DOI:
10.1039/D3NR06102G
(Minireview)
Nanoscale, 2024,
16, 8791-8806
Unleashing the potential: integrating nano-delivery systems with traditional Chinese medicine
Received
30th November 2023
, Accepted 23rd February 2024
First published on 26th February 2024
Abstract
This review explores the potential of integrating nano-delivery systems with traditional Chinese herbal medicine, acupuncture, and Chinese medical theory. It highlights the intersections and potential of nano-delivery systems in enhancing the effectiveness of traditional herbal medicine and acupuncture treatments. In addition, it discusses how the integration of nano-delivery systems with Chinese medical theory can modernize herbal medicine and make it more readily accessible on a global scale. Finally, it analyzes the challenges and future directions in this field.
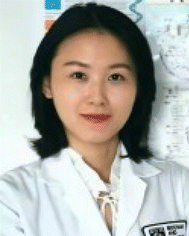 Na Kong | Prof. Na Kong is a Professor at Liangzhu Laboratory, Zhejiang University. She received her MD in oncology from Zhejiang University and completed her postdoctoral training at Brigham and Women's Hospital, Harvard Medical School. Her research interests majorly focus on biomaterials and nanomedicine. |
1. Introduction
Traditional Chinese medicine (TCM) is a time-honored and ancient medical system that has persisted for millennia. TCM encompasses a wide range of treatment modalities, including herbal medicine, acupuncture, and dietary therapy. It is deeply rooted in the philosophy of balance and harmony, emphasizing the interconnectedness of the body, mind, and environment; it also emphasizes individualized treatment and holistic care, aiming not only to address symptoms, but also to target the underlying causes of diseases.1 In recent years, TCM, with its rich history and holistic approach to healing, has gained global recognition for its efficacy and safety. However, the clinical utilization of TCM and its natural active compounds in treating diverse ailments is hindered by several factors, including restricted administration methods, inadequate solubility, instability, brief biological half-life, limited targeting efficacy, vulnerability to metabolism, and rapid elimination.2,3
The progress of contemporary technology, specifically in the realm of nanotechnology, has presented novel prospects for enhancing the efficacy and precision of TCM therapies. Nanotechnology encompasses the manipulation and utilization of materials at the nanoscale, thereby facilitating the emergence of innovative strategies in drug delivery, diagnostics and therapeutics.4–6 The utilization of nanoscale delivery systems represents a cutting-edge field in pharmaceutical science, offering precise control over drug delivery and therapeutic outcomes.7 These delivery systems involve the use of nanoparticles or nanocarriers that exploit their unique nanoscale properties to encapsulate, transport, and release therapeutic agents with unprecedented precision. The multifunctionality of nano-delivery systems, including enhanced drug solubility, prolonged circulation time, and targeted delivery capabilities, has positioned them as the focal point of innovation in the pharmaceutical industry.
Over the past decade, the application of delivery systems has facilitated the development of Chinese herbal formulations and sparked interest in the synergistic interaction between TCM and modern medicine. Nano-delivery systems can improve the solubility and stability of bioactive compounds, prolong their circulation time in the body, and enable targeted delivery to specific tissues or organs. Integrating nanotechnology and TCM opens new avenues for advancing both fields and presents an exciting opportunity for synergistic collaboration.
In this minireview, we aim to explore the integration of these two distinct yet complementary fields, shedding light on potential synergistic effects and applications that arise from the integration of nano-delivery systems in the field of TCM (Fig. 1). We will discuss the intersection points and the potential of nano-delivery systems in enhancing the therapeutic effects of traditional herbal medicine and acupuncture interventions. Furthermore, we will introduce the fusion between the fundamental tenets of TCM and nano-delivery systems. Lastly, we will analyze the challenges and future trajectories within this amalgamated field. Overall, this minireview will provide valuable insights into the integration of nanotechnology and TCM, offering novel perspectives on the development of innovative therapeutic approaches that can improve patient outcomes and advance human health.
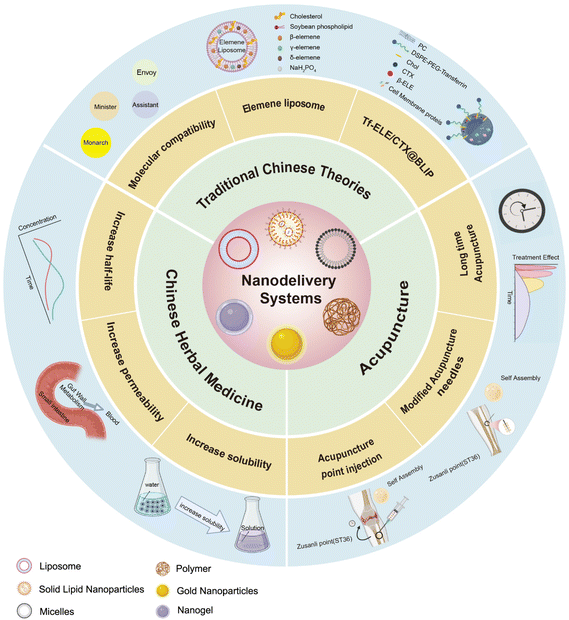 |
| Fig. 1 Schematic of integrating nano-delivery systems with traditional Chinese medicine (figure created with Biorender.com). | |
2. Bibliometric analysis
Bibliometric analysis has emerged as a powerful tool for quickly revealing research focal points and evolutionary trajectories within the field of nano-delivery systems in TCM.8 As depicted in Fig. 2A, a comprehensive examination of publications over the past decade using the Web of Science reveals a consistent and annual increase in the number of research publications related to nano-delivery systems in conjunction with TCM. Remarkably, during the global COVID-19 pandemic, TCM made enduring and substantial contributions to the scientific landscape, catalyzing collaborative research efforts worldwide and resulting in an exponential surge in related scholarly works. When it comes to publication output, China takes the lead, closely followed by India, Iran, and the United States, underscoring the global significance of nano-delivery applications in TCM (Fig. 2B). Furthermore, the correlation cluster analysis of one hundred PubMed-indexed articles highlights the importance of using nano-delivered Chinese herbal remedies in the treatment of tumors as a paramount research focus (Fig. 2C). This prioritization primarily arises from the current prominence of nanomedicine in the fields of cancer diagnosis and therapeutics, with nanomedicine leading the way in driving modern innovations within TCM.4,6,9
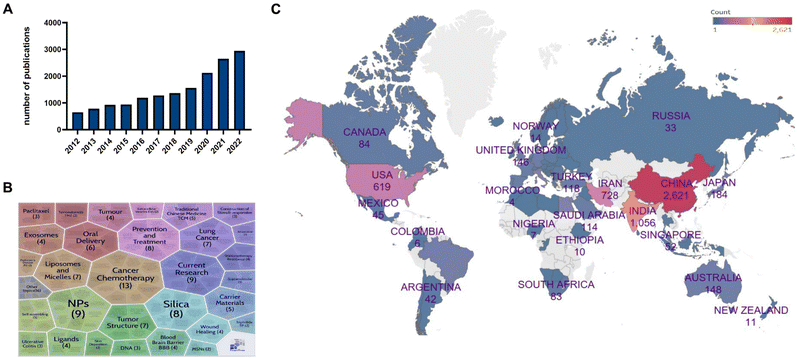 |
| Fig. 2 (A) The annual growth in the number of publications related to nanodelivery and TCM. (B) The countries/regions in the fields of nanodelivery and TCM with the biggest contributions. (C) The correlation cluster analysis, conducted using Carrot2 software, K-means algorithm, 30 classes, showing the main components of the integration of nanodelivery and TCM. | |
3. Basis of the integration of TCM and nano-delivery systems
While the evolutionary trajectories of nanodelivery and TCM exhibit apparent disparities, the intriguing convergence towards integration is noteworthy. This fusion signifies advanced interdisciplinary collaboration aimed at achieving more substantial outcomes in clinical treatment.
3.1 Development course of nano-delivery systems
Nanotechnology encompasses the manipulation and utilization of materials at the nanoscale, commonly defined as dimensions below 100 nanometers (nm). In the context of drug delivery, it offers a framework for the development and utilization of nanoscale materials and structures to transport drugs or other bioactive substances to specific targets in vivo.10,11 The initial nano-delivery system, known as liposomes, was initially documented in 1964.12 Following this discovery, a multitude of drug delivery systems based on organic or inorganic materials have subsequently been developed. In 1976, the advent of the initial controlled-release polymer delivery system for administering large molecules marked a significant milestone. Furthermore, in 1980, a more intricate drug delivery system capable of responding to pH alterations to initiate drug release was elucidated. In addition, in 1987, the pioneering development of long-circulating liposomes, subsequently referred to as “stealth liposomes”, occurred.12–14 Subsequent investigations unveiled the ability of polyethylene glycol (PEG) to prolong the circulation duration of lipid nanoparticles and polymer nanoparticles.15,16 The aforementioned developments have played a significant role in enabling the market success of pharmaceuticals such as Doxil and the nanobody-based drug CabiliviTM. Moreover, these advancements ultimately facilitated the successful introduction of COVID-19 mRNA vaccines, which are encapsulated in lipid nanoparticles (LNPs), during the COVID-19 pandemic.17,18 Consequently, the continuous progress in nano-delivery systems has elevated nanomedicine to make it a prominent and captivating area of contemporary research.
3.2 Foundation of the integration of TCM and nano-delivery systems
Nano-delivery systems and TCM exhibit a notable temporal disparity, as the former represent a relatively nascent discipline while the latter boasts a rich history spanning millennia. Intriguingly, despite their contrasting evolutionary trajectories, a remarkable affinity exists between these domains, as they share substantial congruence in their theoretical underpinnings and therapeutic objectives. Through their amalgamation and advancement, these fields mutually reinforce one another, thereby fostering enhanced efficacy in clinical interventions.19
Nanostructures are commonly found in the cells of Chinese herbal plants, as well as in biological secretion vesicles and herbal decoctions.20–23 These tiny structures play a crucial role in improving the solubility, stability, and bioavailability of active molecules. The inherent existence of these nanostructures reflects the composition of Chinese herbal medicine (CHM), establishing a strong basis for their incorporation. Nanomedicine is predominantly utilized for precise diagnostic procedures and treatments, aligning with the theory of meridian tropism in TCM.24,25 Both nanomedicine and TCM strive to minimize adverse effects and enhance treatment effectiveness. Nanomedicine employs contemporary methodologies to optimize drug formulations and carrier designs, whereas TCM achieves this through traditional practices such as herbal compatibility. This underscores the shared theoretical foundations and objectives of both approaches.
