DOI:
10.1039/D4TB00934G
(Paper)
J. Mater. Chem. B, 2024,
12, 8376-8382
An excimer process induced a turn-on fluorescent probe for detection of ultra-low concentration of mercury ions†
Received
30th April 2024
, Accepted 20th July 2024
First published on 24th July 2024
Abstract
The accumulation of mercury pollution in plants can induce severe injury to human beings. It is a great challenge to monitor ultra-low concentrations of mercury in complicated matrixes. In this study, we successfully developed a strategy via Hg2+-triggered naphthalene-based fluorescent probe 1, which formed excimer that subsequently emitted fluorescence for the successful detection of ultra-low concentrations of Hg2+. The coordination of N and S atoms with Hg2+ facilitated the formation of excimer from the naphthalene-conjugated planes that were in sufficiently close proximity. Suppression of C
N bond rotation also induced the chelation-enhanced fluorescence (CHEF) effect, and the cumulative result of these effects was obvious fluorescent enhancement. Compared with probe 2, the other key factor for detection of Hg2+ is that the electrons of the hydroxyl group can easily transfer to a naphthalene moiety, resulting in an augmented π-electron density that enhanced the π–π stacking of the naphthalene-conjugated excimer. After detailed spectral studies and mechanism discussions, it was realized that probe 1 was able to detect ultra-low concentrations of Hg2+ in PBS buffer solution. The detection limit was calculated to be 1.98 nM. On account of the excellent performances, the probe was successfully applied in monitoring Hg2+ in water and pea sprouts with the potential for application as an advanced warning of contamination.
Introduction
Mercury is widely distributed in the natural environment and exists in various forms. Volcanic eruptions, seismic activity, geological exploitation, gold mining, and oil refining are always accompanied by mercury contamination. The average mercury concentration was calculated to be more than 100 mg kg−1 in industrial and mining areas, which is higher than the background value of 0.4 mg kg−1.1 Potential leakage risks also originate from a variety of supplies used on a daily basis, ranging from preservatives in vaccines, dental consumables, thermometers, and high-pressure mercury lamps.2,3 Mercury exposure is prevalent in daily life through inhaled air, drinking water, and food. Mercury does not degrade, and because it continuously accumulates in biological systems, the potential risk for contamination appears to be very serious and deserves adequate attention.4,5
Quantitative and qualitative measurements are necessary to accurately assess mercury pollution.6,7 Efficient methods for mercury detection should be highly sensitive and selective to distinguish mercury from complicated matrixes. Commonly used methods are colorimetric analysis, atomic absorption spectrometry, and gas chromatography–mass spectrometry.8 However, these methods require sophisticated procedures and expensive instruments, and they are not suitable for real-time in situ visual detection. In this respect, fluorescent probes have become powerful monitoring tools due to their excellent properties, such as small size, easy modification, high selectivity and sensitivity, perfect solubility and biocompatibility, non-invasiveness, and real-time in situ detection with high spatial and temporal resolution.9–14
Hundreds of fluorescent probes have been designed and used for Hg2+ monitoring based on diverse detection mechanisms.15–22 However, excimer formation-induced fluorescent changes for Hg2+ are rare. Intramolecular or intermolecular excimers are mainly constructed with increased π–π electron stacking by fixing two or more planar chromophores at close distances. Besides, excimers can form in concentrated solutions that induce the interaction of neighboring molecules.23,24 Excimer-based fluorescent probes can provide built-in self-calibration for signal correction according to modulated monomer and excimer emission.25–27
The conformations of polycyclic aromatic hydrocarbons such as naphthalene, anthracene, naphthalimide, perylene, pyrene, and analogous derivatives are easily modulated with varying optical properties,28–38 although certain disadvantages such as the solubility and short emission wavelength more or less limit their biological application.39,40 In addition, sensitive detection of Hg2+ directly in aqueous solutions is difficult due to the pronounced hydration effects of Hg2+. Amongst these aromatic fluorophores, naphthalene provides an excellent platform for functionalized design of fluorescent probes that will recognize specific substrates.41–44 Facile modification of hydrophilic groups is essential to increase water solubility. Because of obvious advantages such as stable optical properties and selectivity, it is feasible for widespread development in different domains.
In this study, two fluorescent probes (1 and 2) were successfully synthesized by modifying mercaptoethylamine on a naphthalene fluorophore. The thiol and Schiff base moieties in probe 1 drive the affinity coordination between S, N, and Hg2+, triggering the fluorophores to be situated in sufficiently close proximity to yield excimer. The C
N group acting as a molecular rotor was restricted, and the inhibited isomerization mechanism induced turn-on fluorescence in the presence of Hg2+.45,46 Lone pairs of electrons of hydroxyl groups transferred to the naphthalene moiety, which also resulted in an augmented π-electron density that triggered π–π electron stacking. These interactions further tuned the emissions from monomer to excimer simultaneously, with obvious fluorescence enhancement.
