 116, 4850–4883 CrossRef CAS PubMed.
- H. Wang and S. Zhou, Biomater. Sci., 2016, 4, 1062–1073 RSC.
- S. Goodarzi, T. Da Ros, J. Conde, F. Sefat and M. Mozafari, Mater. Today, 2017, 20, 460–480 CrossRef CAS.
- A. Qureshi, W. P. Kang, J. L. Davidson and Y. Gurbuz, Diamond Relat. Mater., 2009, 18, 1401–1420 CrossRef CAS.
- E. Llobet, Sens. Actuators, B, 2013, 179, 32–45 CrossRef CAS.
- S. Kalytchuk, K. Poláková, Y. Wang, J. P. Froning, K. Cepe, A. L. Rogach and R. Zbořil, ACS Nano, 2017, 11, 1432–1442 CrossRef CAS PubMed.
- A. Salehi-Khojin, F. Khalili-Araghi, M. A. Kuroda, K. Y. Lin, J.-P. Leburton and R. I. Masel, ACS Nano, 2011, 5, 153–158 CrossRef CAS PubMed.
- Z. Zhu, L. Garcia-Gancedo, A. J. Flewitt, H. Xie, F. Moussy and W. I. Milne, Sensors, 2012, 12, 5996 CrossRef PubMed.
- N. L. Teradal and R. Jelinek, Adv. Healthcare Mater., 2017, 6, 1700574 CrossRef PubMed.
- Z. Liu, X. Sun, N. Nakayama-Ratchford and H. Dai, ACS Nano, 2007, 1, 50–56 CrossRef CAS PubMed.
- Z. Liu, S. Tabakman, K. Welsher and H. Dai, Nano Res., 2009, 2, 85–120 CrossRef CAS PubMed.
- H. Wang, Y. Sun, J. Yi, J. Fu, J. Di, A. del Carmen Alonso and S. Zhou, Biomaterials, 2015, 53, 117–126 CrossRef PubMed.
- H. Wang, K. Wang, Q. Mu, Z. R. Stephen, Y. Yu, S. Zhou and M. Zhang, Nanoscale, 2017, 9, 1434–1442 RSC.
- K. Yang, S. Zhang, G. Zhang, X. Sun, S.-T. Lee and Z. Liu, Nano Lett., 2010, 10, 3318–3323 CrossRef CAS PubMed.
- H. Wang, K. Wang, B. Tian, R. Revia, Q. Mu, M. Jeon, F.-C. Chang and M. Zhang, Small, 2016, 12, 6388–6397 CrossRef CAS PubMed.
- H. Wang, Q. Mu, R. Revia, K. Wang, X. Zhou, P. J. Pauzauskie, S. Zhou and M. Zhang, Adv. Healthcare Mater., 2017, 6, 1601080 CrossRef PubMed.
- H. Wang, J. Shen, Y. Li, Z. Wei, G. Cao, Z. Gai, K. Hong, P. Banerjee and S. Zhou, Biomater. Sci., 2014, 2, 915 RSC.
- Z. M. Markovic, B. Z. Ristic, K. M. Arsikin, D. G. Klisic, L. M. Harhaji-Trajkovic, B. M. Todorovic-Markovic, D. P. Kepic, T. K. Kravic-Stevovic, S. P. Jovanovic, M. M. Milenkovic, D. D. Milivojevic, V. Z. Bumbasirevic, M. D. Dramicanin and V. S. Trajkovic, Biomaterials, 2012, 33, 7084–7092 CrossRef CAS PubMed.
- J. Ge, M. Lan, B. Zhou, W. Liu, L. Guo, H. Wang, Q. Jia, G. Niu, X. Huang, H. Zhou, X. Meng, P. Wang, C.-S. Lee, W. Zhang and X. Han, Nat. Commun., 2014, 5, 4596 CAS.
- P. Kalluru, R. Vankayala, C.-S. Chiang and K. C. Hwang, Biomaterials, 2016, 95, 1–10 CrossRef CAS PubMed.
- D. Du, K. Wang, Y. Wen, Y. Li and Y. Y. Li, ACS Appl. Mater. Interfaces, 2016, 8, 3287–3294 CAS.
- M. Dinari, M. M. Momeni and M. Goudarzirad, Surf. Eng., 2016, 32, 535–540 CrossRef CAS.
- S. Lu, R. Cong, S. Zhu, X. Zhao, J. Liu, J. S. Tse, S. Meng and B. Yang, ACS Appl. Mater. Interfaces, 2016, 8, 4062–4068 CAS.
- D. Arthisree and G. M. Joshi, J. Mater. Sci.: Mater. Electron., 2017, 28, 10516–10524 CrossRef CAS.
- J. K. Kim, S. J. Kim, M. J. Park, S. Bae, S. P. Cho, Q. G. Du, D. H. Wang, J. H. Park and B. H. Hong, Sci. Rep., 2015, 5, 14276 CrossRef CAS PubMed.
- Y. Kim and T. S. Lee, Polym. Bull., 2016, 73, 2615–2625 CrossRef CAS.
- S. K. Bhunia, S. Nandi, R. Shikler and R. Jelinek, Nanoscale, 2016, 8, 3400–3406 RSC.
- P. Zhang, W. Li, X. Zhai, C. Liu, L. Dai and W. Liu, Chem. Commun., 2012, 48, 10431–10433 RSC.
- A. Kovalchuk, K. Huang, C. Xiang, A. A. Marti and J. M. Tour, ACS Appl. Mater. Interfaces, 2015, 7, 26063–26068 CAS.
- R. Farag and R. Mohamed, Molecules, 2013, 18, 190 CrossRef CAS PubMed.
- Y. Liu and S. Kumar, ACS Appl. Mater. Interfaces, 2014, 6, 6069–6087 CAS.
- Z. Spitalsky, D. Tasis, K. Papagelis and C. Galiotis, Prog. Polym. Sci., 2010, 35, 357–401 CrossRef CAS.
- J.-C. Huang, Adv. Polym. Technol., 2002, 21, 299–313 CrossRef CAS.
- W. Zhang, A. A. Dehghani-Sanij and R. S. Blackburn, J. Mater. Sci., 2007, 42, 3408–3418 CrossRef CAS.
- N. Jung, S. M. Kim, D. H. Kang, D. Y. Chung, Y. S. Kang, Y.-H. Chung, Y. W. Choi, C. Pang, K.-Y. Suh and Y.-E. Sung, Chem. Mater., 2013, 25, 1526–1532 CrossRef CAS.
- D. Mosconi, D. Mazzier, S. Silvestrini, A. Privitera, C. Marega, L. Franco and A. Moretto, ACS Nano, 2015, 9, 4156–4164 CrossRef CAS PubMed.
