DOI:
10.1039/D4BM00439F
(Review Article)
Biomater. Sci., 2025, Advance Article
Role of NLRP3 inflammasome in nanoparticle adjuvant-mediated immune response
Received
26th March 2024
, Accepted 4th June 2024
First published on 5th June 2024
Abstract
The nucleotide-binding oligomerization domain (NOD)-like receptor (NLR) family pyrin domain-containing 3 (NLRP3) inflammasome is pivotal in orchestrating the immune response induced by nanoparticle adjuvants. Understanding the intricate mechanisms underlying the activation of NLRP3 inflammasome by these adjuvants is crucial for deciphering their immunomodulatory properties. This review explores the involvement of the NLRP3 inflammasome in mediating immune responses triggered by nanoparticle adjuvants. It delves into the signaling pathways and cellular mechanisms involved in NLRP3 activation, highlighting its significance in modulating the efficacy and safety of nanoparticle-based adjuvants. A comprehensive grasp of the interplay between NLRP3 inflammasome and nanoparticle adjuvants holds promise for optimizing vaccine design and advancing immunotherapeutic strategies.
Introduction
Vaccines are essential public health interventions that protect against serious illness and complications. The outbreak of COVID-19 [caused by severe acute respiratory syndrome coronavirus 2 (SARS-CoV-2)] has raised a massive demand for the development of vaccines.1,2 The successful cooperation between multi-disciplinary research, such as virology, biology, chemistry, medicine, bioengineering, computational sciences, and nanotechnology, revolutionized vaccine development in record time.3,4 Vaccination is the process of delivering antigenic substances (i.e., live attenuated, inactivated, viral vector, nucleic acid (DNA/RNA), or recombinant protein) to develop the immune response towards specific pathogens and infectious diseases (Fig. 1).5–8 However, in several instances, antigens or antigenic determinants are combined with substances that enhance the antigen-specific immunity regarding the breadth of the immune response and are called “vaccine adjuvants”.9 Despite immunogenicity, the addition of an adjuvant to the vaccine brings several advantages, including potency, reduction in the booster doses, cost, stability, and others.7,10 Adjuvants can provide a swift and efficient immune response and will be effective towards a wide range of pathogens, even with antigenic drift (“The genetic diversity in viruses occurs due to the accumulation of mutations in the genes responsible for coding virus-surface proteins that are recognized by host antibodies.”).11 Adjuvants are classified broadly as immunomodulatory molecules (including mineral salts, microbial products, cytokines, glycolipids [saponins], polymers, and others.) and non-immunostimulatory delivery systems (such as lipid nanoparticles [LNPs], emulsions, and others.) or combinations of both.12,13
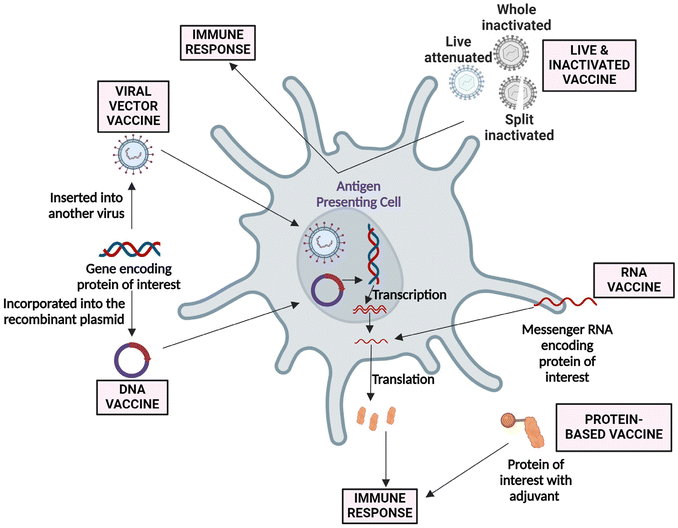 |
| Fig. 1 Approaches followed in developing live, viral vector, DNA, RNA, and protein-based vaccine for SARS-CoV-2. Currently, most of the vaccines are in the clinical phase. An adenovirus-based vaccine (Ad5-nCoV) has been approved for military usage, and an RNA-based vaccine (mRNA-1273) is gearing up for phase III clinical trial.14 | |
Since 1920, various forms of aluminum salts (alum) containing adjuvants have been used as successful adjuvants in many human vaccines, such as tetanus, diphtheria toxoids, and others.15,16 In general, antigens are bound to the adjuvants via non-covalent binding including electrostatic, hydrophobic interactions, hydrogen bonding, and van der Waals forces.17 Later in the 1990s, new adjuvants, i.e., the oil-in-water emulsion system (MF59), were developed to deliver influenza vaccine for the elderly.18 Emulsion adjuvants are based on either oil-in-water or water-in-oil and a three-phase water-oil-water system, where purified mineral oils and biodegradable oils (e.g., squalene, squalane, and others) are used as oils in such emulsions.17 Further, liposome-based adjuvant (AS01) was used in the herpes zoster vaccine. AS01 is composed of two lipids, 3-O-desacyl-4′-monophosphoryl lipid A (MPL), a toll-like receptor 4 (TLR 4) agonist, and QS-21 (from Quillaja saponaria), which trigger the activity of NLRP3 inflammasome pathway.19 Later, the hybrid adjuvant AS04 (combination of MPL lipid and aluminum hydroxide) was developed for human hepatitis B and human papillomavirus vaccines.9,20 Further, in 1994, virosomes or virus-like particles (VLPs) purified from the influenza virus, which are spherical vesicles (∼150 nm size) consisting of the lipid membrane and envelope proteins successfully approved as adjuvants for human vaccines.21 The sudden and unexpected COVID-19 outbreak prompted scientists and pharmaceutical firms to expedite the search for new adjuvants and innovative vaccine approaches to swiftly prevent SARS-CoV-2 infection. In this regard, the protein- or nucleic acid (mRNA or DNA)-based vaccines were seriously studied for the developing vaccines against COVID-19 due to their simplicity and facile scale-up possibility compared to inactivated vaccines. Undoubtedly, the knowledge gained from the development of COVID-19 vaccines will have a profound influence on the future development of vaccines, particularly those utilizing mRNA technology and LNPs.1,2 The use of mRNA technology reduced the cell culture experiments and lowered the chances of contamination compared to other conventional complicated vaccine methods.1,22 LNPs, used to deliver the nucleic acids, are known to act as vaccine adjuvants. A handful of studies have been published on the innate immune response of empty LNPs.3,23,24 In addition, some novel adjuvants have been approved for COVID-19 vaccines, and several have been in clinical trials. A saponin-based adjuvant (i.e., Matrix-M) was used to develop the recombinant protein vaccine (NVX-CoV2373) under clinical evaluation in Phase II.25 A recent study in 2020 reported the usage of alum as an adjuvant in an inactivated vaccine against SARS-CoV-2 developed by Beijing-based Sinovac Biotech, which is in clinical trials.26 This review encompasses the studies conducted by multiple scientists investigating a diverse range of adjuvants, mainly focusing on nanomaterials. These adjuvant nanomaterials play a crucial role in activating the NLRP3 inflammasome and stimulating the immune response in the host, which is advantageous in the field of vaccinology.
In our day-to-day life, people come in contact with various microorganisms, leading to multiple diseases/infections, some of which are life-threatening. To protect and keep ourselves healthy, we need efficacious vaccines that can help us fight against these infections. Earlier vaccines were not so potent, and to address the same, scientists have investigated ways to produce more potent vaccines that are also cost-effective and provide a rapid and effective immune response. The idea was to add adjuvants as they fulfil all the criteria to develop potent and effective vaccines.
Adjuvants play a crucial role in modern vaccine development by enhancing immunity and improving safety.27 Adjuvants can be organic or inorganic derivatives, including complete Freund's adjuvant, a typical example using naturally occurring mineral aluminum salts or bacterial elements.28 However, not all types of vaccines contain adjuvants; for example, the subunit vaccines often lack adjuvants, thereby showing less immunogenicity.28,29 Most vaccines today contain adjuvants approved for human use that enhance and prolong the immune response. Further, the molecular mechanisms by which adjuvants work still need to be fully understood. Particulate adjuvants are essential, especially in directing the adaptive immune response to vaccine antigens. Aluminum hydroxide is one of the most widely used adjuvants in clinical practice.15,16 This adjuvant helps boost immune responses and increase the efficacy of vaccines as shown in Fig. 2. However, researchers are exploring various particulate adjuvants to replace the commonly used “alum”. While alum is not an optimal adjuvant for all protein antigens and has limited ability to induce cell-mediated immunity, other adjuvants, such as chitosan, liposomes, biodegradable microparticles, and nanoparticles, are showing promising results.30,31 These alternative adjuvants can improve the immune response, leading to the development of efficient vaccines.