Combining the solubility advantages of hydroxyl and thiol groups, this probe exhibited turn-on emission in the presence of ultra-low concentrations of Hg2+ in PBS buffer solution. The spectral spectroscopies were investigated involving sensitivity and selectivity testing, and the detection mechanism was identified by mass spectra. probe 1 was finally applied for monitoring real water samples and imaging the roots of pea sprouts.
Results and discussion
Spectral studies of probe 1
probe 1 and probe 2 were synthesized using the following procedures (Scheme 1) and were fully characterized by NMR and MS spectra, which appears in the ESI† (Fig. S1–S6). Having obtained probe 1, fluorescence spectral studies were performed for Hg2+ monitoring in different solutions. The fluorescence emission remained stable in organic solvents, including DMF, DMSO, THF, CH2Cl2, C2H5OH, and CH3OH (Fig. S7, ESI†), while a slight fluctuation was exhibited in PBS buffer solution (Fig. 1). The phenomenon was ascribed to the self-assembly process of probe 1 in PBS buffer to form an excimer with the assistance of hydrogen bonds of sulfydryl and hydroxyl groups, which was suggested by mass spectrum (Fig. S3, ESI†).
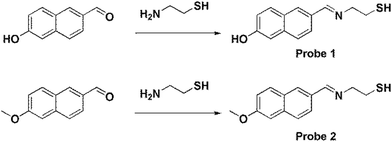 |
| Scheme 1 Synthesis routes for probe 1 and probe 2. | |
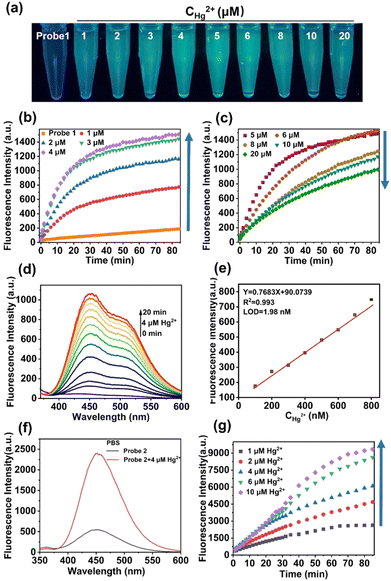 |
| Fig. 1 Spectral studies of probe 1 and probe 2 with Hg2+. (a) Fluorescence photographs of probe 1 with different concentrations of Hg2+ under 365 nm UV light irradiation. (b) and (c) Time-dependent and concentration-dependent fluorescence spectra of probe 1 (10 μM) in the presence of 1 μM, 2 μM, 3 μM, 4 μM, 5 μM, 6 μM, 8 μM, 10 μM, and 20 μM of Hg2+ in PBS buffer solution. (d) Time-dependent fluorescence spectra of probe 1 (10 μM) in the presence of Hg2+ (4 μM) in PBS buffer solution. (e) The curve of F450versus Hg2+ concentration in the range of 100 to 800 nM, indicating a good linear relationship. (f) The fluorescent response of probe 2 (10 μM) with Hg2+ (4 μM) in PBS buffer solution. (g) Time-dependent and concentration-dependent fluorescence spectra of probe 2 (10 μM) in the presence of 1 μM, 2 μM, 4 μM, 6 μM, and 10 μM of Hg2+ in PBS buffer solution. | |
After addition of Hg2+, an obvious fluorescent enhancement was observed in PBS buffer solution because of the coordination of probe 1 with Hg2+ that triggered the aggregation and excimer formation for fluorescence enhancement, and the ratio of fluorescence intensity in the presence and absence of Hg2+ was about 10.4-fold. The fluorescence intensity was nearly unchanged in other solutions (Fig. S7h, ESI†). In comparison, there was greater dispersion of coordination compounds in organic solvents, which prevented aggregation as compared to that in PBS buffer solution, and resulted in no fluorescence change. The detection procedures can also be conducted under different pH values, ranging from 5.8 to 8.0 (Fig. S8, ESI†).
The time-dependent and concentration-dependent fluorescence responses of probe 1 in the presence of Hg2+ were determined (Fig. 1a–d). The fluorescence intensity dramatically increased upon addition of Hg2+ at the first 4 μM. Along with extended time (Fig. 1b), and then decreased with increasing concentrations from 5 μM to 20 μM (Fig. 1c). Moreover, visible colorimetric detection was applied under 365-nm excitation with the same tendency. The color changed from colorless to deep green as the concentration of Hg2+ increased from 1 μM to 4 μM, whereas it weakened to light green from 5 μM to 20 μM (Fig. 1a). The fluorescence intensity attained equilibrium as the time reached about 30 min (Fig. 1b). The detailed fluorescent spectral changes exhibited by probe 1 with Hg2+ (4 μM) were also recorded (Fig. 1d). The fluorescence intensities at 450 nm exhibited a strong linear correlation with Hg2+ in the concentration range from 100 nM to 800 nM (Fig. 1e).