Nanotechnology plays a crucial role in enhancing the quality of pharmaceuticals, while traditional medical practices provide valuable experience and insights to address intricate challenges. The integration of targeted nanomedicine with TCM is gaining prominence due to the growing clinical utilization of nanomedicine and the advancements in integrated medicine. This emerging trend not only enhances the therapeutic possibilities, but also highlights the pragmatic necessity of amalgamating these two fields.
3.3 Nano-delivery systems improve the bioavailability
As nanotechnology progresses, the incorporation of drug loading into nano-sized particles within organic or inorganic materials to fabricate nano-carriers has emerged as a widely accepted and optimized controlled drug delivery technique.26 CHM ingredients are not easily dissolved in water, which leads to low absorption and quick metabolism when taken orally. Furthermore, they have drawbacks like limited targeting, widespread distribution, and rapid elimination. According to scholarly research, the reduction of TCM to the nanoscale has been found to yield various benefits, such as improved stability, an increased surface area for targeted tissue specificity, and enhanced pharmacological activity.27,28 Deng et al. conducted a study revealing that realgar particles below 150 nm demonstrated antitumor effects by inhibiting angiogenesis, while also exhibiting reduced toxicity.29 Through the utilization of nanoscale carriers, drugs can be encapsulated to shield them from degradation and subsequently released in a controlled manner at the desired site in vivo.
3.3.1 Increased solubility.
Nano-delivery systems have been found to provide numerous advantages, including an increased surface area and improved drug solubility.30 One example of this is the utilization of nanoscale particles to enhance the water solubility of honokiol, a compound known for its poor solubility.31 Moreover, previous studies have demonstrated the efficacy of curcumin liposomes and chitosan-encapsulated quercetin nanoparticles in improving bioavailability, thereby broadening their scope for various applications.32,33
3.3.2 Increased permeability.
Amidon et al. have introduced the Biopharmaceutics Classification System, which establishes the significance of drug solubility/dissolution properties in the gastrointestinal (GI) aqueous environment and the permeability of drugs across GI membranes as pivotal determinants influencing oral drug absorption.34,35 During the process of developing nano-delivery systems to enhance drug solubility, it is crucial to consider the membrane permeability of the drug within the human body.36 Augmented permeability facilitates the drug's ability to penetrate biological membranes and enter the systemic circulation upon administration, thereby enhancing absorption and improving bioavailability. A notable illustration of this phenomenon is the augmentation of permeability for drugs with low lipophilicity through the utilization of nano-delivery systems.37 Chen et al. employed amphiphilic poly(lactic acid-co-glycolic acid) (PLGA) copolymer nanoparticles within the small intestine to improve oral bioavailability. Subsequent in vitro permeation studies exhibited the copolymer nanoparticles’ exceptional absorptive characteristics.38 The transdermal delivery techniques further emphasize the enhanced permeation capabilities of nanomedicines. Isopsoralen, a challenging active component found in CHM for vitiligo treatment, faces obstacles due to its inadequate percutaneous absorption, limited epidermal retention, and restricted bioavailability. Pang et al. conducted a study wherein they created a nano-gel containing isopsoralen, which demonstrated the ability to penetrate the corneum and exhibited a threefold increase in epidermal retention compared with the original drug.39
3.3.3 Increased half-life.
It is commonly observed that nanoparticles are eliminated by the reticuloendothelial system (RES) and rapidly accumulate in the liver and spleen shortly after intravenous administration.40 Consequently, for drugs with a brief half-life, the development of a drug carrier capable of evading recognition by the mononuclear phagocyte system (MPS) or the RES represents a promising strategy. Research studies have indicated that the utilization of PEG-modified nanoparticles has been found to effectively improve RES absorption, thereby increasing their half-life in circulation.41 PEG, a biocompatible polymer, is well known for its low toxicity, high water solubility, and minimal immunogenicity, and has been approved by the United States Food and Drug Administration (FDA). In their study, Liu et al. developed a tamibarotene (Am80)-PEG-nanostructured lipid carrier (NLC) (Am80-PEG-NLC), which was further modified with PEG-40 stearate (PEG40-SA), an amphiphilic polymer derivative of hydrophilic PEG, to enhance drug solubility and prolong its duration in the bloodstream. According to reports, this derivative can be readily integrated into the lipid core of colloidal carriers with hydrophilic PEG chains on the surface.42 The findings indicate a decrease in renal accumulation and a notable prolongation of the mean residence time (MRT) for the Am80-PEG-NLC group.43
4. Integration of TCM and nano-delivery systems
4.1 Nano-delivery systems and CHM active compounds
The conventional administration approach for CHM primarily involves oral delivery, which is constrained by inadequate water solubility, low permeability, instability, extensive metabolism in intestinal and hepatic cells, and rapid elimination.44,45 In recent years, the utilization of nanotechnology in CHM has become increasingly prevalent. Consequently, the advent of nano-delivery systems based on nano-encapsulation and nano-adsorption techniques has proved effective in mitigating direct exposure to active constituents of CHM under physiological conditions. This approach not only increases stability and bioavailability, but also diminishes the occurrence of toxic adverse reactions, thereby significantly advancing the progress of CHM.
4.1.1 Nanoencapsulation systems.
Nanoencapsulation is the act of enclosing active compounds within nanometer-scale carriers, which can be composed of diverse materials, such as lipids and polymers. This process aims to improve the stability, solubility, and bioavailability of the encapsulated substance. By utilizing nanoencapsulated nanoparticles, drugs can be delivered specifically to cells or tissues in the body, thereby enhancing their therapeutic effects and reducing potential side effects.
4.1.1.1 Lipid-based nano-delivery systems in CHM compounds.
Lipid nanocarriers, in particular, have emerged as versatile drug delivery systems that have gained considerable recognition in the realm of targeted and controlled drug delivery. These nanocarriers encompass various systems, including liposomes, solid lipid nanoparticles (SLNs), nanostructured lipid carriers (NLCs), and emulsions.
Liposomes, as the first-generation nano-delivery system, are vesicular structures composed of lipid bilayers that enclose aqueous compartments. They offer advantages such as biocompatibility, biodegradability, and the ability to encapsulate both hydrophilic and hydrophobic agents, making them highly valuable in drug delivery.46 For example, Jhaveri et al. conducted a study wherein liposomes were formulated to encapsulate resveratrol and subsequently modified with transferrin (Tf-RES-L) to achieve specific targeting of glioblastomas, which upregulate transferrin receptors. Through a comprehensive series of in vitro and in vivo experiments, the researchers demonstrated that Tf-RES-L exhibits superior targeting capabilities and cytotoxicity against glioblastomas when compared to normal human astrocytes. Furthermore, in vivo investigations indicated that Tf-RES-L significantly prolongs the release of the drug in comparison with free resveratrol, potentially leading to enhanced accumulation of resveratrol at tumor sites and consequently augmenting its therapeutic efficacy (Fig. 3).47
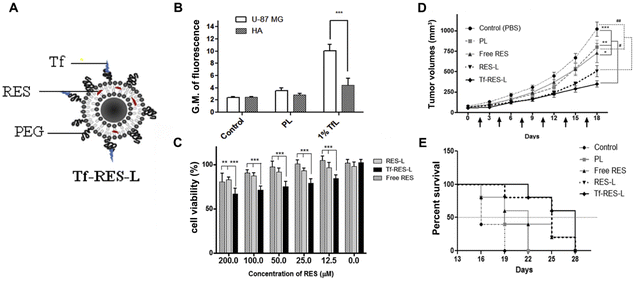 |
| Fig. 3 (A) Schematic illustration of Tf-RES-L. (B) Fluorescence intensity of glioblastomas (U87MG) and human astrocytes (HA) treated with rhodamine-labeled plain liposomes (PL) and liposomes with 1 mol% Tf on their surface. (C) Cytotoxicity of liposome formulations containing 12.5–200 μM RES. (D) Effects of Tf-RES-L on tumor growth inhibition in U87MG tumor xenograft-bearing mice. (E) Survival analysis of U87MG tumor xenograft-bearing mice treated with Tf-RES-L. Reproduced from ref. 46 with permission. Copyright 2018, Elsevier. | |
Solid lipid nanoparticles (SLNs), which represent the second generation of drug delivery systems, consist of a solid lipid core that is biocompatible and a surfactant shell that acts as a stabilizer.48 In comparison with liposomes, SLNs exhibit enhanced stability and a wider range of administration routes, thereby demonstrating favorable attributes such as diminished toxicity and heightened efficiency in oral absorption. In the case of SLNs, the solid core effectively encapsulates herbal constituents, such as frankincense and myrrh essential oils (FMO), preventing their oxidation or volatilization.49 Furthermore, the utilization of SLNs loaded with quercetin resulted in a significant improvement in its absorption.50 To mitigate the adverse effects on the male reproductive system in rats induced by tripterygium glycosides (TGs), researchers developed TGSLNs, which notably reduced the toxicity while maintaining their therapeutic efficacy.51 Xue et al. entrapped berberine within solid lipid nanoparticles (BBR-SLNs) to enhance the anti-diabetic effectiveness of berberine. The in vivo experiments conducted by the researchers demonstrated that the utilization of BBR-SLNs resulted in a higher concentration of berberine in plasma compared to the use of berberine alone. This finding suggests that BBR-SLNs significantly improved the absorption and bioavailability of berberine.52 In addition, it is worth noting that SLNs possess the potential for customization and functionalization through various modifications, enabling them to possess unique capabilities such as targeted delivery and sustained release. A notable example of this is the study conducted by Tan et al., where they synthesized 6-phosphate (M6P)-modified human serum albumin (HSA) and incorporated it into M6P-modified SLNs (MT-SLNs) for targeted drug delivery to fibrotic liver tissues. In their experiments, it was observed that M6P-guided SLNs demonstrated a predilection for accumulation in fibrotic livers and exhibited reduced metabolic rates, suggesting an augmentation in targeted drug delivery and improved bioavailability.53
Nanostructured lipid carriers (NLCs) represent the subsequent iteration of drug delivery systems, surpassing liposomes and presenting improved drug safeguarding and targeting functionalities. Diverging from solid lipid carriers (SLCs), NLCs employ a distinctive lipid structure that integrates both solid and liquid lipids, which enhances drug stability and significantly increases the drug-loading capacity.54 NLCs are composed of a blend of solid and liquid lipids, forming an amorphous solid matrix at both physiological temperature and room temperature.55 The utilization of NLCs has effectively mitigated the concerns surrounding drug expulsion during storage and the limited drug loading capacity observed in SLNs. NLCs offer enhanced control over drug release and improved stability when compared to SLNs.56–58 The emergence of NLCs has addressed the issue of drug expulsion during storage and the low capacity for loading drugs in SLNs.59,60 A notable example is the work of Truong et al., who prepared chitosan-coated nanostructured lipid carriers (CH-NLCs) for the delivery of tetrahydro curcumin, a prominent curcumin metabolite renowned for its anticancer attributes. Their results indicate that CH-NLCs possess notable characteristics such as high loading and encapsulation efficiency, sustained release profiles, and enhanced drug permeation. These results provide evidence supporting the viability of NLCs as an efficient hydrophobic drug delivery system (Fig. 4).61
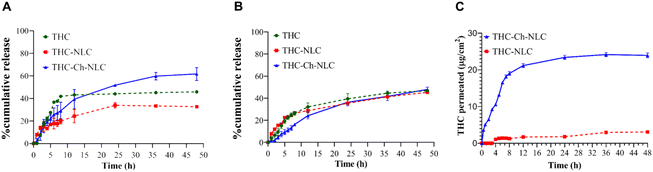 |
| Fig. 4 Release profiles and permeation study of unencapsulated THC and THC from THC-NLCs and THC-Ch-NLCs. (A) Cumulative release of THC under the simulated physiological conditions (pH 7.4). (B) Cumulative release of THC in the tumor microenvironment (pH 5.5). (C) In vitro permeation profiles of THC from THC-NLCs and THC-Ch-NLCs through a Strat-M® artificial skin membrane. Reproduced from ref. 60 with permission. Copyright 2022, Elsevier. | |
Various active components of CHM, including celastrol, gambogic acid, and triptolide, have been successfully co-loaded into NLCs to enhance therapeutic effects.62–64 It is worth noting that the drug-loading capacity of NLCs surpasses those of liposomes and solid lipid nanoparticles (SLNs), enabling the simultaneous delivery of multiple herbal components.65 In addition, NLCs have exhibited exceptional transdermal permeation capabilities for triptolide-loaded NLCs (TPL), surpassing the permeation rate of TPL nanoemulsion by nearly 11-fold.64