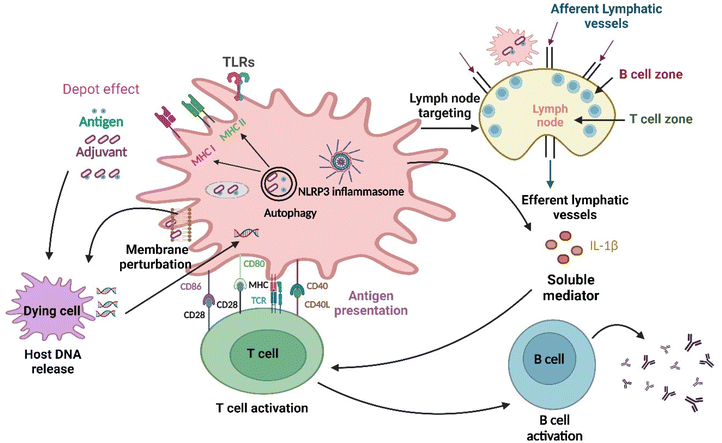 |
| Fig. 2 Different pathways through which nanomaterials activate the immune system: at first, the antigen is released into the bloodstream at a rate regulated by adjuvants, which is the depot effect, and subsequently, the NLRP3 inflammasome gets activated. Changes in the lipid membrane structure also occur in the dendritic cell membrane, leading to the activation of an abortive phagocytic response. The delivery of extracellular and intracellular antigens to major histocompatibility complex (MHC)class II and I molecules for presentation to CD4+ T and CD8+ T lymphocytes, respectively, is facilitated by autophagic regulation.32 Further nanoparticles as adjuvants are delivered to the draining lymph nodes (primary site for immune activation), which target the immune cells residing in them and activate those cells.33 Furthermore, innate and adaptive immune responses are activated by the TLR4 with help from the nanoparticles. The development of humoral immunity by repetitive antigen display on the B cell receptor leads to co-aggregation, triggering, and activation of B cells. The cell-based immunity differentiation of T cells occurs in Th1 or Th2 effector cells. Dendritic cells (highly specialized antigen-presenting cells) can take up foreign material in the extracellular milieu alongside MHC molecules, such as MHC I and MHC II, and also leads to cross-presentation, which is the shuttling of antigen to MHC class I processing pathway for the activation of CD8+ T cell. DNA released following cell damage can also trigger an innate immune response. Lastly, soluble mediators, such as cytokines, chemokines, and immunomodulatory substances, may impact innate and adaptive immune activity, including T cell polarization.34 | |
Role of NLRP3 inflammasome in physiology and pathology
Inflammasomes are crucial in the immune system and are essential for activating inflammatory caspases.35 These protein complexes contain specialized pattern recognition receptors (PRRs) that bind to pro-caspase-1 with the help of an adaptor molecule called apoptosis speck-like protein that contains a caspase activation and recruitment domain (ASC).36,37 Understanding the functioning of inflammasomes can help us develop new approaches to combat inflammatory diseases and promote overall health.38 There are two types of inflammasomes: the ‘canonical inflammasomes’ and the ‘non-canonical inflammasomes’. The ‘canonical inflammasomes’, which include NLRP1, NLRP3, NLRC4, AIM2, and pyrin, are well-known. The ‘non-canonical inflammasomes’ comprise caspase-11 in mice and caspase-4 or -5 in humans.35,39 In general, inflammasomes recognize various pathogen-associated molecular patterns (PAMPs), damage-associated molecular patterns (DAMPs), or any other disturbances in cellular homeostasis.40 The activation of pro-caspase-1 by all canonical inflammasomes leads to the cleavage of pro-inflammatory cytokines, such as interleukin (IL)-1β and IL-18, resulting in the secretion of their active forms. Activated caspase-1 also cleaves a pore-forming protein called Gasdermin D (GSDMD), which creates a large plasma membrane pore in the cell where the inflammasome was activated.41,42 This pore allows for the fast release of IL-1β and IL-18, which leads to cell swelling, resulting in a pro-inflammatory form of cell death called “Pyroptosis”. Furthermore, pyroptosis extends the inflammatory response by releasing more DAMPs and alarmins, including IL-1α.43 The downstream response triggered by all these canonical inflammasomes is a pivotal process in the immune system's response, and its understanding can lead to the development of more effective treatments for inflammatory diseases.38
The mechanism of action of particulate adjuvants is not entirely understood yet. Still, recent studies have highlighted the potential of biodegradable poly(lactic-co-glycolic acid) (PLGA), polystyrene microparticles, and nanoparticles, when combined with TLR agonists, in significantly boosting NLRP3-dependent IL-1β production by murine dendritic cells (DCs).44 These discoveries are a significant step, as they are a progressive step towards understanding the mechanisms underlying inflammasome activation. Overall, these findings could lead to the development of new and effective methods for enhancing immune responses.45 It has been noted that the inclusion of a TLR agonist is necessary for adjuvant-induced IL-1β secretion in vitro.46 However, the adjuvants alone can also stimulate IL-1β secretion in vivo, indicating that endogenous factors can also play a role in the process.47
Recent research has demonstrated that particulate adjuvants can activate the NLRP3 inflammasome, a significant breakthrough. While the mechanisms leading to this activation have been partially resolved, the scientific community is presently involved in understanding the role of NLRP3 in inducing adaptive immunity through particulate adjuvants.48 NLRP3, a protein-coding gene, encodes a pyrin-like protein containing a pyrin domain, a nucleotide-binding site (NBS) domain, and a leucine-rich repeat (LRR) motif.49 This protein interacts with the apoptosis-associated speck-like protein PYCARD/ASC, which contains a caspase recruitment domain and is a component of the NLRP3 inflammasome complex. This complex acts as an upstream activator of NF-κB signaling and is crucial in regulating inflammation, immune response, and apoptosis.50 The NLRP3 inflammasome is essential to the innate immune system and is crucial in protecting humans against microbial infections and cellular damage.51 However, the improper activation of this inflammasome has been linked to various inflammatory disorders, such as Alzheimer's disease, diabetes, atherosclerosis, cancer, cryopyrin-associated periodic syndromes, and others.51,52 To better understand the mechanism behind NLRP3 activation, researchers have identified multiple molecular and cellular events that trigger its activation, including ionic flux, mitochondrial dysfunction, reactive oxygen species production, and lysosomal damage.53,54 The NLRP3 is an important intracellular protein encoded for NOD-, LRR-, and pyrin domain-containing protein 3, which works as a sensor protein for detecting various microbial protein motifs.45 In particular, NLRP3 is responsible for the formation and activation of the NLRP3 inflammasome, thereby resulting in activating the pro-inflammatory response and secreting the IL-1β and IL-18 cytokines via the caspase 1-dependent mechanism and inducing the inflammatory and pyroptotic cell death.45,55 Pyroptosis eliminates infected cells, which stops viruses from sustaining further reproduction in the event of a viral infection. It also liberates the cell's contents, amplifying the innate immune response even more.56 GSDMD plays an essential role in the execution of pyroptosis. GSDMD activation depends on cleavage by caspases 1, 4, 5, 8, and 11, which release the N-terminal fragment, oligomerization, translocation, and ultimately perforation of the plasma membrane.57 NLRP3 activation leads to GSDMD cleavage and allows caspases to cause inflammation and cell death. Despite being protective and essential to host defense, inappropriate activation of the NLRP3 inflammasome results in excessive release of cytokines and a hyperinflammatory response, both locally and systemically.56 For NLRP3 to be an effective therapeutic target in the clinical treatment of inflammatory conditions, further research will be needed focusing on the physiological role of NLRP3 in healthy persons, as its role is not entirely understood.58 However, the function of NLRP3 in physiology is unequivocally proven by preclinical models. They have a role in intestinal homeostasis regulation59 and aging60 and function as a crucial transcription factor in type II helper T cell (Th2) differentiation.61 They also have a role in metabolism, such as regulation of β-cell proliferation, insulin release, and triglyceride levels.58 In the liver, it has been found to negatively regulate the development of non-alcoholic fatty liver disease (NAFLD).62 In cancer, NLRP3 inflammasome is critical in regulating tumor growth by directly triggering pyroptotic cell death or secreting cytokines that cause cell death.63 Apart from its role in physiology, NLRP3 inflammasomes have been involved in many hepatic diseases, such as alcoholic liver disease, non-alcoholic steatohepatitis, viral hepatitis, liver fibrosis, and, most importantly, nanoparticle-induced liver injury.64 Not only in liver diseases, the NLRP3 inflammasome also holds a pivotal position in managing the pathological processes in conditions such as acute myocardial infarction (AMI), systemic lupus erythematosus (SLE), inflammatory bowel disease (IBD), Crohn's disease, bacterial infections, ocular illnesses, and others.65 Further research is needed to fully comprehend how NLRP3 responds to these signaling events and initiates the assembly of the NLRP3 inflammasome. NLRP3 was also found to be involved in autoinflammatory diseases and affects various infections in mice models. The pathogenesis of many acquired inflammatory diseases was also involved with NLRP3 inflammasome activation and regulation.66 Hence, knowledge about the activation and regulation of NLRP3 inflammasome is helping to develop novel pharmacological therapies to target the NLRP3 inflammasome machinery for multiple diseases rapidly.45,66