The limit of detection (LOD) was calculated to be 1.98 nM, which was greatly improved compared with the reported fluorescent probes in Table S1 (ESI†). The quantum yields of probe 1 and probe 1-Hg2+ were calculated to be 4.1% and 8.2%, respectively (Fig. S9a, ESI†). The quantitative fluorescence lifetimes of probe 1 and probe 1-Hg2+ were 1.6631 and 1.6698, respectively (Fig. S10a and b, ESI†). UV-Vis spectra in the absence and presence of Hg2+ were also obtained using an absorption band at 320 nm increased over time (Fig. S11, ESI†).
The fluorescent changes of probe 1 towards Hg2+ is a puzzling phenomenon and may be related to the reason for the high sensitivity of probe 1 for ultra-low concentration detection of Hg2+. To elucidate this phenomenon, contrast probe 2 was synthesized for comparison. Parallel fluorescent and UV-Vis spectra experiments with probe 2 were carried out, and Hg2+ detection was feasible in PBS solution (Fig. S12 and S13, ESI† and Fig. 1f). The time-dependent and concentration-dependent fluorescence responses of probe 2 in the presence of Hg2+ were then determined.
As shown in Fig. 1g, the fluorescent intensity of probe 2 in response to Hg2+ was enhanced as the concentration of Hg2+ increased and over extended time. The quantum yields of probe 2 and probe 2-Hg2+ were calculated to be 10.9% and 17.6%, respectively (Fig. S9b, ESI†). The quantitative fluorescence lifetimes of probe 2 and probe 2-Hg2+ were 5.3900 and 6.2103, respectively (Fig. S10c and d, ESI†). However, this was different from the fluorescent trends of probe 1 (Fig. 1b and c).
Hence, mass spectroscopy was further performed to clarify the response mechanism. According to the spectral studies, the fluorescence intensity of probe 1 and Hg2+ reached the maximum with a concentration ratio of 2
:
1 (Fig. 1b). Therefore, the electrospray ionization-mass spectrometry (ESI-MS) spectra of the probe-Hg2+ coordinated complexes with a ratio of 2
:
1 were measured. As shown in Fig. 2, the coordination mode of probe 1 and probe 2 with Hg2+ was confirmed to be 2
:
1, as suggested by their values of m/z 663.22 and 691.29, respectively (Fig. 2c and d).
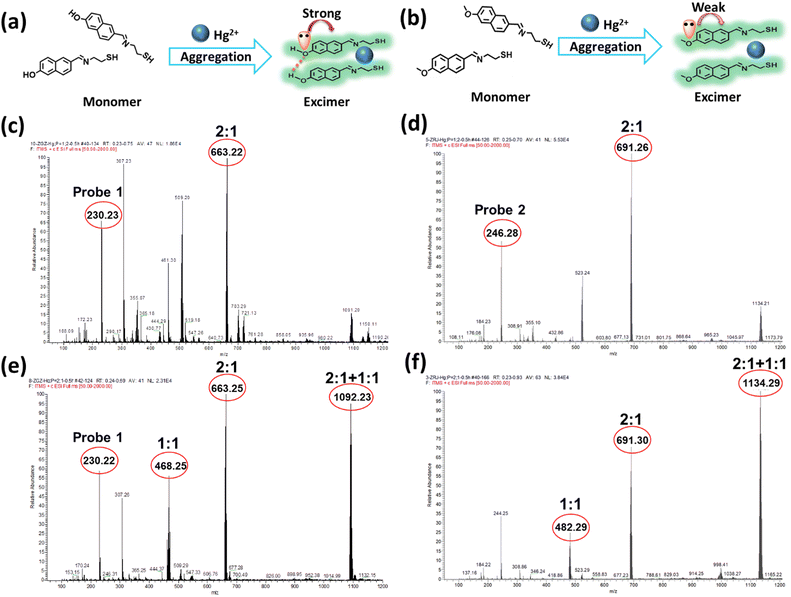 |
| Fig. 2 The coordination mechanisms between probes 1 and 2 with Hg2+. (a) and (b) Schematic diagrams of the excimer formation process of probe 1 and probe 2 with Hg2+. (c) and (d) Mass spectra of the probe 1-Hg2+ (2 : 1) complex and probe 2-Hg2+ (2 : 1) complex. (e) Mass spectra of the probe 1-Hg2+ (1 : 2) complex (m/z 468.25 was assigned to a 1 : 1 complex, m/z 663.25 was assigned to a 2 : 1 complex, and m/z 1092.23 was assigned to a 2 : 1+1 : 1 complex). (f) The mass spectrum of the probe 2-Hg2+ (1 : 2) complex (m/z 482.29 was assigned to a 1 : 1 complex, m/z 691.30 was assigned to a 2 : 1 complex, and m/z 1134.29 was assigned to a 2 : 1+1 : 1 complex). | |
In this case, the complexes of probe 1-Hg2+ and probe 2-Hg2+ mainly existed in a 2
:
1 ratio, and both of them were formed as excimer. However, when the probes and Hg2+ were mixed at the ratio of 1
:
2 (Fig. 2e and f), new peaks at 1092.23 and 1134.29 appeared for the probe 1-Hg2+ and probe 2-Hg2+ complexes, respectively. Different ratios of the probe-Hg2+ complexes were formed, and the excimer would be destroyed as the concentration of Hg2+ increased. probe 1 and probe 2 exhibited the same trends in the mass spectra of their complexes. All the results indicated that Hg2+ possessed the ability to trigger naphthalene-based fluorescent probes so they would form excimer and subsequently emit fluorescence, but this does not adequately explain the changes in fluorescence.