4.1.1.2 Polymer nanoparticles.
Polymeric micelles, characterized by their unique core–shell structure formed through the self-assembly of amphiphilic copolymers such as polyethylene glycol-poly(ε-caprolactone) (PEG-PCL), polyethylene glycol-poly(lactic-co-glycolic acid) (PEG-PLGA), and polyethylene glycol-poly(benzyl-L-glutamate) (PEG-PBLG), represent a highly sophisticated colloidal dispersion system. The hydrophobic core of polymeric micelles functions as a vehicle for lipophilic drugs, leading to a notable enhancement in drug solubility and protection against degradation.66 In contrast, the hydrophilic shell imparts a multitude of advantageous characteristics, including encompassing exceptional biocompatibility, stealth properties, passive targeting through the enhanced permeability and retention (EPR) effect, active targeting using various ligands, responsiveness to changes in temperature and redox-sensitivity, as well as pH-sensitivity.67–71
The current research efforts in the field of polymeric micelles are primarily directed toward attaining accurate cellular targeting and enhancing the accumulation of drugs within cells. An example of this is artemisinin, which is widely recognized for its potent antitumor properties by impeding cell proliferation and including apoptosis. In addition, artemisinin has been found to inhibit lymphangiogenesis in murine models. Wang et al. ingeniously devised a clever approach in their study by developing PEG-PCL micelles loaded with artemisinin. To enhance the targeting efficiency, the micellar shell was modified with LyP-1, a cyclic nonapeptide known for its affinity towards the highly expressed p32/gC1qR receptor found in tumor cells and lymphatic vessels. This modification effectively facilitated endocytosis, resulting in improved drug uptake by both tumor and lymphatic endothelial cells. As a result, the drug accumulated to a greater extent at tumor sites and within tumor lymphatic vessels. The effectiveness of LyP-1-modified polymeric micelles in delivering artemisinin to highly metastatic breast tumors and tumor-associated lymphatic vessels has been unequivocally validated through rigorous in vitro and in vivo experiments (Fig. 5).72
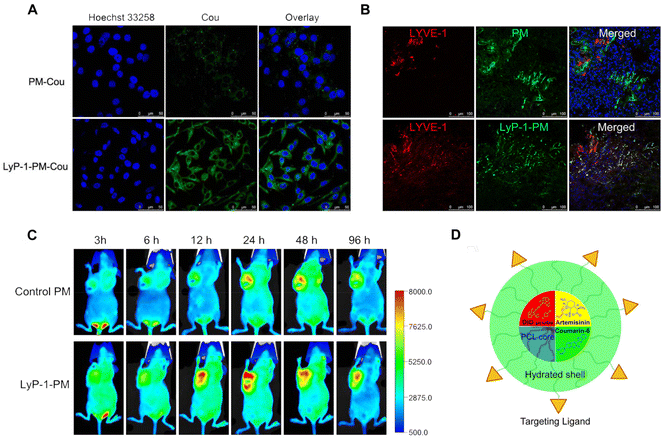 |
| Fig. 5 LyP-1-conjugated PEG-PCL micelles (LyP-1-PM) target metastatic tumors and their lymphatic vessels. (A) Cellular uptake of polymeric micelles by highly metastatic breast cancer cells. (B) Colocalization of LyP-1-PM and PM in breast cancer tissue with the lymphatic endothelial marker (LYVE-1). (C) In vivo near-infrared fluorescence imaging of tumor-bearing nude mice treated with DiD-labled micelles. (D) Schematic illustration of LyP-1-PM. Reproduced from ref. 71 with permission. Copyright 2012, American Chemical Society. | |
The utilization of various polymer types, such as poly(lactic acid) (PLA), poly(glycolic acid) (PGA), and their copolymer, poly(lactide-co-glycolide) (PLGA), has been increasingly observed in TCM research due to the biocompatibility, biodegradability, and versatile degradation kinetics of these polymers. In a study conducted by Snima et al., PLGA nanoparticles were skillfully engineered to encapsulate silymarin, demonstrating selective toxicity to prostate cancer cells and sustained drug release.73 Furthermore, Xu et al. skillfully crafted RGD (Arg-Gly-Asp peptide)-modified PLA nanoparticles for targeted delivery of oridonin, resulting in an enhanced tumor-targeting efficiency and subsequent antitumor efficacy in vivo.74 In conclusion, the proficient manipulation of polymers, particularly PLA, PGA, and PLGA, highlights their potential as powerful tools in TCM research, offering promising opportunities for advanced therapeutic interventions.
4.1.2 Nanoadsorption systems.
Nanoadsorption is a technique that utilizes nanomaterials, such as nanoparticles and nanofibers, to adsorb active compounds. Mesoporous nanomaterials are a type of nanostructured material characterized by pores ranging in size from 2 to 50 nanometers.75 These materials possess distinct advantages compared to conventional polymers or lipid nanocarriers, such as a significantly high specific surface area, remarkable material stability, low biotoxicity, and exceptional drug-loading capabilities.76,77 By capitalizing on these advantages, they are ingeniously designed as carriers for drug delivery, primarily aiming to address the issue of low bioavailability commonly observed in specific active components found in CHM. For instance, Zhang et al. demonstrated their proficiency by employing mesoporous silica nanoparticles (MSN) as a carrier, expertly adsorbing curcumin within the mesopores. The authors successfully achieved a high drug-loading capacity of curcumin by covalently attaching fucoidan to the external surface of mesoporous silica nanoparticles (MSN) through disulfide bonds.78 This approach also ensured responsive drug release, triggered by both glutathione and pH conditions. In a separate study, He et al. effectively developed a drug delivery system using MSN, where paclitaxel was loaded by the solvent evaporation method. Their analytical data indicated that the drug loading concentration of the MSN reached equilibrium, approximately 21%, within a 24-hour timeframe. Remarkably, cytotoxicity assays revealed that the MSN exhibited negligible toxicity to cells, even at a high concentration of 100 μg mL−1. Conversely, the paclitaxel-loaded MSN (MSN@PTX) exhibited significant cytotoxicity against Hep G2 cells, notably surpassing the cytotoxicity of free paclitaxel. These results collectively emphasize the potential of the MSN approach for the adsorption and delivery of paclitaxel.79
Gold nanoparticles (AuNPs) have attracted considerable attention in the realm of biomedical applications due to their unique optical, electronic, and chemical properties. These properties, such as the ability to easily attach therapeutic active ingredients from CHM, agents, antibodies, peptides, and nucleic acids, to their functionalized surfaces, offer great potential for enhancing targeted drug delivery. In addition, the inherent surface plasmon resonance (SPR) of AuNPs plays a dual role by enabling diagnostics and bio-labeling, as well as facilitating photothermal therapies. Under specific light irradiation, targeted tumor cells can be effectively eliminated via heat generated from the nanoparticles.80–82 In a study conducted by Manju et al., multifunctional gold nanoparticles (AuNPs) were reported. These AuNPs were skillfully stabilized through the covalent conjugation of water-soluble curcumin onto AuNPs, followed by functionalization using folic acid-conjugated polyethylene glycol (PEG-FA). This approach significantly enhanced both tumor targeting and the permeability and retention effect of the drug.83 AuNPs exhibit a noteworthy combination of low cytotoxicity and efficient drug-loading capabilities, making AuNPs highly promising candidates for advanced drug delivery systems.84 Zhang et al. pioneered an innovative method by covalently attaching paclitaxel molecules to gold nanoparticles using fluorescent antisense oligonucleotide linkers. This strategy significantly improved the solubility of paclitaxel, as the dense coating of synthetic oligonucleotides on the nanoparticles promoted better dispersion and consequently led to a significant increase in solubility. The increased specific surface area of gold nanoparticles significantly augmented their capacity for drug loading, thereby elevating the solubility of therapeutic agents.85 It is important to note that while gold nanoparticles show immense potential in drug delivery applications, extensive research into their long-term safety, biodistribution, and intricate interactions with various molecules and cells within the biological system is imperative before considering their widespread implementation in clinical settings.