- S. Bhattacharya and S. K. Samanta, Chem. Rev., 2016, 116, 11967–12028 CrossRef CAS PubMed.
- S. Naahidi, M. Jafari, M. Logan, Y. Wang, Y. Yuan, H. Bae, B. Dixon and P. Chen, Biotechnol. Adv., 2017, 35, 530–544 CrossRef CAS PubMed.
- S. Gupta and M. K. Gupta, Nano Rev. Exp., 2017, 8, 1335567 CrossRef.
- D. Liu, F. Yang, F. Xiong and N. Gu, Theranostics, 2016, 6, 1306–1323 CrossRef CAS PubMed.
- E. L. Sievers, R. A. Larson, E. A. Stadtmauer, E. Estey, B. Löwenberg, H. Dombret, C. Karanes, M. Theobald, J. M. Bennett, M. L. Sherman, M. S. Berger, C. B. Eten, M. R. Loken, J. J. M. van Dongen, I. D. Bernstein and F. R. Appelbaum, J. Clin. Oncol., 2001, 19, 3244–3254 CrossRef CAS PubMed.
- I. F. Tannock and D. Rotin, Cancer Res., 1989, 49, 4373–4384 CAS.
- C. Song, V. Appleyard, K. Murray, T. Frank, W. Sibbett, A. Cuschieri and A. Thompson, Int. J. Cancer, 2007, 121, 1055–1058 CrossRef CAS PubMed.
- H. He, L. Sun, J. Ye, E. Liu, S. Chen, Q. Liang, M. C. Shin and V. C. Yang, J. Controlled Release, 2016, 240, 67–76 CrossRef CAS PubMed.
- J. M. Knipe, L. E. Strong and N. A. Peppas, Biomacromolecules, 2016, 17, 788–797 CrossRef CAS PubMed.
- H. R. Culver, J. R. Clegg and N. A. Peppas, Acc. Chem. Res., 2017, 50, 170–178 CrossRef CAS PubMed.
- H. Hatakeyama, Chem. Pharm. Bull., 2017, 65, 612–617 CrossRef CAS PubMed.
- Y. Zhang, J. Yu, H. N. Bomba, Y. Zhu and Z. Gu, Chem. Rev., 2016, 116, 12536–12563 CrossRef CAS PubMed.
- P. Nadrah, O. Planinšek and M. Gaberšček, J. Mater. Sci., 2014, 49, 481–495 CrossRef CAS.
-
M. Karimi, P. S. Zangabad, A. Ghasemi and M. R. Hamblin, Smart Internal Stimulus-Responsive Nanocarriers for Drug and Gene Delivery, Morgan & Claypool Publishers, 2015, pp. 2-1–2-31 Search PubMed.
- R. M. Sankar, K. M. Seeni Meera, D. Samanta, P. Jithendra, A. B. Mandal and S. N. Jaisankar, Colloids Surf., B, 2013, 112, 120–127 CrossRef CAS PubMed.
- K. Sugikawa, A. Kubo and A. Ikeda, Chem. Lett., 2016, 45, 60–62 CrossRef CAS.
- W. Li, Q. Liu, P. Zhang and L. Liu, Acta Biomater., 2016, 40, 254–262 CrossRef CAS PubMed.
- H. Wang, S. Mukherjee, J. Yi, P. Banerjee, Q. Chen and S. Zhou, ACS Appl. Mater. Interfaces, 2017, 9, 18639–18649 CAS.
- X. Jia, M. Pei, X. Zhao, K. Tian, T. Zhou and P. Liu, ACS Biomater. Sci. Eng., 2016, 2, 1641–1648 CrossRef CAS.
- S. Majumdar, G. Krishnatreya, N. Gogoi, D. Thakur and D. Chowdhury, ACS Appl. Mater. Interfaces, 2016, 8, 34179–34184 CAS.
- X. Zhang, Z. Hui, D. Wan, H. Huang, J. Huang, H. Yuan and J. Yu, Int. J. Biol. Macromol., 2010, 47, 389–395 CrossRef CAS PubMed.
- X. Xu, J. Wang, Y. Wang, L. Zhao, Y. Li and C. Liu, Nanomedicine, 2017 DOI:10.1016/j.nano.2017.05.007.
- X. Jia, Y. Han, M. Pei, X. Zhao, K. Tian, T. Zhou and P. Liu, Carbohydr. Polym., 2016, 152, 391–397 CrossRef CAS PubMed.
- A. Chandra and N. Singh, ACS Biomater. Sci. Eng., 2017, 3, 3620–3627 CrossRef CAS.
- L. Zhao, C. Xiao, L. Wang, G. Gai and J. Ding, Chem. Commun., 2016, 52, 7633–7652 RSC.
- W. Zhao, J. Hu and W. Gao, ACS Appl. Mater. Interfaces, 2017, 9, 23528–23535 CAS.
- M. Y. Kim and J. Kim, ACS Biomater. Sci. Eng., 2017, 3, 572–578 CrossRef CAS.
- Y. Zhang, Y. Guan and S. Zhou, Biomacromolecules, 2007, 8, 3842–3847 CrossRef CAS PubMed.
- J. Yu, Y. Zhang, H. Bomba and Z. Gu, Bioeng. Transl. Med., 2016, 1, 323–337 CAS.
- S. Kitano, Y. Koyama, K. Kataoka, T. Okano and Y. Sakurai, J. Controlled Release, 1992, 19, 161–170 CrossRef CAS.
- V. Lapeyre, I. Gosse, S. Chevreux and V. Ravaine, Biomacromolecules, 2006, 7, 3356–3363 CrossRef CAS PubMed.
- Y. Zhang, Y. Guan and S. Zhou, Biomacromolecules, 2006, 7, 3196–3201 CrossRef CAS PubMed.
- M. M. Zhou, J. D. Xie, S. T. Yan, X. M. Jiang, T. Ye and W. T. Wu, Macromolecules, 2014, 47, 6055–6066 CrossRef CAS.
- H. Wang, J. Yi, D. Velado, Y. Yu and S. Zhou, ACS Appl. Mater. Interfaces, 2015, 7, 15735–15745 CAS.
- J. Lee, S. Ko, C. H. Kwon, M. D. Lima, R. H. Baughman and S. J. Kim, Small, 2016, 12, 2085–2091 CrossRef CAS PubMed.
- Y. Wang, H. Xu and L. Ma, Ther. Delivery, 2015, 6, 1157–1169 CrossRef CAS PubMed.
- R. Laga, O. Janoušková, K. Ulbrich, R. Pola, J. Blažková, S. K. Filippov, T. Etrych and M. Pechar, Biomacromolecules, 2015, 16, 2493–2505 CrossRef CAS PubMed.
- H. Vihola, A. Laukkanen, L. Valtola, H. Tenhu and J. Hirvonen, Biomaterials, 2005, 26, 3055–3064 CrossRef CAS PubMed.