For structural analysis of the two probes, the only difference was in their different substituted groups—the hydroxyl group and methoxyl group. The Hammett substituent constants provide direct evidence of the higher electron-donating abilities of the hydroxyl group (−0.37) as compared to the methoxyl group (−0.27).47 In probe 1, the lone pair of electrons in the hydroxyl group was intensively transferred to the naphthalene moiety, resulting in an augmented π-electron density that triggered the π–π electron stacking of the naphthalene conjugation and further facilitated the coordination of N and S atoms with Hg2+. The enhancement of the fluorescence intensity was attributed to the formation of excimer with the extensive conjugated plane of naphthalene, as well as the rotation suppression of the C
N bond and the chelation enhanced fluorescence (CHEF) effect.
Density functional theory (DFT) studies were carried out to elucidate the possible mechanism. probe 2 exhibited a higher energy gap between the HOMO–LUMO orbitals than probe 1, which was unfavorable for coordination with mercury ions due to its lower reactivity (Fig. S14a, ESI†). We also investigated the electron loss abilities of the naphthalene portion in two probes (Fig. S14b and Table S2, ESI†). The results (loss of 0.18442e in probe 1 and loss of 0.18695e in probe 2) proved that the methoxyl group impeded the electron transfer to naphthalene, which is unfavorable for the formation of excimer. Besides, the lack of a hydrogen bond in the π–π stacking mode of probe 2 directly weakened the stacking ability (Fig. S14c, ESI†). Therefore, the methoxyl group impeded the electron transfer to naphthalene and then weakened the π–π electron stacking, which is unfavorable for the formation of excimer. Moreover, the hydrogens in probe 1 were shifted to a field that was higher than that of probe 2 (Fig. S1 and S4, ESI†), which was derived from the stronger π–π stacking that increased the charge densities and further weakened the vibration of hydrogen. Based on these studies, it was determined that probe 1 is more sensitive to low concentrations of Hg2+ in aqueous solutions, which is beneficial for bilateral fixation by hydrogen bond and mercury coordination to facilitate excimer formation as well as lone pair electrons to enhance the π–π electron stacking of the naphthalene conjugation.
To obtain insight into the fluorescent performance in a complicated matrix, selectivity studies were investigated in the presence of interfering metal ions (100 μM), including Cu2+, Mg2+, Fe3+, Fe2+, Cd2+, K+, Cr3+, Ba2+, Na+, Mn2+, Co2+, Ca2+, Ni2+, Al3+, Cu+, Zn2+, and Ag+. As shown in Fig. 3, colorimetric detection under a 365-nm UV lamp was performed. probe 1 (10 μM) was pre-introduced in PBS solution, and there were no obvious fluorescence color changes upon addition of these interfering metal ions except for Ag+ and Hg2+. A slight green color and weak enhancement was exhibited after addition of Ag+, as shown in (Fig. S15, ESI†), whereas a significant color transformation from colorless to deep green emerged upon addition of Hg2+. The fluorescence intensities were further compared with each other, as shown in Fig. 3b and c, and only Hg2+ induced obvious emission enhancement (black bars), which increased to different extents in the presence of interfering metal ions (red bars). Therefore, probe 1 exhibited high selectivity and sensitivity for the detection of Hg2+ in PBS buffer solution.
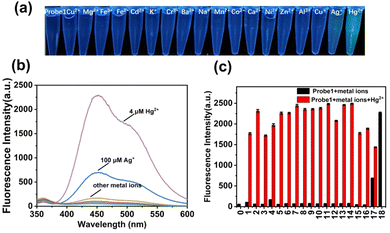 |
| Fig. 3 (a) Fluorescence photograph of probe 1 with different metal ions under 365-nm UV light irradiation. (b) Fluorescence spectra of probe 1 with Hg2+ (4 μM) and other different metal ions (100 μM) in PBS buffer solution. (c) Relevant column diagram for selectivity studies of fluorescence probe 1 with different metal ions (black bars, 0: probe 1, 1–18: Cu2+, Mg2+, Fe3+, Fe2+, Cd2+, K+, Cr3+, Ba2+, Na+, Mn2+, Co2+, Ca2+, Ni2+, Zn2+, Al3+, Cu+, Ag+, and Hg2+) and following the addition of Hg2+ (red bars) in PBS buffer solution (10 mM, pH 7.4). (λex = 320 nm, λem = 450 nm). | |
Determination of Hg2+ in real water samples
Having confirmed the excellent performances of the probes, probe 1 was selected and then applied to evaluate the applicability of detection in real water samples, including tap water, ultrapure water, and lake water. As presented in Table 1, the determination of Hg2+ at ultra-low concentrations was conducted according to the standard addition method, and the recovery rates ranged from 91% to 108%. Therefore, probe 1 can be effectively applied to measure Hg2+ in environmental water samples.