In vaccines, there is an ingredient called adjuvant, which scientists prefer to use because vaccines become more immunogenic when added. Adjuvants are generally of three types: immunostimulants, delivery systems, and a combination of both. Immunostimulatory adjuvants act by targeting TLRs, cyclin GMP-AMP synthase-stimulators of interferon genes (cGAS-STING) pathway, C-type lectin receptor, and other PRRs like the NOD-like receptors (NLR), whereas delivery systems are described as antigen-loading carrier materials that act by improving antigen-presentation and uptake by antigen-presenting cells (APCs).11,12 Nanoparticles act as immunostimulatory adjuvants and target NLRs, especially NLRP3. Various nanoparticles that activate NLRP3 inflammasomes are carbon nanotubes (CNTs), carbon black, polylactic acid and polystyrene nanoparticles, titanium dioxide (TiO2), silicon dioxide (SiO2), aluminum oxyhydroxide, and many more.67–71 The various mechanisms by which nanoparticles activate the NLRP3 inflammasome are:
Lysosomal damage and cathepsin B release
Nanomaterials cause lysosomal damage, which releases cathepsin B, which directly interacts with NLRP3 in the endoplasmic reticulum and activates it.72–74
Activation of TLR4 and NF-κB
Nanoparticles increase the expression of TLR4, which activates NF-κB-inducible kinase (NIK), which in turn phosphorylates IκB kinases (IKKs) and IκB, leading to ubiquitination and degradation of IkB, ultimately activating NF-κB.75,76 It is seen in experiments that NF-κB is a crucial inflammatory activator that sets up the NLRP3-inflammasome for activation by promoting pro-IL-1β and NLRP3 expression.77
Reactive oxygen species generation
Reactive oxygen species (ROS) are essential for cells to respond to stress through signal transduction. If the production of ROS continues to increase, the cellular antioxidant system will become underpowered, resulting in oxidative stress, cell damage, and maybe even cell death. Nanoparticles generating ROS have been shown to induce NLRP3 activation.67,78–82
Plasma membrane perturbation
An essential function of cell membranes is to control substance transport and preserve the dynamic equilibrium of the intracellular environment. Research has demonstrated that nanoparticle molecular interactions with their target cells are essential for maintaining membrane potential and related intracellular processes that might compromise the integrity of the membrane. Various nanoparticles (silica, amorphous aluminum hydroxy phosphate, and others) interact with cells, leading to perturbation of the plasma membrane, which further leads to the NLRP3 activation.83
K+ efflux
Potassium homeostasis is a crucial modulator of programmed cell death. The apoptotic cascade response involves cytosolic potassium efflux and is linked to the response enzymes caspase, cytochrome c, and nucleic acid endonuclease.84 The mechanism that activates NLRP3 due to cellular potassium efflux is triggered by silica, cholesterol, asbestos, monosodium urate, calcium phosphate crystals, and various nanoparticles.85,86 Nanoparticles cause a surge in potassium efflux, easily escaping lysosomes and damaging mitochondria, causing mitochondrial death.87 Activation of the NLRP3 inflammasome in cells can result in the production of the cytokines IL-1β and IL-18 via caspase-1 activation, which is essential for inflammatory-induced programmed cell death called pyroptosis.88,89
The function of NLRP3 inflammasome in vaccine/vaccine adjuvant-induced immune response
Vaccine adjuvant-induced immunogenicity is due to the NLRP3 inflammasome inducing innate signal transduction to adaptive immunity, which leads to protection from various pathogens.90 The function of the NLRP3 inflammasome after getting activated by the adjuvant is the maturity of APCs, which triggers T-cell-mediated responses. Maturation of the APCs, including the loading of the antigen on the major histocompatibility (MHC) molecule, leads to the expression of two co-stimulatory molecules, CD80 and CD86. Other events include increased production and release of stimulatory cytokines (IL-12), which dendritic cells release to polarize naïve T cells. Together, these mechanisms can be seen as a bridge between innate and adaptive immunity, ultimately leading to the differentiation of naïve T-cells into effector and memory cells. However, there is an indirect route also where inflammasome leads to the activation of adaptive immunity by releasing inflammasome-dependent proinflammatory cytokines (IL-1β and IL-18).90–92
Alum adjuvants activate NLRP3 inflammasome
During the initial stages of vaccine development, various aluminum adjuvants, notably aluminum hydroxide (Alhydrogel) and aluminum phosphate (Adju-Phos), were successfully used as primary adjuvants in vaccines against tetanus, pertussis, and diphtheria.93 Additionally, aluminum potassium sulfate (Potash Alum), a mixture of aluminum and magnesium hydroxides (Inject alum), and aluminum hydroxy phosphate sulfate (AHSA) are also part of aluminum-based adjuvants.16 Indeed, the applied alum-based adjuvants are in the form of nanoscale materials against various infectious diseases.94 Although alum-based adjuvants are poor simulators of cell-mediated immunity, their humoral immunity, cost-effectiveness, and high safety profile make them successful in vaccine development.93
A study by Shi et al. compared the immunological effect of a rabies vaccine containing nano-sized aluminum particles (nano alum) and micron-sized aluminum (alum) along with five other adjuvants. Mice immunized with nano-alum showed protection and increased anti-rabies immunoglobulin (IgG) levels over other adjuvants.95 Aluminum hydroxide is one of the best aluminum adjuvants widely used in vaccine development owing to its high safety profile. However, aqueous aluminum hydroxide particles form aggregates, resulting in microparticles (increased size, 1–20 μm). Li et al. synthesized aluminum hydroxide nanoparticles (∼112 nm) conjugated with model proteins (ovalbumin [OVA] and B. anthracis PA) to overcome this limitation. These conjugated nanoparticles induced higher antigen-specific antibody response than micro aluminum hydroxide adjuvants.16 Sun et al. developed γ-aluminum oxyhydroxide (AlOOH) nanorods functionalized with ovalbumin as a model protein to study the immune response. To understand the NLRP3 trigger mechanism in THP-1 cells and murine bone marrow-derived dendritic cells (BMDCs), vivid AlOOH nanorods were synthesized with different shapes, crystallinity, and hydroxyl group contents. The results confirmed that nanorods with higher crystallinity showed better cellular uptake by forming agglomerates, wherein the shape, crystallinity, and hydroxyl content of AlOOH nanoparticles also played an essential role in the NLRP3 trigger mechanism and increased the OVA-specific immune response as shown in Fig. 3A.96
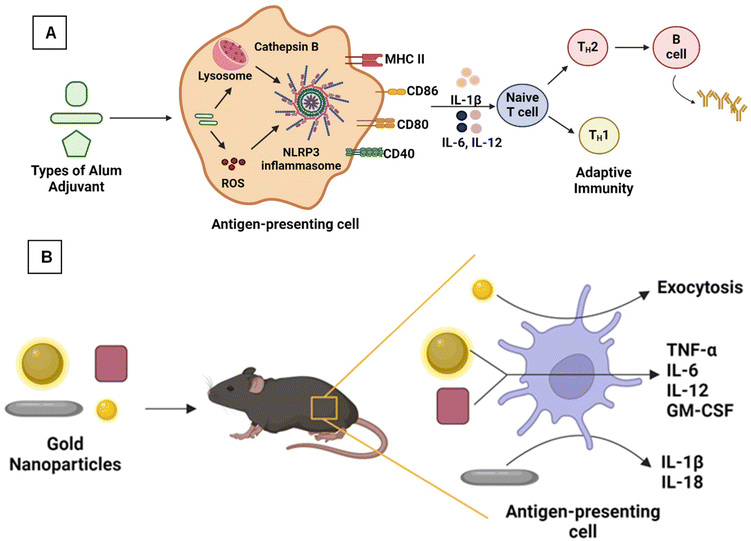 |
| Fig. 3 (A) Aluminum oxyhydroxide nanoparticles showing an adaptive immune response96 and (B) gold nanoparticles of different morphology producing antibodies specific to West Nile virus.97 | |
Similarly, aluminum phosphate is also considered a commonly used aluminum salt-based adjuvant. Vrieling et al. synthesized aluminum phosphate nanoparticles by sonicating the commercially available aluminum phosphate.98 Nanoparticles are stabilized with amino acids, such as threonine, asparagine, aspartic acid, and L-alanyl-L-1-aminoethylphosphonic acid (LAPA), with size distribution in the 400–600 nm range. The adsorption capacity of the particles was studied using lysozyme as a model protein, and it became slightly reduced after the functionalization with asparagine. The immune response induced by the aluminum phosphate particles was analyzed by immunizing mice with diphtheria toxoid adjuvanted with the particles. Only the arginine functionalization increased the specific IgG levels but didn't increase the diphtheria-neutralizing antibodies.98 Lebre et al. developed chitosan-aluminum nanoparticles (CH-Al NPs) with a mean diameter of 280 nm, a positive surface charge, and better stability. Chitosan (CH) is a natural polysaccharide used for various bio-medical applications because of its low toxicity and biocompatibility.99 The in vitro studies showed the low toxicity of the particles. The in vivo studies showed that mice immunized with hepatitis-B antigen (HBsAg) adsorbed CH-Al NPs showed a higher level of anti-HBsAg IgG.99 Later, the same group tried to find out the mechanism of action of CH-Al NPs by undertaking similar in vitro and in vivo studies and found that CH-Al NPs showed NLRP3 activation mechanism (Fig. 3A), which induced cytokine secretion. It is concluded that CH-Al NPs induce innate and adaptive immune responses and can act as an efficient vaccine adjuvant, compared with alum and CH NPs.