- R. H. Pelton and P. Chibante, Colloids Surf., 1986, 20, 247–256 CrossRef CAS.
- C. Wu and S. Zhou, Macromolecules, 1995, 28, 8381–8387 CrossRef CAS.
- M. Karbarz, M. Mackiewicz, K. Kaniewska, K. Marcisz and Z. Stojek, Appl. Mater. Today, 2017, 9, 516–532 CrossRef.
- H. G. Schild, Prog. Polym. Sci., 1992, 17, 163–249 CrossRef CAS.
- M. Patenaude and T. Hoare, ACS Macro Lett., 2012, 1, 409–413 CrossRef CAS.
- N. Lu, J. Liu, J. Li, Z. Zhang, Y. Weng, B. Yuan, K. Yang and Y. Ma, J. Mater. Chem. B, 2014, 2, 3791–3798 RSC.
- A. N. Lukyanov, Z. Gao, L. Mazzola and V. P. Torchilin, Pharm. Res., 2002, 19, 1424–1429 CrossRef CAS.
- K. Knop, R. Hoogenboom, D. Fischer and U. S. Schubert, Angew. Chem., Int. Ed., 2010, 49, 6288–6308 CrossRef CAS PubMed.
- J.-F. Lutz, Ö. Akdemir and A. Hoth, J. Am. Chem. Soc., 2006, 128, 13046–13047 CrossRef CAS PubMed.
- J.-F. Lutz and A. Hoth, Macromolecules, 2006, 39, 893–896 CrossRef CAS.
- J.-F. Lutz, K. Weichenhan, Ö. Akdemir and A. Hoth, Macromolecules, 2007, 40, 2503–2508 CrossRef CAS.
- J.-F. Lutz, J. Polym. Sci., Part A: Polym. Chem., 2008, 46, 3459–3470 CrossRef CAS.
- C. Chi, T. Cai and Z. Hu, Langmuir, 2009, 25, 3814–3819 CrossRef CAS PubMed.
- T. Ishizone, A. Seki, M. Hagiwara, S. Han, H. Yokoyama, A. Oyane, A. Deffieux and S. Carlotti, Macromolecules, 2008, 41, 2963–2967 CrossRef CAS.
- T. Cai, M. Marquez and Z. Hu, Langmuir, 2007, 23, 8663–8666 CrossRef CAS PubMed.
- T. Cai, G. Wang, S. Thompson, M. Marquez and Z. Hu, Macromolecules, 2008, 41, 9508–9512 CrossRef CAS.
- Z. Hu, T. Cai and C. Chi, Soft Matter, 2010, 6, 2115–2123 RSC.
- J.-F. Lutz, Adv. Mater., 2011, 23, 2237–2243 CrossRef CAS.
- H. Wang, J. Di, Y. Sun, J. Fu, Z. Wei, H. Matsui, A. del, C. Alonso and S. Zhou, Adv. Funct. Mater., 2015, 25, 5537–5547 CrossRef CAS.
- X. Ai, J. Mu and B. Xing, Theranostics, 2016, 6, 2439–2457 CrossRef CAS PubMed.
- F. F. Jo Bsis-Vandervliet, J. Biomed. Opt., 1999, 4, 392–396 CrossRef PubMed.
- M. Ferrari and V. Quaresima, NeuroImage, 2012, 63, 921–935 CrossRef PubMed.
- H. Wang, F. Ke, A. Mararenko, Z. Wei, P. Banerjee and S. Zhou, Nanoscale, 2014, 6, 7443–7452 RSC.
- Z. Khatun, M. Nurunnabi, M. Nafiujjaman, G. R. Reeck, H. A. Khan, K. J. Cho and Y.-k. Lee, Nanoscale, 2015, 7, 10680–10689 RSC.
- C. Fiorica, N. Mauro, G. Pitarresi, C. Scialabba, F. S. Palumbo and G. Giammona, Biomacromolecules, 2017, 18, 1010–1018 CrossRef CAS PubMed.
- U. G. Spizzirri, S. Hampel, G. Cirillo, F. P. Nicoletta, A. Hassan, O. Vittorio, N. Picci and F. Iemma, Int. J. Pharm., 2013, 448, 115–122 CrossRef CAS PubMed.
- S. Sun and P. Wu, J. Mater. Chem., 2011, 21, 4095–4097 RSC.
- G. R. Bardajee, Z. Hooshyar, M. Farsi, A. Mobini and G. Sang, Mater. Sci. Eng., C, 2017, 72, 558–565 CrossRef CAS PubMed.
- C. Hou, L. Ji, Q. Zhang, Y. Li and H. Wang, J. Appl. Polym. Sci., 2013, 130, 2422–2428 CrossRef.
- J. V. Alemán, A. V. Chadwick, J. He, M. Hess, K. Horie, R. G. Jones, P. Kratochvíl, I. Meisel, I. Mita, G. Moad, S. Penczek and R. F. T. Stepto, Pure Appl. Chem., 2007, 79, 1801–1829 CrossRef.
- Z. Xing, C. Wang, J. Yan, L. Zhang, L. Li and L. Zha, Soft Matter, 2011, 7, 7992–7997 RSC.
-
M. Karimi, P. S. Zangabad, A. Ghasemi and M. R. Hamblin, Smart Internal Stimulus-Responsive Nanocarriers for Drug and Gene Delivery, Morgan & Claypool Publishers, 2015, pp. 6-1–6-23 Search PubMed.
- X. Liu, H. Guo and L. Zha, Polym. Int., 2012, 61, 1144–1150 CrossRef CAS.
- N. do Nascimento Marques, P. S. Curti, A. M. da Silva Maia and R. D. C. Balaban, J. Appl. Polym. Sci., 2013, 129, 334–345 CrossRef CAS.
- H. Wang, T. Dai, S. Zhou, X. Huang, S. Li, K. Sun, G. Zhou and H. Dou, Sci. Rep., 2017, 7, 40011 CrossRef CAS PubMed.
- Y. Qin, J. Chen, Y. Bi, X. Xu, H. Zhou, J. Gao, Y. Hu, Y. Zhao and Z. Chai, Acta Biomater., 2015, 17, 201–209 CrossRef CAS PubMed.
- T. Zhou, C. Xiao, J. Fan, S. Chen, J. Shen, W. Wu and S. Zhou, Acta Biomater., 2013, 9, 4546–4557 CrossRef CAS PubMed.
- N. A. Jalili, M. Muscarello and A. K. Gaharwar, Bioeng. Transl. Med., 2016, 1, 297–305 CAS.
- A. J. Giustini, A. A. Petryk, S. M. Cassim, J. A. Tate, I. Baker and P. J. Hoopes, Nano LIFE, 2010, 01, 17–32 CrossRef CAS PubMed.