Table 1 Determination of Hg2+ in real water samples
Sample |
Added (nM) |
Found (nM) and RSD (n = 3) |
Recovery (%) |
Tap water |
0 |
Not detected |
— |
200 |
215.58 ± 0.97 |
108 |
500 |
540.29 ± 0.23 |
108 |
800 |
810.27 ± 0.40 |
101 |
Ultrapure water |
0 |
Not detected |
— |
200 |
210.27 ± 1.36 |
105 |
500 |
464.81 ± 0.31 |
93 |
800 |
744.51 ± 0.06 |
93 |
Lake water |
0 |
Not detected |
— |
200 |
181.20 ± 0.29 |
91 |
500 |
507.00 ± 0.61 |
101 |
800 |
791.92 ± 0.29 |
99 |
Imaging of Hg2+ in pea sprouts
As mentioned above, mercury can accumulate in the food chain, and plants are a key link because they act as repositories for mercury from the soil. Detection of Hg2+ in plants not only enables visualization of mercury distribution and bioaccumulation in plants, but also facilitates the use of effective methods to avoid mercury damage. Hence, pea sprouts were employed as a plant model for fluorescent imaging. Four-day-old pea sprouts were incubated with probe 1 for 12 hours, and then incubated with mercury for another 12 hours. The longitudinal and transverse root sections were then imaged under a 10x objective lens. Fig. 4 shows that the fluorescent emissions were quite weak when the tissues were only incubated with probe 1. Once Hg2+ was added, it was transferred into the plant tissues through plant veins and exhibited bright fluorescent emission. The results indicate that probe 1 was capable of visualization imaging of mercury in plants.
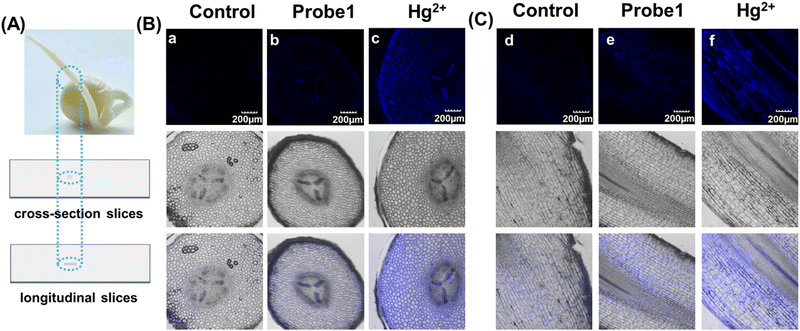 |
| Fig. 4 Fluorescence imaging of probe 1 for Hg2+ determination in pea sprouts. (A) Photograph of a pea sprout and schematic diagrams of root slices. (B) Fluorescence imaging of cross-section slices of pea sprouts. (a) Blank group. (b) Slices of pea sprouts incubated with probe 1 (10 μM) for 12 h. (c) Slices of pea sprouts incubated with probe 1 (10 μM) for 12 h and then treated with Hg2+ (20 μM) for 12 h. (C) Fluorescence imaging of longitudinal slices of pea sprouts. (d) Blank group. (e) Slices of pea sprouts incubated with probe 1 (10 μM) for 12 h. (f) Slices of pea sprouts incubated with probe 1 (10 μM) for 12 h and then treated with Hg2+ (20 μM) for 12 h. Scale bars = 200 μm (λex = 405 nm, λem = 400–500 nm). | |
Experimental
Materials and instruments
All chemical reagents were analytical grade and purchased from commercial sources, and were used without further purification. Fluorescent probes were characterized by NMR and ESI-MS spectra using a Bruker AVANCE IIITM 500 spectrometer and a Thermo LCQ Fleet mass spectrometer, respectively. Fluorescent spectral studies were recorded on a fluorescence spectrophotometer (HITACHI F-4700). The mechanisms of probe coordination with Hg2+ were confirmed by mass spectra. Fluorescence imaging of slices of pea sprouts was obtained using an Olympus fluorescent microscope (FluoView FV1000). DFT calculations were carried out by the Gaussian 16 program packages. All structures were fully optimized at the M06-2X+GD3/def2-TZVP level. Natural atomic charges were generated using the NBO 6.0 program.