Nanogels and microgels are representative examples of polymer crosslinked networks at the nanoscale. They can be synthesized through various polymerization reactions, such as free radical or addition reactions, and different processes, including solution polymerization, mini-emulsion, or precipitation polymerization.86–90 In addition, biopolymers, such as polyacrylic acid (PAA), can be utilized as a structural framework for nanogel construction. PAA demonstrates exceptional biocompatibility and can enhance the disruption of tight junctions between epithelial cells, promoting drug permeation through mucus.91 Furthermore, hydrophobic drugs and macromolecules can be conjugated to the polymer backbone via ester or amide groups, resulting in nanogels with multiple guest molecules. Qian and colleagues successfully fabricated stable PAA-β-cyclodextrin (PAA-βCD)/PAA-paclitaxel (PAA-TAX) nanogels, which were specifically designed for intravaginal drug delivery. The purpose of these nanogels was to prevent drug leakage, prolong residence time within the vaginal cavity (Fig. 6A), and ensure controlled release at targeted sites. The experimental findings suggest that these mucosal-adhesive nanogels exhibit exceptional stability and show potential as carriers for the treatment of cervical cancer (Fig. 6B and C).92
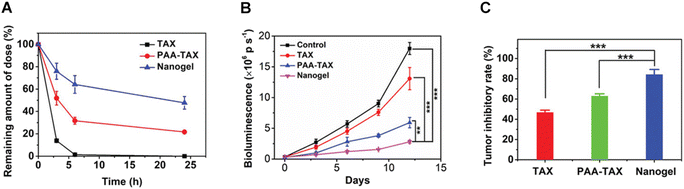 |
| Fig. 6 (A) Amount of TAX retained in the mouse cervicovaginal tract over time after vaginal administration with free TAX, PAA-TAX, and a nanogel. (B) Changes in the bioluminescence signal after treatment with different formulations over time. (C) Tumor inhibitory rate of different groups treated with various formulations. Reproduced from ref. 91 with permission. Copyright 2019, John Wiley and Sons. | |
4.2 Nanostructures in extracted CHM
Herbal decoctions, which are widely employed in TCM to address diverse ailments, undergo a meticulous preparation process that involves the combination of multiple Chinese medicinal ingredients under TCM compounding theory. This intricate process ultimately yields a soluble and complex multicomponent dispersed system, comprising solutes, aggregates, and precipitates. This system exhibits a close association with the transportation, effects, and metabolism of bioactive substances.93,94
Significant progress has been achieved by researchers in the realm of CHM through the identification of bioactive nanoscale aggregates within the dispersion systems of herbal decoctions. One study uncovered the presence of these nanoscale aggregates in 84 different herbal decoctions.95 Subsequently, another study successfully isolated nanoscale aggregates possessing antipyretic effects from a traditional water-extracted preparation called Bai Hu Tang utilizing high-speed centrifugation. Notably, these nanoscale aggregates have demonstrated enhanced antipyretic effects in comparison with other dispersed phases in a rabbit fever model induced by lipopolysaccharides. Further analysis using transmission electron microscopy (TEM) and high-performance liquid chromatography (HPLC) elucidated that the majority of these nanoscale aggregates exhibited a diameter measuring 100 nm. These aggregates were loaded with antipyretic bioactive compounds such as neomangiferin, mangiferin, glycyrrhizic acid, and glycyrrhizic acid ammonium, which possessed the ability to be swiftly assimilated by cells and exert targeted effects on the brain and lungs.23
In another study, researchers successfully isolated spherical colloidal nanoparticles from a conventional medicinal formula known as Ma Xing Shi Gan Tang using liquid chromatography techniques. Reverse-phase chromatography analysis indicated that most pseudoephedrine and ephedrine were associated with isolated spherical nanoparticles rather than being freely dispersed in the solution. Subsequent cellular experiments confirmed these findings.96 Hu et al. made a significant breakthrough in understanding the presence of bioactive molecule aggregates in herbal decoctions. They found that these aggregates encompassed various forms, including the aggregation of individual molecules, the aggregation between different molecules, and the aggregation between different molecules and primary metabolites. In addition, their research revealed the presence of bioactive constituents, namely puerarin, genistein, genistin, and isorhamnetin, in the aggregated forms.97
Another study conducted by Huang et al. focused on the physical mixture of herbs and plant chemical substances. Their observations indicated that this amalgamation displayed a nanofiber morphology, whereas the co-decoction of these constituents resulted in nanospheres. Importantly, the change in the self-assembled morphology driven by thermal energy led to an enhancement in the antimicrobial efficacy. This finding provides empirical support for the potential of co-decoction of CHM to augment bioactivity.98 Furthermore, this finding sheds light on the decoction procedure employed in the preparation of herbal remedies. Within this process, the presence of amphiphilic active monomers, influenced by various forces such as hydrophobic interactions, hydrogen bonds, electrostatic interactions, and van der Waals forces, can induce the formation of nanoparticles. These phenomena have significant implications for the bioavailability and bioactivity of the individual components of CHM.99 These studies provide insight into the complex nature of bioactive molecule aggregates found in herbal decoctions, emphasizing their influence on the bioactivity and bioavailability of CHM components.
4.3 Acupuncture and nanodrug delivery systems
Acupuncture, one of the major therapeutic methods of TCM and boasting a historical lineage of over three millennia, has amassed considerable clinical and theoretical evidence, solidifying its prominent position as a globally prevalent alternative and complementary medical practice. Guided by the principles of TCM, acupuncture serves as a means of modulating the body's physiological functions through the targeted stimulation of acupoints located on the body's surface. This therapeutic technique is widely employed to alleviate or treat a variety of diseases, including endocrine and metabolic disorders, mental and behavioral disorders, neurological diseases, circulatory system disorders, skin diseases, musculoskeletal and connective tissue disorders, and various others.100
4.3.1 Long-time acupuncture and nano-delivery systems.
Acupuncture point injection, also known as acupoint injection, is a modified technique within the field of acupuncture that emerged in China during the 1950s. It originated from the practice of intramuscular injections in Western medicine and has gradually become integrated into TCM through modernization.101 Commonly employed substances for acupoint injections encompass herbal extracts, Western medications, vitamins, bee venom, and saline solution. The administration of injections at acupoints allows for a synergistic interaction between the medication and the meridians and acupoints, which is believed to yield more enduring effects in comparison with conventional acupuncture needling or intramuscular injections.102–104 For instance, Ji et al. conducted research using PLGA gel injected into the Neiguan acupoint (PC6) in rats with myocardial ischemia. Their findings revealed that the injection of the gel at this acupoint significantly reduced the size of the myocardial infarction area, restored pathological changes, alleviated oxidative stress injuries, reduced inflammatory responses, and suppressed myocardial cell apoptosis.105
The incorporation of nanotechnology into TCM has led to increased adoption of nano-delivery systems in acupuncture. These systems involve the loading of bioactive substances onto nano-carriers, which are then administered through acupoint injections. This approach allows for slow release and prolonged stimulation or it involves the modification of acupuncture needles to carry biologically active molecules, thereby regulating the body's physiological functions. This intersection of nanotechnology and acupuncture presents exciting opportunities for enhancing therapeutic outcomes and delving into the intricate mechanisms that underlie the effects of acupuncture.
In the field of pain management, the adenosine A1 receptor (A1R) is recognized as a significant factor in achieving localized analgesia.106 The administration of the selective A1 receptor agonist, 2-chloro-N(6)-cyclopentyladenosine (CCPA), exhibits analgesic properties akin to acupuncture when injected at the Zusanli acupoint (ST36).107 In addition, triptolide (TP), a bioactive compound from TCM derived from the Chinese herb, Tripterygium wilfordii Hook F, is well known for its potent anti-inflammatory and immunosuppressive effects.108 Ren et al. employed HSA, recognized for its arthritic targeting capabilities, as a nano-carrier for encapsulating and delivering TP (TP@HSA NPs).109–112 Furthermore, they developed a nano-composite hydrogel (TP@HSA NP-CCPAGel) by incorporating CCPA into the hydrogel reservoir, allowing for targeted delivery of TP. This hydrogel was administered locally at the Zusanli point (ST36) in rats with rheumatoid arthritis (RA), and the sustained release of CCPA mimicked the pain relief effects observed in the acupuncture therapy (Fig. 7A).112 The results demonstrated that the TP@HSA NP–CCPAGel nanocomposite hydrogel synergistically improves inflammation, prevents bone erosion, and mitigates systemic toxicity, thereby exerting a comprehensive therapeutic effect.
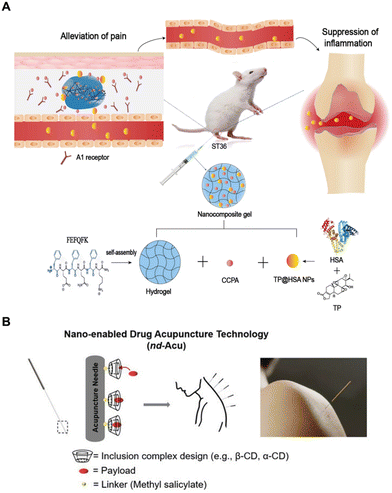 |
| Fig. 7 (A) Therapeutic mechanism of the nano-composite hydrogel (TP@HSA NPs-CCPAGel) against RA. Reproduced from ref. 111 with permission. Copyright 2021, Springer Nature. (B) Schematic of nd–Acu design. Reproduced from ref. 112 with permission. Copyright 2023, Wiley-VCH GmbH. | |
According to the theory of meridians and acupoints, acupuncture achieves therapeutic effects by inserting acupuncture needles into the corresponding acupoints.113,114 Utilizing nano-delivery systems to incorporate biologically active ingredients onto the surface of stainless steel needles allows for the simultaneous release of these ingredients during the mechanical stimulation of acupuncture, thereby enhancing therapeutic efficacy. Xu and colleagues have made significant strides in the traditional acupuncture field by introducing a nano-enabled drug delivery acupuncture technology (nd-Acu) platform. Notably, they achieved this by utilizing an electrochemical procedure to link cyclodextrin modified with methyl salicylate ester onto the surface of acupuncture needles. The hydrophobic interior cavity of cyclodextrin allows for the encapsulation of single or multiple payload molecules as inclusion complexes. Methyl salicylate ester (MeSA) acts as a linker, securing the cyclodextrin loaded with active molecules onto the surface of acupuncture needles. In vivo and in vitro experiments demonstrate excellent drug-loading capacity and time-dependent release, resulting in significantly enhanced overall efficacy compared to traditional acupuncture needles (Fig. 7B).115