- M. Bañobre-López, A. Teijeiro and J. Rivas, Rep. Pract. Oncol. Radiother., 2013, 18, 397–400 CrossRef PubMed.
- H. Wang, J. Yi, S. Mukherjee, P. Banerjee and S. Zhou, Nanoscale, 2014, 6, 13001–13011 RSC.
- S. Yue, X. Sun, N. Wang, Y. Wang, Y. Wang, Z. Xu, M. Chen and J. Wang, ACS Appl. Mater. Interfaces, 2017, 9, 39699–39707 CAS.
- X. Li, M. Rui, J. Song, Z. Shen and H. Zeng, Adv. Funct. Mater., 2015, 25, 4929–4947 CrossRef CAS.
- Y.-P. Sun, B. Zhou, Y. Lin, W. Wang, K. A. S. Fernando, P. Pathak, M. J. Meziani, B. A. Harruff, X. Wang, H. Wang, P. G. Luo, H. Yang, M. E. Kose, B. Chen, L. M. Veca and S.-Y. Xie, J. Am. Chem. Soc., 2006, 128, 7756–7757 CrossRef CAS PubMed.
- S.-T. Yang, X. Wang, H. Wang, F. Lu, P. G. Luo, L. Cao, M. J. Meziani, J.-H. Liu, Y. Liu, M. Chen, Y. Huang and Y.-P. Sun, J. Phys. Chem. C, 2009, 113, 18110–18114 CAS.
- S. Zhu, Q. Meng, L. Wang, J. Zhang, Y. Song, H. Jin, K. Zhang, H. Sun, H. Wang and B. Yang, Angew. Chem., Int. Ed., 2013, 52, 3953–3957 CrossRef CAS PubMed.
- K. Hola, Y. Zhang, Y. Wang, E. P. Giannelis, R. Zboril and A. L. Rogach, Nano Today, 2014, 9, 590–603 CrossRef CAS.
- J. Wang and J. Qiu, J. Mater. Sci., 2016, 51, 4728–4738 CrossRef CAS.
- P. Zuo, X. Lu, Z. Sun, Y. Guo and H. He, Microchim. Acta, 2016, 183, 519–542 CrossRef CAS.
- V. Sharma, P. Tiwari and S. M. Mobin, J. Mater. Chem. B, 2017, 5, 8904–8924 RSC.
- X. Sun and Y. Lei, TrAC, Trends Anal. Chem., 2017, 89, 163–180 CrossRef CAS.
- H. Sun, L. Wu, W. Wei and X. Qu, Mater. Today, 2013, 16, 433–442 CrossRef CAS.
- S. Wang, I. S. Cole and Q. Li, RSC Adv., 2016, 6, 89867–89878 RSC.
- H. Wang, R. Revia, K. Wang, R. J. Kant, Q. Mu, Z. Gai, K. Hong and M. Zhang, Adv. Mater., 2017, 29, 1605416 CrossRef PubMed.
- M. Bacon, S. J. Bradley and T. Nann, Part. Part. Syst. Charact., 2014, 31, 415–428 CrossRef CAS.
- J.-M. Liu, F.-Z. Cao, G.-Z. Fang and S. Wang, Polymers, 2017, 9, 299 CrossRef.
- C. Wang, Y. Li and Y. Wei, Sens. Actuators, B, 2017, 247, 595–601 CrossRef CAS.
- X. T. Zheng, A. Ananthanarayanan, K. Q. Luo and P. Chen, Small, 2015, 11, 1620–1636 CrossRef CAS PubMed.
- J. Ge, Q. Jia, W. Liu, L. Guo, Q. Liu, M. Lan, H. Zhang, X. Meng and P. Wang, Adv. Mater., 2015, 27, 4169–4177 CrossRef CAS PubMed.
- S. Y. Lim, W. Shen and Z. Gao, Chem. Soc. Rev., 2015, 44, 362–381 RSC.
- B. B. Campos, D. Mutavdzic, M. Stankovic, K. Radotic, J. M. Lazaro-Martinez, J. C. G. Esteves da Silva, R. Contreras-Caceres, M. S. Pino-Gonzalez, E. Rodriguez-Castellon and M. Algarra, New J. Chem., 2017, 41, 4835–4842 RSC.
- D. Y. Li, X. M. Zhang, Y. J. Yan, X. W. He, W. Y. Li and Y. K. Zhang, Biosens. Bioelectron., 2016, 79, 187–192 CrossRef CAS PubMed.
- Y. Liu, Y. Tian, Y. Tian, Y. Wang and W. Yang, Adv. Mater., 2015, 27, 7156–7160 CrossRef CAS PubMed.
- C. Wang, J. Mallela, U. S. Garapati, S. Ravi, V. Chinnasamy, Y. Girard, M. Howell and S. Mohapatra, Nanomedicine, 2013, 9, 903–911 CrossRef CAS PubMed.
- M. Zhou, J. Xie, S. Yan, X. Jiang, T. Ye and W. Wu, Macromolecules, 2014, 47, 6055–6066 CrossRef CAS.
- D. S. Kwag, K. Park, K. T. Oh and E. S. Lee, Chem. Commun., 2013, 49, 282–284 RSC.
- S. Kim, D. J. Lee, D. S. Kwag, U. Y. Lee, Y. S. Youn and E. S. Lee, Carbohydr. Polym., 2014, 101, 692–698 CrossRef CAS PubMed.
- H. A. Girard, P. Benayoun, C. Blin, A. Trouvé, C. Gesset, J.-C. Arnault and P. Bergonzo, Diamond Relat. Mater., 2013, 33, 32–37 CrossRef CAS.
- H.-J. Kim, K. Zhang, L. Moore and D. Ho, ACS Nano, 2014, 8, 2998–3005 CrossRef CAS PubMed.
- T. A. Debele, S. L. Mekuria and H.-C. Tsai, Mater. Sci. Eng., C, 2016, 68, 964–981 CrossRef CAS PubMed.
- X. K. Kong, C. L. Chen and Q. W. Chen, Chem. Soc. Rev., 2014, 43, 2841–2857 RSC.
- G. Reina, J. M. Gonzalez-Dominguez, A. Criado, E. Vazquez, A. Bianco and M. Prato, Chem. Soc. Rev., 2017, 46, 4400–4416 RSC.
- X. Huang, Z. Y. Yin, S. X. Wu, X. Y. Qi, Q. Y. He, Q. C. Zhang, Q. Y. Yan, F. Boey and H. Zhang, Small, 2011, 7, 1876–1902 CrossRef CAS PubMed.
- H. Shen, L. M. Zhang, M. Liu and Z. J. Zhang, Theranostics, 2012, 2, 283–294 CrossRef CAS PubMed.