Nanoparticle adjuvants activate NLRP3 inflammasome
Using nanoscale materials as an antigen delivery system increases their targeting ability and bioavailability, leading to increased uptake by the APCs, thereby presenting a better release profile of antigenic material.12 Further, nanoscale materials are garnering attraction as nano-immunomodulators owing to their unique properties, such as immune suppression or simulating activity.47 As shown in Fig. 4, various nanoscale adjuvants, including metal and metal oxide-based vaccine adjuvants (e.g., aluminum, gold, silver, SiO2),95,100,101 polymeric (e.g., chitosan, polystyrene, etc.) nanoparticles,102–104 and carbon-based materials, such as nanodiamonds and carbon nanotubes, have been developed.101,105
 |
| Fig. 4 Representation of different nanomaterials/nanoparticles used as vaccine adjuvants. | |
Gold NPs (AuNPs) are widely explored materials in the biomedical field because of their unique physicochemical properties. Niikura et al. studied the effect of the size and shape of AuNPs in producing immunological responses.97 The spherical, rod, and cubic-shaped AuNPs were studied in vivo and in vitro. The AuNPs were coated with West Nile Virus Envelope (WNVE) to understand the immune response. The 40 nm spherical AuNPs showed comparatively higher amounts of WNVE-specific antibodies in the mice. Whereas rods showed the highest level of internalization by APCs and induced the production of inflammatory cytokines (IL-1β and IL-18), as shown in Fig. 3B.97 In a similar study, Vandebriel et al. compared the effect of the shape of AuNPs in NLRP3 inflammasome activation. PEGylated gold nanorods, nanostars, and nanospheres were exposed to differentiated THP-1 cells (wildtype, ASC, or NLRP3-deficient), and then the cells were studied for gene expression analysis. NLRP3 inflammasome activation by gold nanorods decreased sterol/cholesterol biosynthesis, oxidative phosphorylation, and purinergic receptor signaling. A notable finding at the individual gene level was the low production of the protein paraoxonase-2 (PON2), which is responsible for reducing oxidative stress and possibly related to increased ROS production.100 In conclusion, these two studies proved that the surface area of these differently shaped and sized particles plays a prominent role in the immune response and inflammatory cytokine production.97,100 Like AuNPs, silver nanoparticles (AgNPs) are also used as vaccine adjuvants. Xu et al. investigated the immunological effect of pristine AgNPs as vaccine adjuvants in both in vivo and in vitro conditions by using ovalbumin (OVA) and bovine serum albumin as model antigens. The mice immunized intraperitoneally (i.p.) with AgNPs showed increased levels of antigen-specific IgG, which depends upon the level of NPs used. Also, the NPs displayed Th2-biased immune responses. Finally, they have concluded that the immune response exhibited by the AgNPs is due to the recruitment and activation of local leukocytes, mainly macrophages.101
Polymeric nanomaterials are another class of nanomaterials being explored in the biomedical field. Polymers are considered one of the best biomaterials, mainly because of their flexible physical and mechanical properties, achieved by varying the chemistry.106 Among them, PLGA is a widely used biomedical copolymer. Desai et al. synthesized PLGA-based nanospheres and used Staphylococcal Enterotoxin B (SEB) toxoid as a model vaccine. Both in vitro and in vivo (rabbit) studies of nanospheres containing SEB toxoid have been proven to exhibit enhanced immune response, almost similar to that of alum.107 Gu et al. synthesized PLGA nanoparticles encapsulated with immunopotentiator Angelica sinensis polysaccharide (ASP). The nanoparticle's surface was coated with cationic polymer polyethyleneimine (ASP-PLGA-PEI) so that the positive charge on the nanoparticles would increase the targeting and activation ability towards the APCs, thereby increasing the immune response. The immune response was further studied in vivo using porcine circovirus type 2 (PCV2) as a model protein known to cause porcine circovirus-associated disease (PCAVD).104,108 ASP-PLGA-PEI NPs effectively activated the macrophages and also enhanced antigen uptake by APCs. The in vivo studies showed a higher antigen-specific IgG immune response, indicating that ASP-PLGA-PEI NPs can act as vaccine adjuvants, as shown in the schematic representation of Fig. 5.104 In a work by Wang et al., biodegradable poly (g-glutamic acid) (g-PGA) nanoparticles were used for antigen delivery and activation of the immune system both in vitro and in vivo. FITC-labelled nanoparticles encapsulating Texas Red-labelled ovalbumin (TR-OVA/FITC-NPs) were used to study the cellular intake of OVA. An increased concentration of OVA in the cells was observed compared to control samples. To examine the adjuvant effect of nanoparticles, mice were immunized with human immunodeficiency virus type 1 (HIV-1) p24 encapsulating NPs, which induced HIV-1-specific humoral and cellular immune responses.109
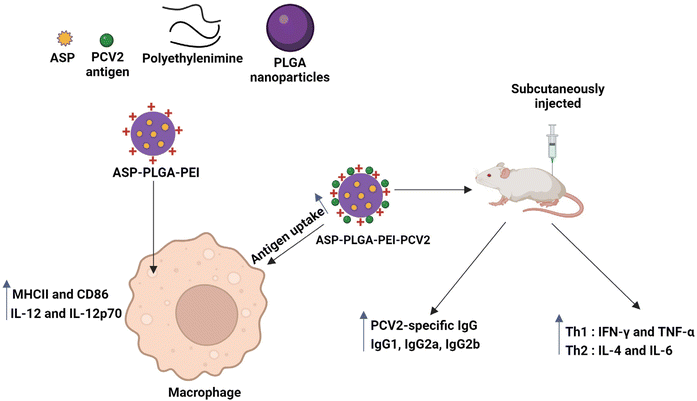 |
| Fig. 5 ASP-PLGA-PEI nanoparticles inducing macrophage activation and antigen uptake by macrophage and also the mice immunized with PCV2 antigen adsorbed ASP-PLGA-PEI showed significant enhancement of PCV2-specific IgG immune response.104 | |
CNTs are one of the allotropic forms of carbon, with a rolled-up graphene layer structure, sometimes with fullerene caps. CNTs are used extensively in different fields, including the biomedical field, because of their unique physicochemical, mechanical, optical, and electronic properties.110 Zhu et al. studied the immune-responsive and adjuvant properties of multi-walled carbon nanotubes (MWCNTs) both in vitro and in vivo.105 The MWCNTs were covalently functionalized with mode antigen ovalbumin (MWCNT-OVA). Increased uptake of MWCNT-OVA by mouse dendritic cells (DC2.4 cell line) was observed along with the upregulation of co-stimulators (CD40/86), the MHCII molecules, and the CD11c molecules. In mice immunized with MWCNT-OVA, increased levels of OVA-specific IgG level were observed with a medium dose. This can be attributed to the activation of the complement system, increased cytokine secretion, and efficient cellular uptake induced by MWCNT.105 Similarly, Meunier et al. investigated the effect of double-walled carbon nanotubes (DWCNTs) on the pro-inflammatory cytokine (IL-1 family) release in an in vitro model. DWCNTs induced the secretion of IL-1β through NLRP3 inflammasome activation in LPS-primed human monocytes but not TNFα or IL-6.111 NLRP3 inflammasome activation was mainly caused by the potassium efflux and phagocytosis processes. These studies have concluded that DWCNTs induce NLRP3 inflammasome activation. So, the potential application of this material in the future should be further studied.111 Several nanoparticle adjuvants are discussed in Table 1, along with different sizes, shapes, and model antigens used.