4.3.2 Acupuncture needle with nano-sensors and nano-delivery systems.
Nano-delivery systems can integrate nano-sensors with acupuncture needles, enabling real-time monitoring of changes in the levels of active molecules in the body, which contributes to a deeper understanding of the molecular mechanisms of acupuncture therapy. Serotonin (5-HT) is a crucial substance released in local reactions of mast cells, playing a key role in pain relief. Acupuncture demonstrates significant efficacy in analgesic treatment, and local acupuncture at specific acupoints can have systemic therapeutic effects.116 Research indicates that the concentration of mast cells in the acupuncture points of both humans and animals is significantly higher than that in sham points. When acupuncture needles penetrate acupoints, there is a significant increase in mast cell degranulation, leading to a substantial elevation in the local 5-HT concentration. Carbon nanotubes (CNTs) are widely used in the sensing field and can measure pH, temperature, and biomedical information. Poly(3,4-ethylenedioxythiophene) (PEDOT) is employed for its excellent conductivity and stability in electrochemical applications. Li and colleagues utilized PEDOT to stably attach carbon nanotubes to the surface of acupuncture needles, developing a novel CNT/AN nanosensor needle. This needle exhibits strong stability and sensitivity, enabling efficient loading of active biomolecules, including 5HT, and real-time monitoring of serotonin levels in the serum of rats during the acupuncture process at the Zusanli acupoint (ST 36) (Fig. 8A).117 Nitric oxide (NO) is a crucial regulator of local circulation.118–120 Li and collaborators found a significant increase in NO levels in the blood after warm needle acupuncture.121 Tsuchiya et al. discovered that acupuncture stimulation at specific acupoints enhanced the production of NO in acupoints and increased local circulation.122 For real-time monitoring of changes in NO in local circulation, Tang et al. utilized the superior electrical properties of graphene and the catalytic properties of porphyrin to develop a functionalized acupuncture needle with high sensitivity and selectivity. This needle is capable of real-time monitoring of NO levels at different acupoints, such as Zusanli (ST36), Zhongwan (CV12), and Quchi (LI11), enabling in vivo detection of signaling molecules (Fig. 8B).123
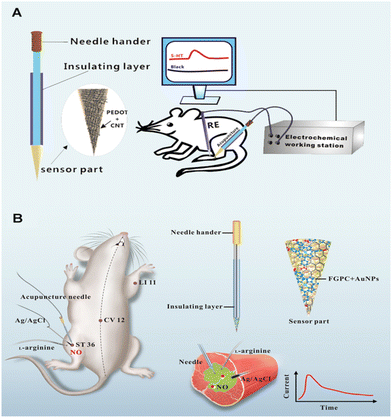 |
| Fig. 8 (A) Schematic diagram of real time and in vivo monitoring of 5-HT by means of the PEDOT/CNT-modified acupuncture needle. Reproduced from ref. 117 with permission. Copyright 2016, Springer Nature. (B) Schematic illustration of a sensitive acupuncture needle microsensor for real-time monitoring of NO in the acupoints of rats. Reproduced from ref. 123 with permission. Copyright 2017, Springer Nature. | |
These groundbreaking applications harness the potential of nano-delivery systems, effectively bridging the gap between acupuncture and pharmaceutical interventions, while enabling the exploration of the molecular mechanisms of acupuncture. This opens up new perspectives for the modernization of traditional Chinese medicine. In addition to revitalizing acupuncture therapy, these advancements pave the way for exploring novel pathways in the development of traditional Chinese medicine. They not only expand the scope of traditional Chinese medicine treatments, but also offer patients more effective and personalized therapeutic options.
4.4 Molecular compatibility theory and nano-delivery systems
Component compatibility, as proposed by academicians Wang Yongyan and Zhang Boli, is rooted in the fundamental principles of TCM.124 It encompasses a multi-step approach that begins with the isolation of individual standard components, followed by the establishment of group–effect relationships to identify the most effective components.
Molecular compatibility combines high efficiency and low toxicity advantages to overcome tumor treatment resistance. It proposes theories and methods for treating cancer by combining traditional Chinese and Western medicine, aiming to enhance the effectiveness of anti-tumor drugs. It follows the principles of traditional Chinese medicine in selecting drugs dialectically and leveraging its advantages in overcoming tumor resistance. It integrates traditional Chinese medicine with modern medicine through the “molecular compatibility” theory, addressing the issue of unclear material. It combines traditional Chinese medicine's drug selection based on dialectics and overcoming tumor resistance with the theory of differentiation and treatment and the principle of compatibility with “monarch, minister, assistant and guide”. In addition, it incorporates the “molecular compatibility” theory, which combines traditional Chinese and Western medicine to overcome the limitations of both approaches. It integrates effective molecular components with well-documented structures, efficacies, and specific functional targets into compound formulas. These formulations manifest as modern preparations that adhere to rigorous quality standards, reflecting an ongoing progression and refinement of component compatibility, indicative of a more advanced stage in its development.
The development of new drugs, guided by the principles of molecular compatibility theory, is characterized by distinct chemical structures, precise molecular formulas, and exact component weights in the formulation. For instance, the composition of elemene liposomes consists of β-elemene, γ-elemene, δ-elemene, soybean phospholipids, cholesterol, disodium hydrogen phosphate, and sodium dihydrogen phosphate. These components are skillfully synthesized into liposomes utilizing nanotechnology.125 Based on the theory of Jun-Chen-Zuo-Shi (monarch, minister, assistant, and envoy) compatibility in TCM, the optimization of molecular compatibility was optimized among the seven components. Among them, β-elemene, known for its anticancer effects, was identified as the principal ingredient (monarch) (Fig. 9), while γ-elemene and δ-elemene acted as auxiliary drugs (minister drugs), playing a synergistic role to enhance the anticancer activity of β-elemene. Cholesterol and soybean phospholipids acted as assistant ingredients, contributing to the formation of a bilayer membrane structure within the liposomes. This nanocapsulation improved the elemene stability, water solubility, and targeted delivery, mitigating the risk of potential toxic side effects. The inclusion of disodium hydrogen phosphate and sodium dihydrogen phosphate as envoy ingredients played a crucial role in regulating pH and water solubility, while also facilitating the formation of a nano-carrier using soybean phospholipids for the effective delivery of elemene. Xie et al. employed liposome targeting technology to encapsulate isolated elemene within a phospholipid bilayer, which has subsequently evolved into China's first industrial production line of liposomes to the rigorous standards of Good Manufacturing Practice (GMP) guidelines. The elemene liposome injections and oral emulsions that were developed and introduced in 1994 have served as a paradigm for the application of nano-delivery systems in the field of TCM. Extensive clinical investigations conducted over the last twenty years have consistently shown that oleanolic acid liposomes possess the ability to effectively impede various cancer cells by targeting multiple pathways, while also enhancing immune function. Notably, these liposomes offer substantial benefits in terms of enhancing patients’ overall well-being, extending their lifespan, preventing the spread and reappearance of cancer, and reversing resistance to multiple drugs.126
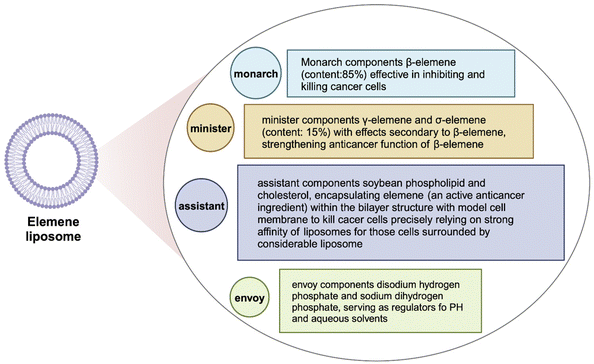 |
| Fig. 9 Molecular compatibility theory in elemene liposomes. Adapted from ref. 125 with permission. Copyright 2023, Elsevier. | |
The theory of molecular compatibility holds great importance in the realm of modern medicine, encompassing the compatibility and application of modern medicines, as well as the research and development of new drugs. The escalation of dosages in Western medicines to augment their effectiveness frequently results in adverse reactions and the emergence of drug resistance, particularly evident in the field of anti-tumor treatment. Molecular compatibility provides an effective approach to tackle this predicament effectively. For example, Li et al. successfully developed actively targeted biomimetic liposomes, Tf-ELE/CTX@BLIP, by coupling elemene (ELE) and cabazitaxel (CTX) liposomes with transferrin (Tf), as well as the encapsulation of cell membrane proteins from RG2 glioma cells within the liposomes. The results demonstrated that Tf-ELE/CTX@BLIP exhibited high stability, low toxicity, and effective blood–brain barrier (BBB) infiltration, and demonstrated a reduction in the tumor volume, consequently leading to prolonged survival in a murine model.127
Currently, there has been a growing proliferation of comparable investigations, including the utilization of elemene in combination with gefitinib for the compatibility treatment of patients with drug-resistant lung adenocarcinoma128 and the use of elemene in combination with docetaxel for the compatibility treatment of patients with drug-resistant pancreatic cancer,129 among other examples. These studies highlight the potential of molecular compatibility theories and the development of novel drug combinations as effective strategies in addressing drug resistance and improving therapeutic outcomes in various diseases.