Spectral analysis
The spectral analysis was conducted in phosphate-buffered saline (PBS, 10 mM, pH 7.4). The stock solutions of probes (10 mM and 1 mM) were prepared using analytically pure dimethyl sulfoxide (DMSO) and stored at 4 °C, and then diluted to a working concentration (10 μM, 0.1% DMSO) with PBS buffer solution. Deionized water was used to prepare 10 mM individual stock solutions of Cu2+, Mg2+, Fe3+, Fe2+, Cd2+, K+, Cr3+, Ba2+, Na+, Mn2+, Co2+, Ca2+, Ni2+, Al3+, Cu+, Zn2+, Ag+, and Hg2+. Selective and competitive experiments of probe 1 (10 μM) in the presence of Hg2+ (4 μM) and with potential interference (100 μM) were conducted in PBS buffer solution after 60 minutes. The lake water, tap water, and ultrapure water were mixed with PBS buffer solution (1
:
19, v/v) and then assessed with working concentrations of probe 1 (10 μM) and Hg2+ (0 to 800 nM).
Preparation of the slices of pea sprouts
Pea seeds were sterilized with ethanol and cultured in ultrapure water medium at room temperature. Roots of four-day-old pea sprouts were incubated with probe 1 (10 μM) for 12 hours and Hg2+ (20 μM) for 12 hours in cascade. After thoroughly washing with ultrapure water, the roots (mature zone) were embedded in 4% low melting point agarose. Longitudinal and transverse cutting was implemented using a vibrating microtome (VT1200S, Leica), and the roots were cut into semi-thin sections of 100-μm thickness. The sections were placed on microscope slides, infiltrated with PBS, and then covered with a coverslip. Fluorescence imaging for slices of pea sprouts in longitudinal and transverse cutting forms was performed, and the sprouts were observed under an Olympus fluorescence microscope with a 10× objective lens. The excitation wavelength was at 405 nm, and the collecting wavelengths were from 400 to 500 nm.
Synthesis of probe 1
In a 50-mL round-bottomed flask, 6-hydroxy-2-naphthaldehyde (172 mg, 1 mmol) and mercaptoethylamine (77 mg, 1 mmol) were dissolved in 5 mL anhydrous ethanol under stirring, and then refluxed for 30 min. The reaction progress was monitored by thin-layer chromatography (TLC). At the end of the reaction, the crude product was filtered to obtain a white solid (probe 1) with a yield of 58%. 1H NMR (500 MHz, DMSO-d6) δ 9.75 (s, 1H), 7.81 (s, 1H), 7.72 (d, J = 8.7 Hz, 1H), 7.65 (d, J = 8.6 Hz, 1H), 7.47 (dd, J = 8.6, 1.6 Hz, 1H), 7.16–6.99 (m, 2H), 5.58 (s, 1H), 3.52 (ddd, J = 11.4, 5.1, 3.4 Hz, 1H), 3.08–2.81 (m, 3H). 13C NMR (126 MHz, DMSO-d6) δ 155.48, 134.86, 134.13, 129.35, 127.19, 126.13, 125.77, 125.41, 118.84, 108.61, 73.43, 52.88, 36.31. ESI-MS [C13H13NOS]+, calcd. 231.07, found [M+1]+: 232.19.
Synthesis of probe 2
In a 50-mL round-bottomed flask, 6-methoxy-2-naphthaldehyde (186 mg, 1 mmol) and mercaptoethylamine (116 mg, 1.5 mmol) were dissolved in 5 mL anhydrous ethanol under stirring, and then refluxed for 30 min. The reaction was monitored by TLC. At the end of the reaction, the crude product was filtered to obtain a white solid (probe 2) with a yield of 60%. 1H NMR (500 MHz, DMSO-d6) δ 7.88 (s, 1H), 7.83–7.73 (m, 2H), 7.54 (dd, J = 8.5, 1.7 Hz, 1H), 7.30 (d, J = 2.4 Hz, 1H), 7.16 (dd, J = 8.9, 2.6 Hz, 1H), 5.61 (s, 1H), 3.87 (s, 3H), 3.57–3.44 (m, 1H), 3.11–2.85 (m, 3H). 13C NMR (126 MHz, DMSO-d6) δ 157.37, 135.94, 133.81, 129.31, 127.99, 126.78, 126.04, 125.34, 118.78, 105.84, 73.28, 55.19, 52.87, 36.34. ESI-MS [C14H15NOS]+, calcd. 245.34, found [M+1]+: 246.22.
Conclusions
In summary, we successfully constructed naphthalene-based fluorescent probe 1 for detection of ultra-low concentrations of mercury. We synthesized contrast probe 2 to use fluorescence and mass spectra to deeply study the response mechanism, which can be ascribed to three key factors. First, the sulfhydryl and C
N group of the Schiff base were responsible for the coordination between probes and Hg2+, which was conducive to inducing excimer formation and then subsequent turn-on fluorescence emission. Second, the hydroxyl group of probe 1 formed an intermolecular hydrogen bond, but this could not occur in probe 2. The bilateral fixation by hydrogen bond and coordination further enhanced the stability of the excimer. Third, the key factor for fluorescence enhancement of probe 1 was speed, because the lone pair of electrons of the hydroxyl group could easily transfer to the naphthalene moiety, resulting in an augmented π-electron density that further enhanced the π–π electron stacking. For these reasons, probe 1 is more sensitive to low concentrations of Hg2+ in aqueous solutions, with a calculated detection limit of 1.98 nM. Hence, probe 1 was successfully applied to detect Hg2+ in water samples and pea sprouts. This holds significant importance for the determination of mercury contamination and bioaccumulation, with potential application for contamination warning in advance.