- H. Zhao, R. Ding, X. Zhao, Y. Li, L. Qu, H. Pei, L. Yildirimer, Z. Wu and W. Zhang, Drug Discovery Today, 2017, 22, 1302–1317 CrossRef CAS PubMed.
- K. Yang, S. A. Zhang, G. X. Zhang, X. M. Sun, S. T. Lee and Z. A. Liu, Nano Lett., 2010, 10, 3318–3323 CrossRef CAS PubMed.
- J. T. Robinson, S. M. Tabakman, Y. Y. Liang, H. L. Wang, H. S. Casalongue, D. Vinh and H. J. Dai, J. Am. Chem. Soc., 2011, 133, 6825–6831 CrossRef CAS PubMed.
- L. M. Zhang, J. G. Xia, Q. H. Zhao, L. W. Liu and Z. J. Zhang, Small, 2010, 6, 537–544 CrossRef CAS PubMed.
- X. X. Ma, H. Q. Tao, K. Yang, L. Z. Feng, L. Cheng, X. Z. Shi, Y. G. Li, L. Guo and Z. Liu, Nano Res., 2012, 5, 199–212 CrossRef CAS.
- B. A. Chen, M. Liu, L. M. Zhang, J. Huang, J. L. Yao and Z. J. Zhang, J. Mater. Chem., 2011, 21, 7736–7741 RSC.
- L. M. Zhang, Z. X. Lu, Q. H. Zhao, J. Huang, H. Shen and Z. J. Zhang, Small, 2011, 7, 460–464 CrossRef CAS PubMed.
- H. Kim, J. Kim, M. Lee, H. C. Choi and W. J. Kim, Adv. Healthcare Mater., 2016, 5, 1918–1930 CrossRef CAS PubMed.
- S. Viswanathan, T. N. Narayanan, K. Aran, K. D. Fink, J. Paredes, P. M. Ajayan, S. Filipek, P. Miszta, H. C. Tekin, F. Inci, U. Demirci, P. Li, K. I. Bolotin, D. Liepmann and V. Renugopalakrishanan, Mater. Today, 2015, 18, 513–522 CrossRef CAS.
- X. M. Sun, Z. Liu, K. Welsher, J. T. Robinson, A. Goodwin, S. Zaric and H. J. Dai, Nano Res., 2008, 1, 203–212 CrossRef CAS PubMed.
- E. Q. Song, W. Y. Han, C. Li, D. Cheng, L. R. Li, L. C. Liu, G. Z. Zhu, Y. Song and W. H. Tan, ACS Appl. Mater. Interfaces, 2014, 6, 11882–11890 CAS.
- Z. Khatun, M. Nurunnabi, M. Nafiujjaman, G. R. Reeck, H. A. Khan, K. J. Cho and Y. K. Lee, Nanoscale, 2015, 7, 10680–10689 RSC.
- A. A. Popov, S. Yang and L. Dunsch, Chem. Rev., 2013, 113, 5989–6113 CrossRef CAS PubMed.
- H. W. Kroto, J. R. Heath, S. C. O'Brien, R. F. Curl and R. E. Smalley, Nature, 1985, 318, 162–163 CrossRef CAS.
- H. Murayama, S. Tomonoh, J. M. Alford and M. E. Karpuk, Fullerenes, Nanotubes, Carbon Nanostruct., 2004, 12, 1–9 CAS.
- J. Coro, M. Suárez, L. S. R. Silva, K. I. B. Eguiluz and G. R. Salazar-Banda, Int. J. Hydrogen Energy, 2016, 41, 17944–17959 CrossRef CAS.
- I. Rašović, Mater. Sci. Technol., 2017, 33, 777–794 CrossRef.
- S. Marchesan, T. Da Ros, G. Spalluto, J. Balzarini and M. Prato, Bioorg. Med. Chem. Lett., 2005, 15, 3615–3618 CrossRef CAS PubMed.
- Z. S. Martinez, E. Castro, C. S. Seong, M. R. Ceron, L. Echegoyen and M. Llano, Antimicrob. Agents Chemother., 2016, 60, 5731–5741 CrossRef CAS PubMed.
- E. Castro, A. H. Garcia, G. Zavala and L. Echegoyen, J. Mater. Chem. B, 2017, 5, 6523–6535 RSC.
- D. Y. Lyon, L. Brunet, G. W. Hinkal, M. R. Wiesner and P. J. J. Alvarez, Nano Lett., 2008, 8, 1539–1543 CrossRef CAS PubMed.
- A. B. Kornev, A. S. Peregudov, V. M. Martynenko, J. Balzarini, B. Hoorelbeke and P. A. Troshin, Chem. Commun., 2011, 47, 8298–8300 RSC.
- N. Gharbi, M. Pressac, M. Hadchouel, H. Szwarc, S. R. Wilson and F. Moussa, Nano Lett., 2005, 5, 2578–2585 CrossRef CAS PubMed.
- Z. Y. Chen, L. J. Ma, Y. Liu and C. Y. Chen, Theranostics, 2012, 2, 238–250 CrossRef CAS PubMed.
- R. Partha and J. L. Conyers, Int. J. Nanomed., 2009, 4, 261–275 CrossRef CAS.
- V. Krishna, A. Singh, P. Sharma, N. Iwakuma, Q. A. Wang, Q. Z. Zhang, J. Knapik, H. B. Jiang, S. R. Grobmyer, B. Koopman and B. Moudgil, Small, 2010, 6, 2236–2241 CrossRef CAS PubMed.
- J. J. Shi, X. Y. Yu, L. Wang, Y. Liu, J. Gao, J. Zhang, R. Ma, R. Y. Liu and Z. Z. Zhang, Biomaterials, 2013, 34, 9666–9677 CrossRef CAS PubMed.
- C. Chen, J. Geng, F. Pu, X. Yang, J. Ren and X. Qu, Angew. Chem., Int. Ed., 2011, 50, 882–886 CrossRef CAS PubMed.
- Y. S. Youn, D. S. Kwag and E. S. Lee, J. Pharm. Invest., 2016, 47, 1–10 Search PubMed.
- S. Kim, J. Park, Y. S. Youn, K. T. Oh, J. H. Bae and E. S. Lee, J. Bioact. Compat. Polym., 2015, 30, 275–288 CrossRef CAS.
- Z. Chen, L. Ma, Y. Liu and C. Chen, Theranostics, 2012, 2, 238–250 CrossRef CAS PubMed.
- M. Prato, K. Kostarelos and A. Bianco, Acc. Chem. Res., 2008, 41, 60–68 CrossRef CAS PubMed.
- S. Iijima, Nature, 1991, 354, 56–58 CrossRef CAS.
- S. Iijima and T. Ichihashi, Nature, 1993, 363, 603–605 CrossRef CAS.
- M. Monthioux and V. L. Kuznetsov, Carbon, 2006, 44, 1621–1623 CrossRef CAS.