5. Conclusions and perspectives
The emergence of nano-delivery systems serves as a tangible manifestation of the convergence of modern science and technology and traditional medicine, infusing the field of TCM with renewed vigor and prospects. Bibliometric studies indicate a consistent rise in global interest regarding nano-delivery systems in the realm of TCM. Researchers from various countries such as China, the United States, and India, play pivotal roles in this field, highlighting its global importance. The application of nano-delivery systems offers multiple advantages, providing robust support for both research and practice in TCM. Primarily, it significantly enhances the bioavailability and efficacy of active constituents found in traditional Chinese herbs. Through the encapsulation of these active components within nanocarriers, drugs can be delivered more stably and precisely to specific tissues or cells, thereby improving therapeutic results. For instance, the incorporation of active compounds such as baicalin or Panax notoginseng saponins into nanoparticles has been found to significantly improve their bioavailability and mitigate adverse effects, presenting a promising strategy for optimizing the therapeutic effectiveness of TCM. Secondly, nano-delivery systems provide innovative opportunities for formulating intricate combinations of TCM ingredients and serve as a technological foundation for the development of new herbal medicines. This aids a more comprehensive comprehension of the molecular compatibility theory in TCM, promoting the integration of traditional theories with modern science to enhance predictability and personalized treatment possibilities. Furthermore, nano-delivery systems offer modern technological support for traditional Chinese therapeutic methods such as acupuncture. By combining drug carriers with acupuncture, more precise treatment targets can be achieved, thereby enhancing efficacy and diversifying treatment options, while cupping, moxibustion, and massage, due to their unique operational techniques and the training methods of Qigong (movement and concentration exercises), may not easily benefit from the advancements in nanotechnology.130 However, recent research holds the promise of providing new insights. Beige adipose tissue plays a pivotal role in maintaining systemic energy balance. Polyethylene glycol (PEG)-crosslinked polydopamine nanoparticles (PDA) represent a biologically safe injectable photothermal hydrogel capable of converting near-infrared (NIR) light input into a precisely controlled temperature output. In a study by Li et al., localized heating therapy (LHT) employing PEG-crosslinked PDA post-injection effectively stimulated beige adipose tissue activation in mice. This approach demonstrated promising results in preventing and treating obesity in mice, without inducing adverse reactions. Moxibustion, a traditional Chinese medicine practice exemplifying local hyperthermia therapy, holds potential when combined with such innovative methods, expanding the therapeutic applications of moxibustion.131 Drawing inspiration from cupping therapy, Lallow and colleagues devised a groundbreaking vaccination technique to transfect DNA molecules into skin cells. They introduced purified DNA and applying negative pressure at the injection site, which induces tension and relaxation in the skin, facilitating the absorption of DNA molecules by skin cells. This method was employed in animal experiments to deliver a DNA vaccine against COVID-19, resulting in a robust immune response approximately 100 times more potent than administering the DNA vaccine alone.132 With the continuous development of nanotechnology, the integration of traditional Chinese medicine techniques such as acupuncture, cupping, moxibustion, and massage with nanotechnology has infused new vitality, providing broader application scenarios and accelerating the modernization of traditional Chinese medicine.
Despite significant progress in the research of nano-delivery systems within TCM, several challenges persist. First, the preparation techniques need ongoing improvement to enhance the stability, drug-loading capacity, and production efficiency of nano-delivery systems. Second, the prioritization of drug safety and toxicity assessment, especially in extensive and prolonged utilization, is of utmost importance. In addition, there is a need for additional refinement in the optimization of storage conditions and transportation issues for nano-delivery systems to guarantee the enduring efficacy and safety of drugs. Nevertheless, in terms of prospects, a diverse array of applications for nano-delivery systems within the field of TCM is anticipated. First, increased integration of TCM therapies with nano-delivery systems is expected to augment treatment effectiveness. For example, further research into the combination of drug carriers with acupuncture techniques has the potential to yield novel treatment modalities to replace traditional acupuncture, providing a better treatment experience for patients. Moreover, the utilization of nano-delivery systems holds significant promise in facilitating the modernization and internationalization of TCM, thereby bolstering its global recognition and application. In addition, it is imperative to strengthen safety assessment and regulatory measures of nano-delivery systems to ensure their clinical applicability, controllability, and sustainability.
Author contributions
Prof. Kong, Prof. Xie, and Prof. Wu conceived the overall framework of this review. J. Zou, M. Li, and Z. Liu wrote the original draft. W. Luo, S. Han, and F. Xiao and Prof. Tao reviewed and edited the draft.
Conflicts of interest
There are no conflicts to declare.
Acknowledgements
This study was supported by the National Natural Science Foundation of China (Grant No. 81730108 and 81973635 to T. X. and 82122076 to N. K.), the Science and Technology Development Fund, Macau SAR (No.: 0098/2021/A2 and 0048/2023/AFJ), and the China Postdoctoral Science Foundation (No.: 2023M733022).
References
- Y.-Y. Lu, Q.-L. Chen, Y. Guan, Z.-Z. Guo, H. Zhang, W. Zhang, Y.-Y. Hu and S.-B. Su, BMC Complementary Altern. Med., 2014, 14, 371 CrossRef PubMed.
- Y. Xu, K. Feng, H. Zhao, L. Di, L. Wang and R. Wang, Theranostics, 2022, 12, 1683–1714 CrossRef CAS PubMed.
- M. Huang, R. Li, M. Yang, A. Zhou, H. Wu, Z. Li and H. Wu, Front. Pharmacol., 2022, 13, 989139 CrossRef CAS PubMed.
- K. M. Nelson, N. D. Irvin-Choy, M. K. Hoffman, J. P. Gleghorn and E. S. Day, Adv. Drug Delivery Rev., 2021, 170, 425–438 CrossRef CAS PubMed.
- X.-Q. Zhang, X. Xu, N. Bertrand, E. Pridgen, A. Swami and O. C. Farokhzad, Adv. Drug Delivery Rev., 2012, 64, 1363–1384 CrossRef CAS PubMed.
- J. H. Park, D. Hong, J. Lee and I. S. Choi, Acc. Chem. Res., 2016, 49, 792–800 CrossRef CAS PubMed.
- K. Y. Vlasova, A. Piroyan, I. M. Le-Deygen, H. M. Vishwasrao, J. D. Ramsey, N. L. Klyachko, Y. I. Golovin, P. G. Rudakovskaya, I. I. Kireev, A. V. Kabanov and M. Sokolsky-Papkov, J. Colloid Interface Sci., 2019, 552, 689–700 CrossRef CAS PubMed.
- J. S. Brandt, O. Hadaya, M. Schuster, T. Rosen, M. V. Sauer and C. V. Ananth, JAMA Netw. Open, 2019, 2, e1918007 CrossRef PubMed.
- M. Bar-Zeev, Y. D. Livney and Y. G. Assaraf, Drug Resist. Updates, 2017, 31, 15–30 CrossRef PubMed.
- A. Florczak, T. Deptuch, A. Lewandowska, K. Penderecka, E. Kramer, A. Marszalek, A. Mackiewicz and H. Dams-Kozlowska, J. Nanobiotechnol., 2020, 18, 177 CrossRef CAS PubMed.
- M. J. Hajipour, H. Aghaverdi, V. Serpooshan, H. Vali, S. Sheibani and M. Mahmoudi, Nat. Commun., 2021, 12, 2984 CrossRef CAS PubMed.
- M. B. Yatvin, W. Kreutz, B. A. Horwitz and M. Shinitzky, Science, 1980, 210, 1253–1255 CrossRef CAS PubMed.
- R. Langer and J. Folkman, Nature, 1976, 263, 797–800 CrossRef CAS PubMed.
- T. M. Allen and A. Chonn, FEBS Lett., 1987, 223, 42–46 CrossRef CAS PubMed.
- R. Gref, Y. Minamitake, M. T. Peracchia, V. Trubetskoy, V. Torchilin and R. Langer, Science, 1994, 263, 1600–1603 CrossRef CAS PubMed.
- A. L. Klibanov, K. Maruyama, V. P. Torchilin and L. Huang, FEBS Lett., 1990, 268, 235–237 CrossRef CAS PubMed.
- L. Miao, Y. Zhang and L. Huang, Mol. Cancer, 2021, 20, 41 CrossRef CAS PubMed.
- S. Duggan, Drugs, 2018, 78, 1639–1642 CrossRef CAS PubMed.
- J. Zhang, K. Hu, L. Di, P. Wang, Z. Liu, J. Zhang, P. Yue, W. Song, J. Zhang, T. Chen, Z. Wang, Y. Zhang, X. Wang, C. Zhan, Y.-C. Cheng, X. Li, Q. Li, J.-Y. Fan, Y. Shen, J.-Y. Han and H. Qiao, Adv. Drug Delivery Rev., 2021, 178, 113964 CrossRef CAS PubMed.
- Q. Cai, L. Qiao, M. Wang, B. He, F.-M. Lin, J. Palmquist, S.-D. Huang and H. Jin, Science, 2018, 360, 1126–1129 CrossRef CAS PubMed.
- G. van Niel, G. D'Angelo and G. Raposo, Nat. Rev. Mol. Cell Biol., 2018, 19, 213–228 CrossRef CAS PubMed.
- X. Li, Z. Liang, J. Du, Z. Wang, S. Mei, Z. Li, Y. Zhao, D. Zhao, Y. Ma, J. Ye, J. Xu, Y. Zhao, J. Chang, Y. Qin, L. Yu, C. Wang and C. Jiang, Sci. China: Life Sci., 2019, 62, 333–348 CrossRef CAS PubMed.
- S. Lü, H. Su, S. Sun, Y. Guo, T. Liu, Y. Ping and Y. Li, Sci. Rep., 2018, 8, 12209 CrossRef PubMed.
- Y.-X. Chang, Y.-G. Sun, J. Li, Q.-H. Zhang, X.-R. Guo, B.-L. Zhang, H. Jin and X.-M. Gao, J. Chromatogr. B: Anal. Technol. Biomed. Life Sci., 2012, 911, 71–75 CrossRef CAS PubMed.
- P. Liu, S. Liu, G. Chen and P. Wang, Front. Med., 2013, 7, 277–279 CrossRef PubMed.
- C. X. Song, V. Labhasetwar, H. Murphy, X. Qu, W. R. Humphrey, R. J. Shebuski and R. J. Levy, J. Controlled Release, 1997, 43, 197–212 CrossRef.
- D. F. Nixon, C. Hioe, P. D. Chen, Z. Bian, P. Kuebler, M. L. Li, H. Qiu, X. M. Li, M. Singh, J. Richardson, P. McGee, T. Zamb, W. Koff, C. Y. Wang and D. O'Hagan, Vaccine, 1996, 14, 1523–1530 CrossRef CAS PubMed.
- J. Molpeceres, M. Guzman, M. R. Aberturas, M. Chacon and L. Berges, J. Pharm. Sci., 1996, 85, 206–213 CrossRef CAS PubMed.
- Y. Deng, H. Xu, K. Huang, X. Yang, C. Xie and J. Wu, Pharmacol. Res., 2001, 44, 513–518 CrossRef CAS PubMed.
- G. Yan, Y. Wang, X. Han, Q. Zhang, H. Xie, J. Chen, D. Ji, C. Mao and T. Lu, Dose-Response, 2019, 17, 1559325819872854 CrossRef PubMed.
- W. Wu, L. Wang, L. Wang, Y. Zu, S. Wang, P. Liu and X. Zhao, Int. J. Nanomed., 2018, 13, 5469–5483 CrossRef CAS PubMed.