Table 1 Summarizes various nanomaterial adjuvants, their specific properties, and their applications in different biological models
Nanomaterial adjuvants |
Shape |
Model used |
Comments/summary |
Ref. |
Nanoalum (190 nm) |
— |
A titer of inactivated rabies virus of aG strain (108 TCID50 per ml). It is ultrafiltrated and inactivated |
Nanoalum showed better effectiveness over other adjuvants due to complex formation with antigen. |
95
|
Aluminum hydroxide (112 nm) |
|
Ovalbumin Bacillus anthracis Mouse BMDCs. |
The aluminum hydroxide NPs adjuvant activity is stronger than that of the conventional aluminum hydroxide microparticles |
16
|
γ-Aluminum oxyhydroxide (∼20 nm and lengths of 150–200 nm) |
|
THP-1 & BMDC |
AlOOH nanorods are the most redox-active materials with low crystallinity and the highest hydroxyl content, which activate the NLRP3 inflammasome and cause BMDCs and THP-1 cells to produce IL-1β. |
96
|
Aluminum phosphate NPs (stabilized) {200–300 nm} |
— |
Model antigen (lysozyme) |
Aluminum phosphate was stabilized by threonine, asparagine, and L-alanyl-L-1-aminoethylphosphonic acid |
98
|
Chitosan aluminum (CH-Al) nanomaterials {280 nm} |
|
Hepatitis B surface antigen (HBsAg) |
CH-NP formulations modulated dendritic cell cytokine production. CH-Al NPs activated the NLRP3 inflammasome, increasing IL-1β release, suppressing type 1 T helper (Th1) and Th17 cell-polarizing cytokines, IL-12p70 or IL-23. They did not enhance pro-inflammatory cytokine production but increased dendritic cell maturation. |
99
|
Gold NPs (AuNP) {20 and 40 nm spheres in diameter, 40 × 10 nm rods 40 × 40 × 40 nm cube} |
|
West Nile virus (WNV) envelope protein |
The 40 nm spherical West Nile virus E-coated AuNP (Sphere40-Es) produced the most significant level of WNVE-specific antibodies. |
97
|
Gold nanorods (40 × 16) nm and (60 × 14) nm |
|
Differentiated THP-1 cells (wildtype, ASC, or NLRP3-deficient) |
Nanorods activated NLRP3 inflammasome. |
100
|
Silver nanoparticles (141 nm) {spherical} |
|
Ovalbumin and bovine serum albumin. |
AgNPs increased antigen-specific IgG production in a nanoparticle concentration-dependent fashion. |
101
|
Polylactic polyglycolic acid copolymer (PLGA) (100–150 nm) |
|
Staphylococcal Enterotoxin B (SEB) toxoid |
This study suggested the use of biodegradable nanosphere as a vaccine adjuvant. |
107
|
Angelica sinensis polysaccharide-Polylactic polyglycolic acid-Polyethyleneimine (ASP-PLGA-PEI) {201.7–278.6 nm} |
|
Fluorescein isothiocyanate-labelled ovalbumin (FITC-OVA) |
The ASP-PLGA-PEI nanoparticles notably stimulated macrophages. This nanoparticle promotes the expression of MHCII, and CD86, producing IL-1β and IL-12p70 |
104
|
Poly (gamma-glutamic acid) nanoparticles {200 nm} |
— |
Ovalbumin |
Poly (g-glutamic Acid) nanoparticles have a good potential for AIDS Vaccine |
109
|
Multi-walled carbon nanotubes {200–400 nm} |
|
Ovalbumin |
MWCNTs improve the immune response and have great qualities for use as an adjuvant |
105
|
Double-walled carbon nanotubes (DWCNTs) {0.5–2.5 nm, 1.2–3.2 nm} |
|
LPS-primed human monocytes |
In LPS-primed human monocytes, DWCNTs promote IL-1β and IL-18 production but not TNFα or IL-6 |
111
|
Mesoporous Silica SBA-15 {2 μm long, 10 to 12 nm internal diameter} |
|
Bovine serum albumin as a model antigen |
SBA-15 induced co-production of IgG2a and IgG1 isotypes in mice model, which is higher than aluminum hydroxide salts in comparison |
112
|
Biodegradable vaccines adjuvants
To avoid the long-term toxicity of adjuvants, an ideal adjuvant should not have a negative health impact and should exhibit properties such as unreactive (neutral), biodegradable, and fast excretable from the body.113,114 Therefore, biodegradable vaccine adjuvants represent a promising avenue in vaccine development, offering enhanced efficacy and safety profiles compared to traditional adjuvants.115 These adjuvants are designed in a way that would boost the immune response to vaccines while also degrading in the body over time and minimizing the risk of long-term adverse effects. By employing biocompatible and biodegradable materials, such as polymers or lipids, these adjuvants can be engineered to gradually degrade and be eliminated from the body, reducing the risk of chronic inflammation and autoimmune reactions.114 Several studies have demonstrated the efficacy of biodegradable adjuvants (e.g., Mn3(PO4)2 nanoparticle – nano-MnP) in enhancing the immune response to vaccines.116 For example, chitosan was found to be a potential biodegradable vaccine adjuvant due to its high biocompatibility and biodegradability. It has a good safety profile and enhances adjuvanticity by inducing Ag-specific IgG1/IgG2a and Th1/Th2/Th17 responses.117 Chitosan was shown to activate NLRP3-dependent IL-1β secretion in bone marrow-derived dendritic cells (BMDCs), bone marrow-derived macrophages (BMDMs), and human peripheral blood mononuclear cells.118–120 Besides the NLRP3 inflammasome pathway, chitosan also stimulates the cyclic GMP-AMP synthase (cGAS) – a stimulator of the interferon genes (STING) pathway to boost cellular immunity. When combined with CpG (cytosine phosphoguanine), chitosan-enhanced Ag-specific Th1, Th17, and IgG2 responses significantly depended on the NLRP3 inflammasome in vivo. Furthermore, the adjuvant properties of chitosan were also evaluated in combination with aluminum salts, and the resulting composite chitosan-aluminum sulfate nanoparticles were shown to induce IL-1β production in BMDCs via NLRP3- and ASC-dependent mechanisms.121
Possible risks to health using commercial adjuvants and new nanoparticle-based adjuvants
Despite benefits, health risks are associated with health when using adjuvants. The most potential side effect of adjuvants is the autoimmune/inflammatory syndrome induced by adjuvants (ASIA) first described by Shoenfeld et al. in the year 2011.122 This syndrome comprises various immune-mediated illnesses that are more prone to arise in genetically predisposed individuals following exposure to adjuvants. The key characteristic of this illness is the development of autoantibodies.123 Aluminum adjuvants have been linked to a disease known as macrophagic myofasciitis (MMF) in people who have received hepatitis A and B and tetanus toxoid vaccinations. MMF patients experience arthromyalgias, persistent tiredness, muscular weakness, and even multiple sclerosis.124 There are various types of aluminum adjuvants that differ in shape and size and might have a toxicologic effect on the body, and evaluation of the toxicity is critical. Badran et al. conducted a study to check the safety (toxicological evaluation) of highly used aluminum adjuvants such as Al oxyhydroxide (AlOOH) and Al hydroxyphosphate (AlOHPO4) having different physicochemical properties.125 The toxicology results confirmed that the toxicity of both these Al-based adjuvants depends on the size, charge and shape.125 Though gold nanoparticles exhibited high cell uptake and caused immune stimulation via proinflammatory cytokine generation, their long-term cytotoxicity and in vivo biodistribution, excretion, and possible in vivo biodegradation are not completely understood.126–128 Adjuvants in veterinary vaccines show systemic, nonspecific side effects, including fever, arthritis, uveitis, anorexia, discomfort, and tiredness.129 In human studies, alum adjuvants showed side effects, such as local site response and flu-like symptoms, while CpG/DNA adjuvants headache is the commonly reported side effect. Systemic reactogenicity is also a concern for commercial adjuvants such as oil emulsions and saponins that promote local tissue damage. The various systemic reactogenicity include headache, fever, malaise, diarrhea, arthralgia, myalgia, and lethargy.130 From these multiple studies, it is clear that adjuvants have some side effects, so they must be tested preclinically to check their immunological and toxicological safety.
Future perspective
Adjuvants in vaccines play a huge role in providing immunity. However, due to various restrictions and limitations, a small number of adjuvants compatible with humans pass the test (clinical trials) and get approved. Generally, as seen from numerous studies in the scientific community, the use of natural components is more in making an adjuvant. In the future, synthetic components could be used, which will be better than natural ones. Our understanding of the various pathways, among which NLRP3 is a major one, provides us knowledge as to how to develop a suitable vaccine adjuvant. Continuous innovation in technology and research will make nanoparticle adjuvants safer and cost-effective with minimal side effects and protect us with the highest level of immunity. The possible in vivo biodegradability of various nanoscale-based materials’ potential for vaccine adjuvants (e.g., gold, silver, and others) must be carefully studied to understand the long-term fate of the vaccine adjuvants and their toxicity on humans.131 However, adjuvant-free vaccines have also recently been reported for COVID-19 using modified antigenic protein, i.e., oxidized N-glycan of SARS-CoV-2 receptor-binding domain (RBD) glycoprotein.132 This work opened the door for developing adjuvant-free vaccines using chemically modified antigenic materials.
Conclusions
This review summarizes the critical role of NLRP3 inflammasome in the immune response of different nanoparticle vaccine adjuvants. The mechanism of action of different nanomaterials like aluminum hydroxide NPs, inorganic nanoparticles like AuNPs and AgNPs, polymeric nanomaterials, and carbon-based nanocomposites with NLRP3 inflammasome is extensively discussed. Aluminum hydroxide NPs conjugated with model proteins induced higher antigen-specific antibody response than micro aluminum hydroxide adjuvants. It is also concluded that the chitosan-aluminum nanoparticles are more efficient vaccine adjuvants than the chitosan nanoparticles. Gold nanoparticles play a significant role in immune response and inflammatory cytokine production, and studies have proved that the shape and size of these particles determine the efficiency of their response. Alum is considered one of the best vaccine adjuvants and both in vitro and in vivo studies have shown that the polymeric PLGA nanospheres have similar efficiency in immune response. In like ways, carbon-based nanomaterials have also been attributed to the activation of cytokine secretion and increased cellular uptake. Therefore, this review gives a wholesome summary of different nanomaterials and their efficiency as vaccine adjuvants.
Conflicts of interest
There are no conflicts to declare.
Acknowledgements
R. K. wants to acknowledge the funding sources of the Science and Engineering Research Board under the Department of Science and Technology (DST), Government of India, project number EEQ/2022/000614. R. K. also thanks for the financial support through the RLS fellowship (BT/RLF/Re-entry/20/2020) awarded by the Department of Biotechnology, Govt. India. K. Swetha also acknowledges the funding for her PhD from DST-INSPIRE Fellowship by Govt. India (DST/INSPIRE/03/2022/000487). Mr Momitul Ahmed and Bidya Dhar Sahu thank the Department of Pharmaceuticals, Ministry of Chemicals and Fertilizers, Government of India for the necessary support. The authors thank Hari Krishnareddy Rachamala (Department of Molecular Biology, Mayo Clinic, Jacksonville, Florida, United States) for proofreading this manuscript.