- J. Bernholc, D. Brenner, M. Buongiorno Nardelli, V. Meunier and C. Roland, Annu. Rev. Mater. Res., 2002, 32, 347–375 CrossRef CAS.
- M. Moniruzzaman, A. Sahin and K. I. Winey, Carbon, 2009, 47, 645–650 CrossRef CAS.
- S. Roy and A. Banerjee, RSC Adv., 2012, 2, 2105–2111 RSC.
- N. Martín, T. Da Ros and J.-F. Nierengarten, J. Mater. Chem. B, 2017, 5, 6425–6427 RSC.
- S. Jin, P. Wijesekara, P. D. Boyer, K. N. Dahl and M. F. Islam, J. Mater. Chem. B, 2017, 5, 6657–6665 RSC.
- J. Bartelmess, S. J. Quinn and S. Giordani, Chem. Soc. Rev., 2015, 44, 4672–4698 RSC.
- C. Guo, W. T. Al-Jamal, F. M. Toma, A. Bianco, M. Prato, K. T. Al-Jamal and K. Kostarelos, Bioconjugate Chem., 2015, 26, 1370–1379 CrossRef CAS PubMed.
- N. W. Kam, M. O'Connell, J. A. Wisdom and H. Dai, Proc. Natl. Acad. Sci. U. S. A., 2005, 102, 11600–11605 CrossRef CAS PubMed.
- J. T. Robinson, K. Welsher, S. M. Tabakman, S. P. Sherlock, H. Wang, R. Luong and H. Dai, Nano Res., 2010, 3, 779–793 CrossRef CAS PubMed.
- T. Murakami, H. Nakatsuji, M. Inada, Y. Matoba, T. Umeyama, M. Tsujimoto, S. Isoda, M. Hashida and H. Imahori, J. Am. Chem. Soc., 2012, 134, 17862–17865 CrossRef CAS PubMed.
- S. Diao, J. L. Blackburn, G. Hong, A. L. Antaris, J. Chang, J. Z. Wu, B. Zhang, K. Cheng, C. J. Kuo and H. Dai, Angew. Chem., Int. Ed., 2015, 54, 14758–14762 CrossRef CAS PubMed.
- G. Hong and H. Dai, Methods Mol. Biol., 2016, 1444, 167–181 Search PubMed.
- K. Welsher, Z. Liu, S. P. Sherlock, J. T. Robinson, Z. Chen, D. Daranciang and H. Dai, Nat. Nanotechnol., 2009, 4, 773–780 CrossRef CAS PubMed.
- B. K. Gorityala, J. Ma, X. Wang, P. Chen and X.-W. Liu, Chem. Soc. Rev., 2010, 39, 2925–2934 RSC.
- S. Prakash, M. Malhotra, W. Shao, C. Tomaro-Duchesneau and S. Abbasi, Adv. Drug Delivery Rev., 2011, 63, 1340–1351 CrossRef CAS PubMed.
- S. R. Shin, H. Bae, J. M. Cha, J. Y. Mun, Y.-C. Chen, H. Tekin, H. Shin, S. Farshchi, M. R. Dokmeci, S. Tang and A. Khademhosseini, ACS Nano, 2012, 6, 362–372 CrossRef CAS PubMed.
- G. Cirillo, S. Hampel, U. G. Spizzirri, O. I. Parisi, N. Picci and F. Iemma, BioMed Res. Int., 2014, 2014, 17 Search PubMed.
- X. Shi, Y. Zheng, C. Wang, L. Yue, K. Qiao, G. Wang, L. Wang and H. Quan, RSC Adv., 2015, 5, 41820–41829 RSC.
- A. Servant, L. Methven, R. P. Williams and K. Kostarelos, Adv. Healthcare Mater., 2013, 2, 806–811 CrossRef CAS PubMed.
- X. Dong, C. Wei, J. Liang, T. Liu, D. Kong and F. Lv, Colloids Surf., B, 2017, 154, 253–262 CrossRef CAS PubMed.
- O. A. Shenderova and G. E. McGuire, Biointerphases, 2015, 10, 030802 CrossRef PubMed.
- V. N. Mochalin, O. Shenderova, D. Ho and Y. Gogotsi, Nat. Nanotechnol., 2012, 7, 11–23 CrossRef CAS PubMed.
- A. M. Schrand, S. A. C. Hens and O. A. Shenderova, Crit. Rev. Solid State Mater. Sci., 2009, 34, 18–74 CrossRef CAS.
- L. Moore, J. Yang, T. T. Lan, E. Osawa, D. K. Lee, W. D. Johnson, J. Xi, E. K. Chow and D. Ho, ACS Nano, 2016, 10, 7385–7400 CrossRef CAS PubMed.
- X. Chen and W. Zhang, Chem. Soc. Rev., 2017, 46, 734–760 RSC.
- E. K. Chow, X.-Q. Zhang, M. Chen, R. Lam, E. Robinson, H. Huang, D. Schaffer, E. Osawa, A. Goga and D. Ho, Sci. Transl. Med., 2011, 3, 73ra21 Search PubMed.
- L. Moore, E. K.-H. Chow, E. Osawa, J. M. Bishop and D. Ho, Adv. Mater., 2013, 25, 3532–3541 CrossRef CAS PubMed.
- W. W.-W. Hsiao, Y. Y. Hui, P.-C. Tsai and H.-C. Chang, Acc. Chem. Res., 2016, 49, 400–407 CrossRef CAS PubMed.
- R. Kaur and I. Badea, Int. J. Nanomed., 2013, 8, 203–220 CrossRef PubMed.
- T.-J. Wu, Y.-K. Tzeng, W.-W. Chang, C.-A. Cheng, Y. Kuo, C.-H. Chien, H.-C. Chang and J. Yu, Nat. Nanotechnol., 2013, 8, 682–689 CrossRef CAS PubMed.
- D.
E. J. Waddington, M. Sarracanie, H. Zhang, N. Salameh, D. R. Glenn, E. Rej, T. Gaebel, T. Boele, R. L. Walsworth, D. J. Reilly and M. S. Rosen, Nat. Commun., 2017, 8, 15118 CrossRef PubMed.
- M. Hartmann, P. Betz, Y. Sun, S. N. Gorb, T. K. Lindhorst and A. Krueger, Chem. – Eur. J., 2012, 18, 6485–6492 CrossRef CAS PubMed.
- X. Wang, X. C. Low, W. Hou, L. N. Abdullah, T. B. Toh, M. Mohd Abdul Rashid, D. Ho and E. K.-H. Chow, ACS Nano, 2014, 8, 12151–12166 CrossRef CAS PubMed.
- S. Jabeen, A. Kausar, B. Muhammad, S. Gul and M. Farooq, Polym.-Plast. Technol. Eng., 2015, 54, 1379–1409 CrossRef CAS.