Author contributions
Shujing Fang: investigation, writing – original draft. K. M. K. Swamy: investigation. Wen-Yan Zan: writing – review and editing. Juyoung Yoon: conceptualization, writing – review and editing. Shudi Liu: conceptualization, writing – original draft, writing – review and editing.
Data availability
The data supporting this article have been included as part of the ESI.†
Conflicts of interest
There are no conflicts to declare.
Acknowledgements
This work was financially supported by the Natural Science Foundation of China (21701074). J. Yoon thanks the National Research Foundation (NRF) funded by the Korean government (MSIT) (2022R1A2C3005420 and 2020R1A6C101B194). K. M. K. Swamy thanks the Brain Pool program funded by the Ministry of Science and ICT through the National Research Foundation of Korea (RS-2023-00304224).
Notes and references
- B. Gao, L. Han, H. Hao and H. Zhou, Ecol. Indic., 2016, 67, 577–585 CrossRef CAS.
- K. G. Pavithra, P. SundarRajan, P. S. Kumar and G. Rangasamy, Chemosphere, 2023, 312, 137314 CrossRef CAS PubMed.
- T. W. Clarkson, L. Magos and G. J. Myers, N. Engl. J. Med., 2003, 349, 1731–1737 CrossRef CAS PubMed.
- T. W. Clarkson, Crit. Rev. Clin. Lab. Sci., 2008, 34, 369–403 CrossRef PubMed.
- T. W. Clarkson and L. Magos, Crit. Rev. Toxicol., 2006, 36, 609–662 CrossRef CAS PubMed.
- S. Liu, X. Zhang, C. Yan, P. Zhou, L. Zhang, Q. Li, R. Zhang, L. Chen and L. Zhang, J. Hazard. Mater., 2022, 424, 127701 CrossRef CAS PubMed.
- Y. Wang, L. Zhang, X. Han, L. Zhang, X. Wang and L. Chen, Chem. Eng. J., 2021, 406, 127166 CrossRef CAS.
- T. A. Saleh, G. Fadillah, E. Ciptawati and M. Khaled, TrAC, Trends Anal. Chem., 2020, 132, 116016 CrossRef CAS.
- J. Yin, L. Huang, L. Wu, J. Li, T. D. James and W. Lin, Chem. Soc. Rev., 2021, 50, 12098–12150 RSC.
- X. Qian and Z. Xu, Chem. Soc. Rev., 2015, 44, 4487–4493 RSC.
- J. Du, M. Hu, J. Fan and X. Peng, Chem. Soc. Rev., 2012, 41, 4511–4535 RSC.
- S. Liu, L. Zhang, H. Kim, J. Sun and J. Yoon, Coord. Chem. Rev., 2024, 501, 215575 CrossRef CAS.
- D. Rajput, N. Pradhan, S. Mansuri, V. Soppina and S. Kanvah, J. Mater. Chem. B, 2024, 12, 4698–4707 RSC.
- Z. L. Li, A. X. Ma, J. Q. Liu, K. Wang, B. C. Zhu, D. W. Pang and D. M. Kong, J. Mater. Chem. B, 2024, 12, 4398–4408 RSC.
- R. AbhijnaKrishna and S. Velmathi, Coord. Chem. Rev., 2022, 459, 214401 CrossRef CAS.
- H. Shuai, C. Xiang, L. Qian, F. Bin, L. Xiaohui, D. Jipeng, Z. Chang, L. Jiahui and Z. Wenbin, Dyes Pigm., 2021, 187, 109125 CrossRef CAS.
- S.-Y. Chen, Z. Li, K. Li and X.-Q. Yu, Coord. Chem. Rev., 2021, 429, 213691 CrossRef CAS.
- S. Liu, D. Feng, L. Zhang, H. Song, Y. Wang, X. Zhang, Q. Zhao and L. Chen, Spectrochim. Acta, A, 2020, 243, 118817 CrossRef CAS PubMed.
- E. M. Nolan and S. J. Lippard, Chem. Rev., 2008, 108, 3443–3480 CrossRef CAS PubMed.
- A. Kumar, Virender, M. Saini, B. Mohan, Shayoraj and M. Kamboj, Microchem. J., 2022, 181, 107798 CrossRef CAS.
- S. Fang, L. Zhang, Y. Zhao, X. Zhang, L. Zhang, L. Chen, J. Yoon and S. Liu, Sens. Actuators, B, 2024, 411, 135768 CrossRef CAS.
- A. K. Jha, S. Umar, R. K. Arya, D. Datta and A. Goel, J. Mater. Chem. B, 2016, 4, 4934–4940 RSC.