References
- E. Dolgin, Nature, 2021, 589, 189–191 Search PubMed.
- P. Ball, Nature, 2021, 589, 16–18 Search PubMed.
- K. Swetha, N. G. Kotla, L. Tunki, A. Jayaraj, S. K. Bhargava, H. Hu, S. R. Bonam and R. Kurapati, Vaccines, 2023, 11, 658 CrossRef.
- B. Lu, J. M. Lim, B. Yu, S. Song, P. Neeli, N. Sobhani, S. R. Bonam, R. Kurapati, J. Zheng, D. Chai and P. Kurungottu, Front. Immunol., 2024, 15, 1332939 CrossRef PubMed.
- B. Sun and T. Xia, J. Mater. Chem. B, 2016, 4, 5496–5509 RSC.
- C. Weiss, M. Carriere, L. Fusco, I. Capua, J. A. Regla-Nava, M. Pasquali, J. A. Scott, F. Vitale, M. A. Unal, C. Mattevi, D. Bedognetti, A. Merkoçi, E. Tasciotti, A. Yilmazer, Y. Gogotsi, F. Stellacci and L. G. Delogu, ACS Nano, 2020, 14, 6383–6406 CrossRef.
- F. Amanat and F. Krammer, Immunity, 2020, 52, 583–589 CrossRef PubMed.
- J. Castrodeza-Sanz, I. Sanz-Muñoz and J. M. Eiros, Vaccines, 2023, 11, 902 Search PubMed.
- N. P. H. Knudsen, A. Olsen, C. Buonsanti, F. Follmann, Y. Zhang, R. N. Coler, C. B. Fox, A. Meinke, U. D'Oro, D. Casini, A. Bonci, R. Billeskov, E. De Gregorio, R. Rappuoli, A. M. Harandi, P. Andersen and E. M. Agger, Sci. Rep., 2016, 6, 19570 CrossRef.
- S. R. Bonam, C. D. Partidos, S. K. M. Halmuthur and S. Muller, Trends Pharmacol. Sci., 2017, 38, 771–793 CrossRef.
- T. Zhao, Y. Cai, Y. Jiang, X. He, Y. Wei, Y. Yu and X. Tian, Signal Transduction Targeted Ther., 2023, 8, 283 CrossRef.
- M. Zhu, R. Wang and G. Nie, Hum. Vaccines Immunother., 2014, 10, 2761–2774 CrossRef.
- R. Sharma, A. Palanisamy, K. Dhama, G. Mal, B. Singh and K. P. Singh, Hum. Vaccines Immunother., 2020, 16, 2944–2953 CrossRef.
- D. S. Chauhan, R. Prasad, R. Srivastava, M. Jaggi, S. C. Chauhan and M. M. Yallapu, Bioconjugate Chem., 2020, 31, 2021–2045 CrossRef PubMed.
- N. W. Baylor, W. Egan and P. Richman, Vaccine, 2002, 20, S18–S23 CrossRef.
- X. Li, A. M. Aldayel and Z. Cui, J. Controlled Release, 2014, 173, 148–157 CrossRef.
- H. F. Stils Jr., ILAR J., 2005, 46, 280–293 CrossRef PubMed.
- D. T. O'Hagan, R. van der Most, R. N. Lodaya, M. Coccia and G. Lofano, npj Vaccines, 2021, 6, 158 CrossRef PubMed.
- A. M. Didierlaurent, B. Laupèze, A. Di Pasquale, N. Hergli, C. Collignon and N. Garçon, Expert Rev. Vaccines, 2017, 16, 55–63 CrossRef PubMed.
- G. Del Giudice, R. Rappuoli and A. M. Didierlaurent, Semin. Immunol., 2018, 39, 14–21 CrossRef PubMed.
- C. Moser, M. Amacker, A. R. Kammer, S. Rasi, N. Westerfeld and R. Zurbriggen, Expert Rev. Vaccines, 2007, 6, 711–721 CrossRef.
- S. S. Rosa, D. M. F. Prazeres, A. M. Azevedo and M. P. C. Marques, Vaccine, 2021, 39, 2190–2200 CrossRef.
- J. Connors, D. Joyner, N. J. Mege, G. M. Cusimano, M. R. Bell, J. Marcy, B. Taramangalam, K. M. Kim, P. J. C. Lin, Y. K. Tam, D. Weissman, M. A. Kutzler, M.-G. Alameh and E. K. Haddad, Commun. Biol., 2023, 6, 188 CrossRef PubMed.
- S. R. Bonam, N. C. Hazell, M. J. Mathew, Y. Liang, X. Zhang, Z. Wei, M.-G. Alameh, D. Weissman and H. Hu, Vaccines, 2024, 12, 543 CrossRef PubMed.
- R. M. Mallory, N. Formica, S. Pfeiffer, B. Wilkinson, A. Marcheschi, G. Albert, H. McFall, M. Robinson, J. S. Plested, M. Zhu, S. Cloney-Clark, B. Zhou, G. Chau, A. Robertson, S. Maciejewski, H. L. Hammond, L. Baracco, J. Logue, M. B. Frieman, G. Smith, N. Patel, G. M. Glenn, M. Adams, M. Arya, E. Athan, I. Berger, P. Bradley, T. Briskin, R. Glover II, P. Griffin, J. Kim, S. Kitchener, T. Klein, A. Leah, I. Leelasena, C. Lemech, J. Lickliter, M. B. Manning, F. Napier-Flood, P. Nugent, S. Thackwray and M. Turner, Lancet Infect. Dis., 2022, 22, 1565–1576 CrossRef PubMed.
- Q. Gao, L. Bao, H. Mao, L. Wang, K. Xu, M. Yang, Y. Li, L. Zhu, N. Wang, Z. Lv, H. Gao, X. Ge, B. Kan, Y. Hu, J. Liu, F. Cai, D. Jiang, Y. Yin, C. Qin, J. Li, X. Gong, X. Lou, W. Shi, D. Wu, H. Zhang, L. Zhu, W. Deng, Y. Li, J. Lu, C. Li, X. Wang, W. Yin, Y. Zhang and C. Qin, Science, 2020, 369, 77–81 CrossRef PubMed.
- S. K. Verma, P. Mahajan, N. K. Singh, A. Gupta, R. Aggarwal, R. Rappuoli and A. K. Johri, Front. Immunol., 2023, 14, 1043109 CrossRef.
- K. Ivanov, E. Garanina, A. Rizvanov and S. Khaiboullina, Pathogens, 2020, 9, 252 CrossRef PubMed.
- A. Vartak and S. J. Sucheck, Vaccines, 2016, 4, 12 CrossRef PubMed.
- D. T. O’Hagan and N. M. Valiante, Nat. Rev. Drug Discovery, 2003, 2, 727–735 CrossRef.
- I. M. van der Lubben, J. C. Verhoef, G. Borchard and H. E. Junginger, Eur. J. Pharm. Sci., 2001, 14, 201–207 CrossRef PubMed.
- N. L. Patterson and J. D. Mintern, Protein Cell, 2012, 3, 911–920 CrossRef.
- J. Lee, S. Kang, H. Park, J. G. Sun, E. C. Kim and G. Shim, Pharmaceutics, 2023, 15, 565 CrossRef.
- A. He, X. Li, Z. Dai, Q. Li, Y. Zhang, M. Ding, Z.-f. Wen, Y. Mou and H. Dong, J. Nanobiotechnol., 2023, 21, 236 CrossRef.
- J. Yao, K. Sterling, Z. Wang, Y. Zhang and W. Song, Signal Transduction Targeted Ther., 2024, 9, 10 CrossRef.
- D. Li and M. Wu, Signal Transduction Targeted Ther., 2021, 6, 291 CrossRef.
- E. S. Alnemri, J. Clin. Immunol., 2010, 30, 512–519 CrossRef.
- Y. Li, H. Huang, B. Liu, Y. Zhang, X. Pan, X.-Y. Yu, Z. Shen and Y.-H. Song, Signal Transduction Targeted Ther., 2021, 6, 247 CrossRef.
- K. P. Downs, H. Nguyen, A. Dorfleutner and C. Stehlik, Mol. Aspects Med., 2020, 76, 100924 CrossRef.
- G. Santoni, C. Cardinali, M. B. Morelli, M. Santoni, M. Nabissi and C. Amantini, J. Neuroinflammation, 2015, 12, 21 CrossRef PubMed.
- D. Zheng, T. Liwinski and E. Elinav, Cell Discovery, 2020, 6, 36 CrossRef CAS PubMed.
- Y. Pan, W. Cai, J. Huang, A. Cheng, M. Wang, Z. Yin and R. Jia, Front. Immunol., 2022, 13, 991044 CrossRef PubMed.
- Y. Huang, W. Xu and R. Zhou, Cell. Mol. Immunol., 2021, 18, 2114–2127 CrossRef PubMed.
- A. M. Weiss, S. Hossainy, S. J. Rowan, J. A. Hubbell and A. P. Esser-Kahn, Macromolecules, 2022, 55, 6913–6937 CrossRef PubMed.