- S. Pacelli, R. Maloney, A. R. Chakravarti, J. Whitlow, S. Basu, S. Modaresi, S. Gehrke and A. Paul, Sci. Rep., 2017, 7, 6577 CrossRef PubMed.
- S. Pacelli, F. Acosta, A. R. Chakravarti, S. G. Samanta, J. Whitlow, S. Modaresi, R. P. H. Ahmed, J. Rajasingh and A. Paul, Acta Biomater., 2017, 58, 479–491 CrossRef CAS PubMed.
- D.-K. Lee, S. V. Kim, A. N. Limansubroto, A. Yen, A. Soundia, C.-Y. Wang, W. Shi, C. Hong, S. Tetradis, Y. Kim, N.-H. Park, M. K. Kang and D. Ho, ACS Nano, 2015, 9, 11490–11501 CrossRef CAS PubMed.
- C. Hong, D. Song, D.-K. Lee, L. Lin, H. C. Pan, D. Lee, P. Deng, Z. Liu, D. Hadaya, H.-L. Lee, A. Mohammad, X. Zhang, M. Lee, C.-Y. Wang and D. Ho, Proc. Natl. Acad. Sci. U. S. A., 2017, 114, 7218–7225 CrossRef PubMed.
- P. Rouhani, N. Govindaraju, J. K. Iyer, R. Kaul, A. Kaul and R. N. Singh, Mater. Sci. Eng., C, 2016, 63, 323–332 CrossRef CAS PubMed.
- L. Zhao, Y.-H. Xu, T. Akasaka, S. Abe, N. Komatsu, F. Watari and X. Chen, Biomaterials, 2014, 35, 5393–5406 CrossRef CAS PubMed.
- J.-P. Boudou, M.-O. David, V. Joshi, H. Eidi and P. A. Curmi, Diamond Relat. Mater., 2013, 38, 131–138 CrossRef CAS.
- M. C. Lukowiak, B. Ziem, K. Achazi, G. Gunkel-Grabole, C. S. Popeney, B. N. S. Thota, C. Bottcher, A. Krueger, Z. Guan and R. Haag, J. Mater. Chem. B, 2015, 3, 719–722 RSC.
- D. G. Lim, N. Rajasekaran, D. Lee, N. A. Kim, H. S. Jung, S. Hong, Y. K. Shin, E. Kang and S. H. Jeong, ACS Appl. Mater. Interfaces, 2017, 9, 31543–31556 CAS.
- P. M. Peiris, L. Bauer, R. Toy, E. Tran, J. Pansky, E. Doolittle, E. Schmidt, E. Hayden, A. Mayer, R. A. Keri, M. A. Griswold and E. Karathanasis, ACS Nano, 2012, 6, 4157–4168 CrossRef CAS PubMed.
- R. Toy, P. M. Peiris, K. B. Ghaghada and E. Karathanasis, Nanomedicine, 2014, 9, 121–134 CrossRef CAS PubMed.
- A. Gabizon and D. Papahadjopoulos, Proc. Natl. Acad. Sci. U. S. A., 1988, 85, 6949–6953 CrossRef CAS.
- W. Richtering and A. Pich, Soft Matter, 2012, 8, 11423 RSC.
- M. Karg, Colloid Polym. Sci., 2012, 290, 673–688 CAS.
- G. R. Hendrickson, M. H. Smith, A. B. South and L. A. Lyon, Adv. Funct. Mater., 2010, 20, 1697–1712 CrossRef CAS.
- M. H. Smith and L. A. Lyon, Acc. Chem. Res., 2012, 45, 985–993 CrossRef CAS PubMed.
- J. Dubbert, T. Honold, J. S. Pedersen, A. Radulescu, M. Drechsler, M. Karg and W. Richtering, Macromolecules, 2014, 47, 8700–8708 CrossRef CAS.
- V. Lapeyre, N. Renaudie, J.-F. Dechezelles, H. Saadaoui, S. Ravaine and V. Ravaine, Langmuir, 2009, 25, 4659–4667 CrossRef CAS PubMed.
- R. Contreras-Cáceres, L. Schellkopf, C. Fernández-López, I. Pastoriza-Santos, J. Pérez-Juste and M. Stamm, Langmuir, 2015, 31, 1142–1149 CrossRef PubMed.
- Y. Chen, X. Zheng, H. Qian, Z. Mao, D. Ding and X. Jiang, ACS Appl. Mater. Interfaces, 2010, 2, 3532–3538 CAS.
- H. Wang, J. Yi, Y. Yu and S. Zhou, Nanoscale, 2017, 9, 509–516 RSC.
- F. Q. Schafer and G. R. Buettner, Free Radical Biol. Med., 2001, 30, 1191–1212 CrossRef CAS PubMed.
- D. E. Hutter, B. G. Till and J. J. Greene, Exp. Cell Res., 1997, 232, 435–438 CrossRef CAS PubMed.
- Y.-E. K. Lee and R. Kopelman, Wiley Interdiscip. Rev.: Nanomed. Nanobiotechnol., 2009, 1, 98–110 CrossRef CAS PubMed.
- Y.-E. K. Lee, R. Kopelman and R. Smith, Annu. Rev. Anal. Chem., 2009, 2, 57–76 CrossRef PubMed.
- M. Stubbs, P. M. J. McSheehy, J. R. Griffiths and C. L. Bashford, Mol. Med. Today, 2000, 6, 15–19 CrossRef CAS PubMed.
- R. D. Coakley, B. R. Grubb, A. M. Paradiso, J. T. Gatzy, L. G. Johnson, S. M. Kreda, W. K. O'Neal and R. C. Boucher, Proc. Natl. Acad. Sci. U. S. A., 2003, 100, 16083–16088 CrossRef CAS PubMed.
- P. Çakir, A. Cutivet, M. Resmini, B. T. S. Bui and K. Haupt, Adv. Mater., 2013, 25, 1048–1051 CrossRef PubMed.
- M. Bertolla, L. Cenci, A. Anesi, E. Ambrosi, F. Tagliaro, L. Vanzetti, G. Guella and A. M. Bossi, ACS Appl. Mater. Interfaces, 2017, 9, 6908–6915 CAS.
- G. Wulff and A. Sarhan, Angew. Chem., Int. Ed., 1972, 84, 364 Search PubMed.
- R. Arshady and K. Mosbach, Macromol. Chem. Phys., 1981, 182, 687–692 CrossRef CAS.
- S. C. Lu, Mol. Aspects Med., 2009, 30, 42–59 CrossRef CAS PubMed.
- A. Meister and M. E. Anderson, Annu. Rev. Biochem., 1983, 52, 711–760 CrossRef CAS PubMed.