- A. S. Belova, Y. N. Kononevich, D. S. Ionov, V. A. Sazhnikov, A. D. Volodin, A. A. Korlyukov, P. V. Dorovatovskii, M. V. Alfimov and A. M. Muzafarov, Dyes Pigm., 2023, 208, 110852 CrossRef.
- C. Wang, X. Yang, R. Duan, X. Li, Y. Liu, W. Liu, B.-G. He, S. Zhang, B. Yang, K. Wang, B. Zou, Y. Qi, Q. Li, H. Liu and Y. Dai, Dyes Pigm., 2024, 223, 111953 CrossRef CAS.
- Y. Chen, Molecules, 2022, 27, 8628 CrossRef CAS PubMed.
- Y. Chen, S. Zheng, M. H. Kim, X. Chen and J. Yoon, Curr. Opin. Chem. Biol., 2023, 75, 102321 CrossRef CAS PubMed.
- S. Zeng, X. Liu, Y. S. Kafuti, H. Kim, J. Wang, X. Peng, H. Li and J. Yoon, Chem. Soc. Rev., 2023, 52, 5607–5651 RSC.
- R. Long, C. Tang, Q. Wei, C. Tong, X. Tong, S. Shi, Y. Guo and Y. Yang, Sens. Actuators, B, 2021, 348, 130666 CrossRef CAS.
- J.-B. Li, Y. Liu, X.-J. Zheng and D. Wang, Microchem. J., 2019, 150, 104123 CrossRef CAS.
- S. Chatterjee, H. Gohil, I. Raval, S. Chatterjee and A. R. Paital, Small, 2019, 15, e1804749 CrossRef PubMed.
- Z. Xu, J. Yoon and D. R. Spring, Chem. Commun., 2010, 46, 2563–2565 RSC.
- J. Cui, H. Nie, X. Liang, J. Bai and X. Zhang, J. Mol. Liq., 2022, 366, 120325 CrossRef CAS.
- G. Han, D. Kim, Y. Park, J. Bouffard and Y. Kim, Angew. Chem., 2015, 54, 3912–3916 CrossRef CAS PubMed.
- Y. Wang, J. Chen, Y. Chen, W. Li and C. Yu, Anal. Chem., 2014, 86, 4371–4378 CrossRef CAS PubMed.
- S. Bhuin, P. Sharma, P. Chakraborty, O. P. Kulkarni and M. Chakravarty, J. Mater. Chem. B, 2023, 11, 188–203 RSC.
- X. Chen, K. H. Baek, Y. Kim, S. J. Kim, I. Shin and J. Yoon, Tetrahedron, 2010, 66, 4016–4021 CrossRef CAS.
- K. Inoue, R. Kawakami, M. Murakami, T. Nakayama, S. Yamamoto, K. Inoue, T. Tsuda, K. Sayama, T. Imamura, D. Kaneno, S. Hadano, S. Watanabe and Y. Niko, J. Mater. Chem. B, 2022, 10, 1641–1649 RSC.
- X. Gou, M. A. H. Nawaz, C. Liu, N. Yang, J. Ren, H. Zhou, Y. Li, J. Zhu, W. Han and C. Yu, J. Mater. Chem. B, 2022, 10, 5774–5783 RSC.
- L. Wang, C. Lou, M. Zhao, B. Zhao, H. Zhao, W. Ma, A. Wang, X. Wang, N. Wang and Y. Li, Inorg. Chem. Commun., 2021, 129, 108662 CrossRef CAS.
- T. Puangsamlee, Y. Tachapermpon, P. Kammalun, K. Sukrat, C. Wainiphithapong, J. Sirirak and N. Wanichacheva, J. Lumin., 2018, 196, 227–235 CrossRef CAS.
- X. J. Wang, G. W. Li, Y. P. Cheng, Q. L. Sun, Y. Q. Hao, C. H. Wang and L. T. Liu, Front. Chem., 2022, 10, 813108 CrossRef CAS PubMed.
- Y. Gao, H. Liu, S. Zhang, Q. Gu, Y. Shen, Y. Ge and B. Yang, Phys. Chem. Chem. Phys., 2018, 20, 12129–12137 RSC.
- X. Wu, N. Duan, S. Yang, H. Tian and B. Sun, ChemistrySelect, 2020, 5, 1683–1687 CrossRef CAS.
- X. Wu, N. Duan, Y. Li, S. Yang, H. Tian and B. Sun, J. Photochem. Photobiol., A, 2020, 388, 112209 CrossRef.
- B. Musikavanhu, Y. Liang, Z. Xue, L. Feng and L. Zhao, Molecules, 2023, 28, 6960 CrossRef CAS PubMed.
- N. Kumari, S. Singh, M. Baral and B. K. Kanungo, J. Fluoresc., 2023, 33, 859–893 CrossRef CAS PubMed.
- C. Hansch, A. Leo and R. W. Taft, Chem. Rev., 2002, 91, 165–195 CrossRef.
|
This journal is © The Royal Society of Chemistry 2024 |