- N. Hamano, R. Böttger, S. E. Lee, Y. Yang, J. A. Kulkarni, S. Ip, P. R. Cullis and S.-D. Li, Mol. Pharm., 2019, 16, 3957–3967 CrossRef CAS PubMed.
- M. B. Tayemeh, M. R. Kalbassi, H. Paknejad and H. S. Joo, Environ. Res., 2020, 185, 109477 CrossRef CAS PubMed.
- R. Löbenberg and G. L. Amidon, Eur. J. Pharm. Biopharm., 2000, 50, 3–12 CrossRef PubMed.
- M. N. Martinez and G. L. Amidon, J. Clin. Pharmacol., 2002, 42, 620–643 CrossRef CAS PubMed.
- A. Dahan, A. Beig, D. Lindley and J. M. Miller, Adv. Drug Delivery Rev., 2016, 101, 99–107 CrossRef CAS PubMed.
- H. Li, L. Dong, Y. Liu, G. Wang, G. Wang and Y. Qiao, Int. J. Pharm., 2014, 466, 133–138 CrossRef CAS PubMed.
- T. Chen, W. Liu, S. Xiong, D. Li, S. Fang, Z. Wu, Q. Wang and X. Chen, ACS Appl. Mater. Interfaces, 2019, 11, 45276–45289 CrossRef CAS PubMed.
- J.-Y. Pang, X. Liu, B.-D. Shen, C.-Y. Shen, W.-Q. Lian, J. Liu, C.-X. Hu, R.-N. Zhong, R.-C. Xu and H.-L. Yuan, Zhongguo Zhongyao Zazhi, 2017, 42, 2473–2478 Search PubMed.
- R. Gref, A. Domb, P. Quellec, T. Blunk, R. H. Müller, J. M. Verbavatz and R. Langer, Adv. Drug Delivery Rev., 1995, 16, 215–233 CrossRef CAS PubMed.
- S. Stolnik, L. Illum and S. S. Davis, Adv. Drug Delivery Rev., 1995, 16, 195–214 CrossRef CAS.
- J. Shen, Y. Wang, Q. Ping, Y. Xiao and X. Huang, J. Controlled Release, 2009, 137, 217–223 CrossRef CAS PubMed.
- X. Liu, Z. Zhang, Y. Jiang, Y. Hu, Z. Wang, J. Liu, R. Feng, J. Zhang and G. Huang, Drug Delivery, 2015, 22, 223–229 CrossRef CAS PubMed.
- J.-Q. Ruan, W.-I. Leong, R. Yan and Y.-T. Wang, J. Agric. Food Chem., 2010, 58, 5770–5776 CrossRef CAS PubMed.
- L. Tang, Q. Feng, J. Zhao, L. Dong, W. Liu, C. Yang and Z. Liu, Food Chem. Toxicol., 2012, 50, 1460–1467 CrossRef CAS PubMed.
- V. P. Torchilin, Nat. Rev. Drug Discovery, 2005, 4, 145–160 CrossRef CAS PubMed.
- A. Jhaveri, P. Deshpande, B. Pattni and V. Torchilin, J. Controlled Release, 2018, 277, 89–101 CrossRef CAS PubMed.
- D. K. Mishra, R. Shandilya and P. K. Mishra, Nanomedicine, 2018, 14, 2023–2050 CrossRef CAS PubMed.
- F. Shi, J.-H. Zhao, Y. Liu, Z. Wang, Y.-T. Zhang and N.-P. Feng, Int. J. Nanomed., 2012, 7, 2033–2043 CrossRef CAS PubMed.
- H. Li, X. Zhao, Y. Ma, G. Zhai, L. Li and H. Lou, J. Controlled Release, 2009, 133, 238–244 CrossRef CAS PubMed.
- M. Xue, Z.-Z. Jiang, T. Wu, M. Yan, J.-P. Liu, X.-M. Mu, Y.-W. Su and L.-Y. Zhang, Arzneimittelforschung, 2011, 61, 571–576 CAS.
- M. Xue, M.-X. Yang, W. Zhang, X.-M. Li, D.-H. Gao, Z.-M. Ou, Z.-P. Li, S.-H. Liu, X.-J. Li and S.-Y. Yang, Int. J. Nanomed., 2013, 8, 4677–4687 CrossRef PubMed.
- X. Tan, Y. Hao, N. Ma, Y. Yang, W. Jin, Y. Meng, C. Zhou, W. Zheng and Y. Zhang, Drug Delivery, 2023, 30, 2219432 CrossRef PubMed.
- Y. Liu and N. Feng, Adv. Colloid Interface Sci., 2015, 221, 60–76 CrossRef CAS PubMed.
- V. Jenning, A. F. Thünemann and S. H. Gohla, Int. J. Pharm., 2000, 199, 167–177 CrossRef CAS PubMed.
- W. Mehnert and K. Mäder, Adv. Drug Delivery Rev., 2001, 47, 165–196 CrossRef CAS PubMed.
- R. H. Müller, M. Radtke and S. A. Wissing, Adv. Drug Delivery Rev., 2002, 54(Suppl 1), S131–S155 CrossRef PubMed.
- A. Saupe, S. A. Wissing, A. Lenk, C. Schmidt and R. H. Müller, Bio-Med. Mater. Eng., 2005, 15, 393–402 CAS.
- K. Rajpoot, Curr. Pharm. Des., 2019, 25, 3943–3959 CrossRef CAS PubMed.
- K. Westesen, H. Bunjes and M. H. J. Koch, J. Controlled Release, 1997, 48, 223–236 CrossRef CAS.
- T. H. Truong, K. P. Alcantara, B. P. I. Bulatao, F. N. Sorasitthiyanukarn, C. Muangnoi, N. Nalinratana, O. Vajragupta, P. Rojsitthisak and P. Rojsitthisak, Carbohydr. Polym., 2022, 288, 119401 CrossRef CAS PubMed.
- Q. Kang, J. Liu, Y. Zhao, X. Liu, X.-Y. Liu, Y.-J. Wang, N.-L. Mo and Q. Wu, Artif. Cells, Nanomed., Biotechnol., 2018, 46, S585–S597 CrossRef CAS PubMed.
- D. Kebebe, Y. Wu, B. Zhang, J. Yang, Y. Liu, X. Li, Z. Ma, P. Lu, Z. Liu and J. Li, Int. J. Nanomed., 2019, 14, 6179–6195 CrossRef CAS PubMed.
- Y. Gu, X. Tang, M. Yang, D. Yang and J. Liu, Int. J. Pharm., 2019, 554, 235–244 CrossRef CAS PubMed.
- S. Sun, Q. Guan, E. Shang, H. Xiao, X. Yu, L. Shi, C. Zhao, Y. Guo, S. Lv and Y. Li, Pak. J. Pharm. Sci., 2020, 33, 109–119 CAS.
- S.-F. Chen, W.-F. Lu, Z.-Y. Wen, Q. Li and J.-H. Chen, Die Pharmazie, 2012, 67, 781–788 CAS.
- J. Wang, G. Yang, X. Guo, Z. Tang, Z. Zhong and S. Zhou, Biomaterials, 2014, 35, 3080–3090 CrossRef CAS PubMed.
- Y. Yu, X. Zhang and L. Qiu, Biomaterials, 2014, 35, 3467–3479 CrossRef CAS PubMed.
- L. Lv, Y. Shen, M. Li, X. Xu, M. Li, S. Guo and S. Huang, J. Biomed. Nanotechnol., 2014, 10, 324–335 CrossRef CAS PubMed.
- Z. Zheng, J. Zhang, J. Jiang, Y. He, W. Zhang, X. Mo, X. Kang, Q. Xu, B. Wang and Y. Huang, J. Immunother. Cancer, 2020, 8, e000207 CrossRef PubMed.
- J. W. Singer, R. Bhatt, J. Tulinsky, K. R. Buhler, E. Heasley, P. Klein and P. de Vries, J. Controlled Release, 2001, 74, 243–247 CrossRef CAS PubMed.
- Z. Wang, Y. Yu, J. Ma, H. Zhang, H. Zhang, X. Wang, J. Wang, X. Zhang and Q. Zhang, Mol. Pharm., 2012, 9, 2646–2657 CrossRef CAS PubMed.
- K. S. Snima, P. Arunkumar, R. Jayakumar and V.-K. Lakshmanan, J. Biomed. Nanotechnol., 2014, 10, 559–570 CrossRef CAS PubMed.
- J. Xu, J.-H. Zhao, Y. Liu, N.-P. Feng and Y.-T. Zhang, Int. J. Nanomed., 2012, 7, 211–219 CAS.
- N. Shadjou and M. Hasanzadeh, Mater. Sci. Eng., C, 2015, 55, 401–409 CrossRef CAS PubMed.
- A. P. Singh, A. Biswas, A. Shukla and P. Maiti, Signal Transduction Targeted Ther., 2019, 4, 33 CrossRef PubMed.
- P. Yang, S. Gai and J. Lin, Chem. Soc. Rev., 2012, 41, 3679–3698 RSC.
- X. Zhang, Y. Zhu, L. Fan, J. Ling, L.-Y. Yang, N. Wang and X.-K. Ouyang, Int. J. Biol. Macromol., 2022, 211, 368–379 CrossRef CAS PubMed.
- Y. He, S. Liang, M. Long and H. Xu, Mater. Sci. Eng., C, 2017, 78, 12–17 CrossRef CAS PubMed.
- M.-E. Kyriazi, D. Giust, A. H. El-Sagheer, P. M. Lackie, O. L. Muskens, T. Brown and A. G. Kanaras, ACS Nano, 2018, 12, 3333–3340 CrossRef CAS PubMed.
- K. Lee, V. P. Drachev and J. Irudayaraj, ACS Nano, 2011, 5, 2109–2117 CrossRef CAS PubMed.
- J. Chen, M. Gong, Y. Fan, J. Feng, L. Han, H. L. Xin, M. Cao, Q. Zhang, D. Zhang, D. Lei and Y. Yin, ACS Nano, 2022, 16, 910–920 CrossRef CAS PubMed.
- S. Manju and K. Sreenivasan, J. Colloid Interface Sci., 2012, 368, 144–151 CrossRef CAS PubMed.
- J. Bresee, C. M. Bond, R. J. Worthington, C. A. Smith, J. C. Gifford, C. A. Simpson, C. J. Carter, G. Wang, J. Hartman, N. A. Osbaugh, R. K. Shoemaker, C. Melander and D. L. Feldheim, J. Am. Chem. Soc., 2014, 136, 5295–5300 CrossRef CAS PubMed.