- C. Hwang, A. J. Sinskey and H. F. Lodish, Science, 1992, 257, 1496–1502 CAS.
- G. K. Such, Y. Yan, A. P. R. Johnston, S. T. Gunawan and F. Caruso, Adv. Mater., 2015, 27, 2278–2297 CrossRef CAS PubMed.
- R. L. Joana, S. Gory, B. Pedro, O. Rita and M. L. Carla, Curr. Pharm. Des., 2013, 19, 7169–7184 CrossRef.
- H. Chen, D. Liu and Z. Guo, Chem. Lett., 2016, 45, 242–249 CrossRef CAS.
- J. Yao, J. Feng and J. Chen, Asian J. Pharm. Sci., 2016, 11, 585–595 CrossRef.
- M. Huo, Y. Chen and J. Shi, Expert Opin. Drug Delivery, 2016, 13, 1195–1198 CrossRef PubMed.
- R. Contreras-Cáceres, A. Sánchez-Iglesias, M. Karg, I. Pastoriza-Santos, J. Pérez-Juste, J. Pacifico, T. Hellweg, A. Fernández-Barbero and L. M. Liz-Marzán, Adv. Mater., 2008, 20, 1666–1670 CrossRef.
- R. A. Álvarez-Puebla, R. Contreras-Cáceres, I. Pastoriza-Santos, J. Pérez-Juste and L. M. Liz-Marzán, Angew. Chem., Int. Ed., 2009, 48, 138–143 CrossRef PubMed.
- G. Hong, S. Diao, A. L. Antaris and H. Dai, Chem. Rev., 2015, 115, 10816–10906 CrossRef CAS PubMed.
- B. Zhu and E. M. Sevick-Muraca, Br. J. Radiol., 2014, 88, 20140547 CrossRef PubMed.
- E. İ. Altınoğlu and J. H. Adair, Wiley Interdiscip. Rev.: Nanomed. Nanobiotechnol., 2010, 2, 461–477 CrossRef PubMed.
- G. Prencipe, S. M. Tabakman, K. Welsher, Z. Liu, A. P. Goodwin, L. Zhang, J. Henry and H. Dai, J. Am. Chem. Soc., 2009, 131, 4783–4787 CrossRef CAS PubMed.
- D. E. Dolmans, D. Fukumura and R. K. Jain, Nat. Rev. Cancer, 2003, 3, 380 CrossRef CAS PubMed.
- S. S. Lucky, K. C. Soo and Y. Zhang, Chem. Rev., 2015, 115, 1990–2042 CrossRef CAS PubMed.
- D. Jaque, L. Martinez Maestro, B. del Rosal, P. Haro-Gonzalez, A. Benayas, J. L. Plaza, E. Martin Rodriguez and J. Garcia Sole, Nanoscale, 2014, 6, 9494–9530 RSC.
- J. V. Frangioni, Curr. Opin. Chem. Biol., 2003, 7, 626–634 CrossRef CAS PubMed.
- B. Zhang, R. B. Kumar, H. Dai and B. J. Feldman, Nat. Med., 2014, 20, 948–953 CrossRef CAS PubMed.
- A. N. Bashkatov, E. A. Genina, V. I. Kochubey and V. V. Tuchin, J. Phys. D: Appl. Phys., 2005, 38, 2543 CrossRef CAS.
- N. G. Horton, K. Wang, D. Kobat, C. G. Clark, F. W. Wise, C. B. Schaffer and C. Xu, Nat. Photonics, 2013, 7, 205–209 CrossRef CAS PubMed.
- A. N. Bashkatov, E. A. Genina and V. V. Tuchin, J. Innovative Opt. Health Sci., 2011, 04, 9–38 CrossRef.
- X.-D. Zhang, H. Wang, A. L. Antaris, L. Li, S. Diao, R. Ma, A. Nguyen, G. Hong, Z. Ma, J. Wang, S. Zhu, J. M. Castellano, T. Wyss-Coray, Y. Liang, J. Luo and H. Dai, Adv. Mater., 2016, 28, 6872–6879 CrossRef CAS PubMed.
- G. Hong, J. C. Lee, J. T. Robinson, U. Raaz, L. Xie, N. F. Huang, J. P. Cooke and H. Dai, Nat. Med., 2012, 18, 1841–1846 CrossRef CAS PubMed.
- G. Hong, J. T. Robinson, Y. Zhang, S. Diao, A. L. Antaris, Q. Wang and H. Dai, Angew. Chem., Int. Ed., 2012, 51, 9818–9821 CrossRef CAS PubMed.
- R. A. Revia and M. Zhang, Mater. Today, 2016, 19, 157–168 CrossRef CAS PubMed.
- E. T. Ahrens, U. Rothbächer, R. E. Jacobs and S. E. Fraser, Proc. Natl. Acad. Sci. U. S. A., 1998, 95, 8443–8448 CrossRef CAS.
- S. Wilhelm, A. J. Tavares, Q. Dai, S. Ohta, J. Audet, H. F. Dvorak and W. C. W. Chan, Nat. Rev. Mater., 2016, 1, 16014 CrossRef CAS.
- A. Verma and F. Stellacci, Small, 2010, 6, 12–21 CrossRef CAS PubMed.
- O. Shimoni, Y. Yan, Y. Wang and F. Caruso, ACS Nano, 2013, 7, 522–530 CrossRef CAS PubMed.
- P. Kolhar and S. Mitragotri, Adv. Funct. Mater., 2012, 22, 3759–3764 CrossRef CAS.
- J. A. Champion, Y. K. Katare and S. Mitragotri, J. Controlled Release, 2007, 121, 3–9 CrossRef CAS PubMed.
- K. Yang and Y.-Q. Ma, Nat. Nanotechnol., 2010, 5, 579–583 CrossRef CAS PubMed.
- R. Vácha, F. J. Martinez-Veracoechea and D. Frenkel, Nano Lett., 2011, 11, 5391–5395 CrossRef PubMed.
- S. E. A. Gratton, P. A. Ropp, P. D. Pohlhaus, J. C. Luft, V. J. Madden, M. E. Napier and J. M. DeSimone, Proc. Natl. Acad. Sci. U. S. A., 2008, 105, 11613–11618 CrossRef CAS PubMed.
- N. Doshi, B. Prabhakarpandian, A. Rea-Ramsey, K. Pant, S. Sundaram and S. Mitragotri, J. Controlled Release, 2010, 146, 196–200 CrossRef CAS PubMed.
- J. F. Alexander, V. Kozlovskaya, J. Chen, T. Kuncewicz, E. Kharlampieva and B. Godin, Adv. Healthcare Mater., 2015, 4, 2657–2666 CrossRef CAS PubMed.
|
This journal is © The Royal Society of Chemistry 2018 |
Click here to see how this site uses Cookies. View our privacy policy here.