- K. V. Swanson, M. Deng and J. P. Y. Ting, Nat. Rev. Immunol., 2019, 19, 477–489 CrossRef.
- M. Luchner, S. Reinke and A. Milicic, Pharmaceutics, 2021, 13, 142 CrossRef PubMed.
- B. Pulendran, P. S. Arunachalam and D. T. O’Hagan, Nat. Rev. Drug Discovery, 2021, 20, 454–475 CrossRef PubMed.
- J. Fu and H. Wu, Annu. Rev. Immunol., 2023, 41, 301–316 CrossRef PubMed.
- B. Nógrádi, Á. Nyúl-Tóth, M. Kozma, K. Molnár, R. Patai, L. Siklós, I. Wilhelm and I. A. Krizbai, Front. Pharmacol., 2020, 11, 584184 CrossRef PubMed.
- V. Compan, F. Martín-Sánchez, A. Baroja-Mazo, G. López-Castejón, A. I. Gomez, A. Verkhratsky, D. Brough and P. Pelegrín, J. Immunol., 2015, 194, 1261–1273 CrossRef PubMed.
- C. Zhao and W. Zhao, Front. Immunol., 2020, 11, 211 CrossRef PubMed.
- Y. Chen, X. Ye, G. Escames, W. Lei, X. Zhang, M. Li, T. Jing, Y. Yao, Z. Qiu, Z. Wang, D. Acuña-Castroviejo and Y. Yang, Cell. Mol. Biol. Lett., 2023, 28, 51 CrossRef PubMed.
- Y. Yang, H. Wang, M. Kouadir, H. Song and F. Shi, Cell Death Dis., 2019, 10, 128 CrossRef PubMed.
- A. Akbal, A. Dernst, M. Lovotti, M. S. J. Mangan, R. M. McManus and E. Latz, Cell. Mol. Immunol., 2022, 19, 1201–1214 CrossRef PubMed.
- H. M. Hoffman, J. L. Mueller, D. H. Broide, A. A. Wanderer and R. D. Kolodner, Nat. Genet., 2001, 29, 301–305 CrossRef.
- J. Harris and N. A. Borg, Front. Immunol., 2022, 13, 987453 CrossRef.
- J. Ding, K. Wang, W. Liu, Y. She, Q. Sun, J. Shi, H. Sun, D.-C. Wang and F. J. N. Shao, J. Hazard. Mater., 2016, 535, 111–116 Search PubMed.
- R. Coll, L. O’Neill and K. Schroder, Cell Death Discovery, 2016, 2, 1–5 CrossRef.
- S. A. Hirota, J. Ng, A. Lueng, M. Khajah, K. Parhar, Y. Li, V. Lam, M. S. Potentier, K. Ng and M. Bawa, Inflamm. Bowel Dis., 2011, 17, 1359–1372 CrossRef.
- Y.-H. Youm, R. W. Grant, L. R. McCabe, D. C. Albarado, K. Y. Nguyen, A. Ravussin, P. Pistell, S. Newman, R. Carter and A. Laque, Cell Metab., 2013, 18, 519–532 CrossRef PubMed.
- M. Bruchard, C. Rebé, V. Derangère, D. Togbé, B. Ryffel, R. Boidot, E. Humblin, A. Hamman, F. Chalmin and H. Berger, Nat. Immunol., 2015, 16, 859–870 CrossRef PubMed.
- J. Henao-Mejia, E. Elinav, C. Jin, L. Hao, W. Z. Mehal, T. Strowig, C. A. Thaiss, A. L. Kau, S. C. Eisenbarth and M. J. J. N. Jurczak, Nature, 2012, 482, 179–185 CrossRef.
- W. Zhiyu, N. Wang, Q. Wang, C. Peng, J. Zhang, P. Liu, A. Ou, S. Zhong, M. D. Cordero and Y. Lin, Oncotarget, 2016, 7, 50766 CrossRef PubMed.
- J. Luan and D. Ju, Front. Immunol., 2018, 9, 2201 CrossRef.
- Z.-Y. Zhang, X.-L. Bao, Y.-Y. Cong, B. Fan and G.-Y. Li, Oxid. Med. Cell. Longevity, 2020, 2020, 2896036 Search PubMed.
- Y. He, M. Y. Zeng, D. Yang, B. Motro and G. Núñez, Nature, 2016, 530, 354–357 CrossRef PubMed.
- O. Lunov, T. Syrovets, C. Loos, G. U. Nienhaus, V. Mailänder, K. Landfester, M. Rouis and T. Simmet, ACS Nano, 2011, 5, 9648–9657 CrossRef PubMed.
- A. S. Yazdi, G. Guarda, N. Riteau, S. K. Drexler, A. Tardivel, I. Couillin and J. Tschopp, Proc. Natl. Acad. Sci. U. S. A., 2010, 107, 19449–19454 CrossRef PubMed.
- T. Morishige, Y. Yoshioka, A. Tanabe, X. Yao, S.-i. Tsunoda, Y. Tsutsumi, Y. Mukai, N. Okada and S. Nakagawa, Biochem. Biophys. Res. Commun., 2010, 392, 160–165 CrossRef PubMed.
- A. C. Reisetter, L. V. Stebounova, J. Baltrusaitis, L. Powers, A. Gupta, V. H. Grassian and M. M. Monick, J. Biol. Chem., 2011, 286, 21844–21852 CrossRef PubMed.
- J. Palomäki, E. Välimäki, J. Sund, M. Vippola, P. A. Clausen, K. A. Jensen, K. Savolainen, S. Matikainen and H. Alenius, ACS Nano, 2011, 5, 6861–6870 CrossRef PubMed.
- V. Hornung, F. Bauernfeind, A. Halle, E. O. Samstad, H. Kono, K. L. Rock, K. A. Fitzgerald and E. Latz, Nat. Immunol., 2008, 9, 847–856 CrossRef PubMed.
- P. T. Knotigova, J. Mašek, F. e. Hubatka, J. Kotouček, P. Kulich, P. Simeckova, E. k. Bartheldyová, M. Machala, T. Švadláková and J. Krejsek, Mol. Pharm., 2019, 16, 3441–3451 CrossRef PubMed.
- T. Svadlakova, F. Hubatka, P. Turanek Knotigova, P. Kulich, J. Masek, J. Kotoucek, J. Macak, M. Motola, M. Kalbac and M. Kolackova, Nanomaterials, 2020, 10, 418 CrossRef PubMed.
- Y. Ze, L. Sheng, X. Zhao, J. Hong, X. Ze, X. Yu, X. Pan, A. Lin, Y. Zhao and C. Zhang, PLoS One, 2014, 9, e92230 CrossRef PubMed.
- A. M. Mahmoud, E. M. Desouky, W. G. Hozayen, M. Bin-Jumah, E.-S. El-Nahass, H. A. Soliman and A. A. Farghali, Biomolecules, 2019, 9, 528 CrossRef PubMed.
- Z. Zhong, A. Umemura, E. Sanchez-Lopez, S. Liang, S. Shalapour, J. Wong, F. He, D. Boassa, G. Perkins, S. R. Ali, M. D. McGeough, M. H. Ellisman, E. Seki, A. B. Gustafsson, H. M. Hoffman, M. T. Diaz-Meco, J. Moscat and M. Karin, Cell, 2016, 164, 896–910 CrossRef CAS.
- B. Hindman and Q. Ma, Arch. Toxicol., 2019, 93, 887–907 CrossRef PubMed.
- X. Zhang, J. Luan, W. Chen, J. Fan, Y. Nan, Y. Wang, Y. Liang, G. Meng and D. Ju, Nanoscale, 2018, 10, 9141–9152 RSC.
- Q. Chi, T. Xu, Y. He, Z. Li, X. Tang, X. Fan and S. Li, J. Hazard. Mater., 2022, 439, 129502 CrossRef PubMed.
- M. Winter, H.-D. Beer, V. Hornung, U. Krämer, R. P. Schins and I. Förster, Nanotoxicology, 2011, 5, 326–340 CrossRef PubMed.
- L. Zhou, P. Li, M. Zhang, B. Han, C. Chu, X. Su, B. Li, H. Kang, J. Ning and B. Zhang, Chemosphere, 2020, 241, 125075 CrossRef.
- X. Liao, Y. Liu, J. Zheng, X. Zhao, L. Cui, S. Hu, T. Xia and S. Si, Nanomaterials, 2022, 12, 3908 CrossRef PubMed.
- S. P. Yu, Prog. Neurobiol., 2003, 70, 363–386 CrossRef.
- M. N. Darisipudi, D. Thomasova, S. R. Mulay, D. Brech, E. Noessner, H. Liapis and H.-J. Anders, J. Am. Soc. Nephrol., 2012, 23, 1783 CrossRef PubMed.
- E. A. Warren and C. K. Payne, RSC Adv., 2015, 5, 13660–13666 RSC.
- Q. Wang, Y. Sun, Z. Zhang and Y. Duan, Biomaterials, 2015, 56, 229–240 CrossRef PubMed.
- B. Sharma, C. B. McLeland, T. M. Potter, S. T. Stern and P. P. Adiseshaiah, Methods Mol. Biol., 2018, 135–147 CrossRef.
- N. Kelley, D. Jeltema, Y. Duan and Y. He, Int. J. Mol. Sci., 2019, 20, 3328 CrossRef PubMed.