- X.-Q. Zhang, X. Xu, R. Lam, D. Giljohann, D. Ho and C. A. Mirkin, ACS Nano, 2011, 5, 6962–6970 CrossRef CAS PubMed.
- H. J. M. Wolff, M. Kather, H. Breisig, W. Richtering, A. Pich and M. Wessling, ACS Appl. Mater. Interfaces, 2018, 10, 24799–24806 CrossRef CAS PubMed.
-
A. Pich and W. Richtering, Chemical Design of Responsive Microgels, Springer Berlin Heidelberg, 2010, pp. 1–37, DOI:10.1007/12_2010_70.
- K. Landfester and A. V. Musyanovych, Adv. Polym. Sci., 2010, 234, 39–63 CrossRef CAS.
- K. Albrecht, M. Moeller and J. Groll, Adv. Polym. Sci., 2010, 234, 65–93 CrossRef CAS.
- F. Krahl and K. F. Arndt, Adv. Polym. Sci., 2010, 234, 95–128 CrossRef CAS.
- N. A. Nafee, F. A. Ismail, N. A. Boraie and L. M. Mortada, Drug Dev. Ind. Pharm., 2004, 30, 985–993 CrossRef CAS PubMed.
- Q. Qian, L. Shi, X. Gao, Y. Ma, J. Yang, Z. Zhang, J. Qian and X. Zhu, Small, 2019, 15, e1903208 CrossRef PubMed.
- G. Wang, C. Yang, K. Zhang, J. Hu and W. Pang, Molecules, 2015, 20, 12376–12388 CrossRef CAS PubMed.
- L. Ke, J. Zhou, W. Lu, G. Gao and P. Rao, Trends Food Sci. Technol., 2011, 22, 492–497 CrossRef CAS.
- Y. Zhuang, J. Yan, W. Zhu, L. Chen, D. Liang and X. Xu, J. Ethnopharmacol., 2008, 117, 378–384 CrossRef PubMed.
- J. Zhou, G. Gao, Q. Chu, H. Wang, P. Rao and L. Ke, J. Ethnopharmacol., 2014, 151, 1116–1123 CrossRef CAS PubMed.
- J. Hu, Z. Wu, J. Yan, W. Pang, D. Liang and X. Xu, J. Ethnopharmacol., 2009, 123, 267–274 CrossRef PubMed.
- X. Huang, X. Liu, X. Lin, Z. Yuan, Y. Zhang, Z. Wang, W. Pi, H. Zhao, H. Lei and P. Wang, J. Nanobiotechnol., 2022, 20, 527 CrossRef CAS PubMed.
- B. Su, Y. Kan, J. Xie, J. Hu and W. Pang, Molecules, 2016, 21, 845 CrossRef PubMed.
- J. Wen, X. Chen, Y. Yang, J. Liu, E. Li, J. Liu, Z. Zhou, W. Wu and K. He, Am. J. Chin. Med., 2021, 49, 1–23 CrossRef PubMed.
- M. Wang, Y.-H. Gao, J. Xu, Y. Chi, X.-B. Wei, G. Lewith and J.-P. Liu, Complement. Ther. Med., 2015, 23, 469–483 CrossRef PubMed.
- T. Sha, L. L. Gao, C. H. Zhang, J. G. Zheng and Z. H. Meng, QJM, 2016, 109, 639–641 CrossRef CAS PubMed.
- X.-K. Xu, C.-S. Jia, J.-L. Wang, J. Shi, L. Qin, X. Zhang and X.-P. Zhang, Zhenci Yanjiu, 2012, 37, 155–160 Search PubMed.
- Y.-H. Zhu and Y.-H. Chen, Zhongguo Zhenjiu, 2005, 25, 46–48 Search PubMed.
- C. Ji, F. Song, G. Huang, S. Wang, H. Liu, S. Liu, L. Huang, S. Liu, J. Zhao, T. J. Lu and F. Xu, Life Sci., 2018, 211, 51–62 CrossRef CAS PubMed.
- J. K. Hurt and M. J. Zylka, Mol. Pain, 2012, 8, 28 CrossRef CAS PubMed.
- N. Goldman, M. Chen, T. Fujita, Q. Xu, W. Peng, W. Liu, T. K. Jensen, Y. Pei, F. Wang, X. Han, J.-F. Chen, J. Schnermann, T. Takano, L. Bekar, K. Tieu and M. Nedergaard, Nat. Neurosci., 2010, 13, 883–888 CrossRef CAS PubMed.
- D. Fan, Q. Guo, J. Shen, K. Zheng, C. Lu, G. Zhang, A. Lu and X. He, Int. J. Mol. Sci., 2018, 19, 376 CrossRef PubMed.
- J. Zhong, Q. Zhang, Z. Zhang, K. Shi, Y. Sun, T. Liu, J. Lin and K. Yang, Nano Res., 2021, 15, 153–161 CrossRef.
- W. Li, Y. Song, X. Liang, Y. Zhou, M. Xu, Q. Lu, X. Wang and N. Li, Biomaterials, 2021, 276, 121063 CrossRef CAS PubMed.
- F. Yan, H. Li, Z. Zhong, M. Zhou, Y. Lin, C. Tang and C. Li, Int. J. Nanomed., 2019, 14, 9113–9125 CrossRef CAS PubMed.
- S. Ren, H. Liu, X. Wang, J. Bi, S. Lu, C. Zhu, H. Li, W. Kong, R. Chen and Z. Chen, J. Nanobiotechnol., 2021, 19, 409 CrossRef CAS PubMed.
- L. Yao, Q. Ye, Y. Liu, S. Yao, S. Yuan, Q. Xu, B. Deng, X. Tang, J. Shi, J. Luo, J. Wu, Z. Wu, J. Liu, C. Tang, L. Wang and N. Xu, Nat. Commun., 2023, 14, 810 CrossRef CAS PubMed.
- F. Lin, Z. Wang, L. Xiang, L. Wu, Y. Liu, X. Xi, L. Deng and W. Cui, Adv. Sci., 2022, 9, e2200079 CrossRef PubMed.
- W. Xu, Y. Xiao, M. Zhao, J. Zhu, Y. Wang, W. Wang, P. Wang and H. Meng, Adv. Sci., 2023, e2302586, DOI:10.1002/advs.202302586.
- D. Zhang, G. Ding, X. Shen, W. Yao, Z. Zhang, Y. Zhang, J. Lin and Q. Gu, Explore, 2008, 4, 170–177 CrossRef PubMed.
- Y.-T. Li, L.-N. Tang, Y. Ning, Q. Shu, F.-X. Liang, H. Wang and G.-J. Zhang, Sci. Rep., 2016, 6, 28018 CrossRef CAS PubMed.
- S.-X. Ma, X.-Y. Li, T. Sakurai and M. Pandjaitan, Nitric Oxide, 2007, 17, 60–68 CrossRef CAS PubMed.
- J.-X. Chen and S.-X. Ma, J. Altern. Complementary Med., 2005, 11, 423–431 CrossRef PubMed.
- S.-H. Hsiao and L.-J. Tsai, J. Acupunct. Meridian Stud., 2008, 1, 42–50 CrossRef PubMed.
- S. Li, K. Chen, Y. Wu, J. Jiao and L. Tao, J. Tradit. Chin. Med., 2003, 23, 127–128 Search PubMed.
- M. Tsuchiya, E. F. Sato, M. Inoue and A. Asada, Anesth. Analg., 2007, 104, 301–307 CrossRef PubMed.
- L. Tang, Y. Li, H. Xie, Q. Shu, F. Yang, Y.-L. Liu, F. Liang, H. Wang, W. Huang and G.-J. Zhang, Sci. Rep., 2017, 7, 6446 CrossRef PubMed.
- J.-H. Zhang, Y. Zhu, X.-H. Fan and B.-L. Zhang, Acta Pharmacol. Sin., 2015, 36, 654–658 CrossRef CAS PubMed.
-
S. Wang, Y. Sun, B. Shan and T. Xie, in Elemene Antitumor Drugs, ed. T. Xie, Elsevier, 2023, pp. 3–31 Search PubMed.
- B. Zhai, Y. Zeng, Z. Zeng, N. Zhang, C. Li, Y. Zeng, Y. You, S. Wang, X. Chen, X. Sui and T. Xie, Int. J. Nanomed., 2018, 13, 6279–6296 CrossRef CAS PubMed.
- J. Li, H. Zeng, Y. You, R. Wang, T. Tan, W. Wang, L. Yin, Z. Zeng, Y. Zeng and T. Xie, J. Nanobiotechnol., 2021, 19, 289 CrossRef CAS PubMed.
- S. Liu, Q. Li, G. Li, Q. Zhang, L. Zhuo, X. Han, M. Zhang, X. Chen, T. Pan, L. Yan, T. Jin, J. Wang, Q. Lv, X. Sui and T. Xie, Cell Death Dis., 2020, 11, 969 CrossRef CAS PubMed.
- N. Kong, M. Deng, X.-N. Sun, Y.-D. Chen and X.-B. Sui, Front. Pharmacol., 2018, 9, 125 CrossRef PubMed.
- M. Zeng, D. Guo, G. Fernández-Varo, X. Zhang, S. Fu, S. Ju, H. Yang, X. Liu, Y.-C. Wang, Y. Zeng, G. Casals and E. Casals, Mol. Pharm., 2023, 20, 886–904 CrossRef CAS PubMed.
- Y. Li, D. Wang, X. Ping, Y. Zhang, T. Zhang, L. Wang, L. Jin, W. Zhao, M. Guo, F. Shen, M. Meng, X. Chen, Y. Zheng, J. Wang, D. Li, Q. Zhang, C. Hu, L. Xu and X. Ma, Cell, 2022, 185, 949–966 CrossRef CAS PubMed.
- E. O. Lallow, N. C. Jhumur, I. Ahmed, S. B. Kudchodkar, C. C. Roberts, M. Jeong, J. M. Melnik, S. H. Park, K. Muthumani, J. W. Shan, J. D. Zahn, D. I. Shreiber, J. P. Singer, Y. K. Park, J. N. Maslow and H. Lin, Sci. Adv., 2021, 7, eabj0611 CrossRef CAS PubMed.
Footnote |
† These authors contributed equally to this work. |
|
This journal is © The Royal Society of Chemistry 2024 |