- S. Reinke, A. Thakur, C. Gartlan, J. S. Bezbradica and A. Milicic, Vaccines, 2020, 8, 554 CrossRef.
- J. Harris, F. A. Sharp and E. C. Lavelle, Eur. J. Immunol., 2010, 40, 634–638 CrossRef.
- M. Chen, H. Wang, W. Chen and G. Meng, Int. Immunopharmacol., 2011, 11, 549–554 CrossRef.
- H. Hogenesch, Front. Immunol., 2012, 3, 406 Search PubMed.
- L. Mao, Z. Chen, Y. Wang and C. Chen, J. Inorg. Biochem., 2021, 219, 111454 CrossRef PubMed.
- W. Shi, Y. Kou, J. Xiao, L. Zhang, F. Gao, W. Kong, W. Su, C. Jiang and Y. Zhang, Vaccine, 2018, 36, 5020–5029 CrossRef PubMed.
- B. Sun, Z. Ji, Y.-P. Liao, M. Wang, X. Wang, J. Dong, C. H. Chang, R. Li, H. Zhang, A. E. Nel and T. Xia, ACS Nano, 2013, 7, 10834–10849 CrossRef PubMed.
- K. Niikura, T. Matsunaga, T. Suzuki, S. Kobayashi, H. Yamaguchi, Y. Orba, A. Kawaguchi, H. Hasegawa, K. Kajino, T. Ninomiya, K. Ijiro and H. Sawa, ACS Nano, 2013, 7, 3926–3938 CrossRef.
- H. Vrieling, M. Espitia Ballestas, M. Hamzink, G.-J. Willems, P. Soema, W. Jiskoot, G. Kersten and B. Metz, Colloids Surf., B, 2019, 181, 648–656 CrossRef.
- F. Lebre, D. Bento, J. Ribeiro, M. Colaço, G. Borchard, M. C. P. de Lima and O. Borges, Int. J. Pharm., 2017, 527, 103–114 CrossRef PubMed.
- R. J. Vandebriel, S. Remy, J. P. Vermeulen, E. G. E. Hurkmans, K. Kevenaar, N. G. Bastús, B. Pelaz, M. G. Soliman, V. F. Puntes, W. J. Parak, J. L. A. Pennings and I. Nelissen, Int. J. Mol. Sci., 2022, 23, 5763 CrossRef.
- Y. Xu, H. Tang, J.-h. Liu, H. Wang and Y. Liu, Toxicol. Lett., 2013, 219, 42–48 CrossRef CAS PubMed.
- C. Tomaro-Duchesneau, S. Saha, M. Malhotra, I. Kahouli and S. Prakash, J. Pharm., 2013, 2013, 103527 Search PubMed.
- A.-K. Fuchs, T. Syrovets, K. A. Haas, C. Loos, A. Musyanovych, V. Mailänder, K. Landfester and T. Simmet, Biomaterials, 2016, 85, 78–87 CrossRef PubMed.
- P. Gu, A. Wusiman, S. Wang, Y. Zhang, Z. Liu, Y. Hu, J. Liu and D. Wang, Carbohydr. Polym., 2019, 223, 115128 CrossRef PubMed.
- X. Zhu, J. Sun, Y. Zhang and X. Sun, Immunol. Lett., 2016, 178, 77–84 CrossRef PubMed.
- B. D. Ulery, L. S. Nair and C. T. Laurencin, J. Polym. Sci., Part B: Polym. Phys., 2011, 49, 832–864 CrossRef PubMed.
- M. P. Desai, J. M. Hilfinger, G. L. Amidon, R. J. Levy and V. Labhasetwar, J. Microencapsulation, 2000, 17, 215–225 CrossRef PubMed.
- K. Wu, W. Hu, B. Zhou, B. Li, X. Li, Q. Yan, W. Chen, Y. Li, H. Ding, M. Zhao, S. Fan, L. Yi and J. Chen, Int. J. Mol. Sci., 2022, 23, 14126 CrossRef PubMed.
- X. Wang, T. Uto, T. Akagi, M. Akashi and M. Baba, J. Med. Virol., 2008, 80, 11–19 CrossRef PubMed.
- J. Simon, E. Flahaut and M. Golzio, Materials, 2019, 12, 624 CrossRef.
- E. Meunier, A. Coste, D. Olagnier, H. Authier, L. Lefèvre, C. Dardenne, J. Bernad, M. Béraud, E. Flahaut and B. Pipy, Nanomedicine, 2012, 8, 987–995 CrossRef.
- L. V. Carvalho, R. d. C. Ruiz, K. Scaramuzzi, E. B. Marengo, J. R. Matos, D. V. Tambourgi, M. C. A. Fantini and O. A. Sant'Anna, Vaccine, 2010, 28, 7829–7836 CrossRef CAS.
- D. N. Desai, A. Mahal, R. Varshney, A. J. Obaidullah, B. Gupta, P. Mohanty, P. Pattnaik, N. C. Mohapatra, S. Mishra, V. Kandi, A. A. Rabaan and R. K. Mohapatra, ACS Omega, 2023, 8, 27953–27968 CrossRef CAS PubMed.
- S. Hasanzadeh, M. Farokhi, M. Habibi, M. A. Shokrgozar, R. Ahangari Cohan, F. Rezaei, M. R. Asadi Karam and S. Bouzari, ACS Biomater. Sci. Eng., 2020, 6, 4573–4582 CrossRef CAS PubMed.
- R. J. Bose, M. Kim, J. H. Chang, R. Paulmurugan, J. J. Moon, W. G. Koh, S. H. Lee and H. Park, J. Ind. Eng. Chem., 2019, 77, 12–24 CrossRef CAS.
- S.-H. Zhou, R.-Y. Zhang, Z.-W. You, Y.-K. Zou, Y. Wen, J. Wang, D. Ding, M.-M. Bian, Z.-M. Zhang, H. Yuan, G.-F. Yang and J. Guo, ACS Appl. Mater. Interfaces., 2023, 15, 8914–8926 CrossRef CAS PubMed.
- P. Li and F. Wang, Drug Discoveries Ther., 2015, 9, 88–93 CrossRef CAS.
- E. C. Carroll, L. Jin, A. Mori, N. Muñoz-Wolf, E. Oleszycka, H. B. T. Moran, S. Mansouri, C. P. McEntee, E. Lambe, E. M. Agger, P. Andersen, C. Cunningham, P. Hertzog, K. A. Fitzgerald, A. G. Bowie and E. C. Lavelle, Immunity, 2016, 44, 597–608 CrossRef.
- C. L. Bueter, C. K. Lee, J. P. Wang, G. R. Ostroff, C. A. Specht and S. M. Levitz, J. Immunol., 2014, 192, 5943–5951 CrossRef.
- A. Mori, E. Oleszycka, F. A. Sharp, M. Coleman, Y. Ozasa, M. Singh, D. T. O'Hagan, L. Tajber, O. I. Corrigan, E. A. McNeela and E. C. Lavelle, Eur. J. Immunol., 2012, 42, 2709–2719 CrossRef.
- S. Awate, L. A. Babiuk and G. Mutwiri, Front. Immunol., 2013, 4, 114 Search PubMed.
- Y. Shoenfeld and N. Agmon-Levin, J. Autoimmun., 2011, 36, 4–8 CrossRef.
- A. Watad, K. Sharif and Y. Shoenfeld, Mediterr. J. Rheumatol., 2017, 28, 64–69 CrossRef PubMed.
- F.-J. Authier, P. Cherin, A. Creange, B. Bonnotte, X. Ferrer, A. Abdelmoumni, D. Ranoux, J. Pelletier, D. Figarella-Branger, B. Granel, T. Maisonobe, M. Coquet, J.-D. Degos and R. K. Gherardi, Brain, 2001, 124, 974–983 CrossRef.
- G. Badran, L. Angrand, J. D. Masson, G. Crépeaux and M. O. David, Vaccine, 2022, 40, 4881–4888 CrossRef PubMed.
- L. A. Dykman and N. G. Khlebtsov, Chem. Sci., 2017, 8, 1719–1735 RSC.
- A. Balfourier, N. Luciani, G. Wang, G. Lelong, O. Ersen, A. Khelfa, D. Alloyeau, F. Gazeau and F. Carn, Proc. Natl. Acad. Sci. U. S. A., 2019, 117, 103–113 CrossRef PubMed.
- S. A. C. Carabineiro, Molecules, 2017, 22, 857 CrossRef PubMed.
- A. R. Spickler and J. A. Roth, J. Vet. Intern. Med., 2003, 17, 273–281 CrossRef PubMed.
- N. Petrovsky, Drug Saf., 2015, 38, 1059–1074 CrossRef.
- R. Mateu Ferrando, L. Lay and L. Polito, Drug Discovery Today: Technol., 2020, 38, 57–67 CrossRef PubMed.
- R.-Y. Zhang, S.-H. Zhou, R.-R. Feng, Y. Wen, D. Ding, Z.-M. Zhang, H.-W. Wei and J. Guo, ACS Chem. Biol., 2023, 18, 915–923 CrossRef PubMed.
Footnote |
† Equal contribution. |
|
This journal is © The Royal Society of Chemistry 2025 |
Click here to see how this site uses Cookies. View our privacy policy here.