DOI:
10.1039/D5SC00083A
(Perspective)
Chem. Sci., 2025,
16, 6573-6582
Biocatalytic recycling of plastics: facts and fiction
Received
6th January 2025
, Accepted 24th March 2025
First published on 31st March 2025
Abstract
Due to the lack of efficient end-of-life management, the mass production of plastics has resulted in serious environmental problems. Sustainable biological approaches using enzymes to degrade and recycle plastic waste are emerging as a complement to conventional methods to promote a circular economy of plastics. Only a fraction of the plastic waste generated is currently suitable for biocatalytic deconstruction and the development of economically and environmentally competitive processes is still pending. Inconsistent claims about new plastic-degrading enzymes reveal a need for robust and standardized analysis methods to ensure reproducible results and a realistic evaluation of their potential. This paper critically reviews enzymatic synthetic polymer degradation and its recycling challenges.
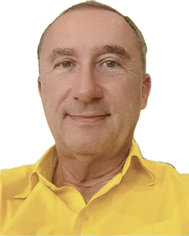 Wolfgang Zimmermann | Prof. Dr Wolfgang Zimmermann is an Emeritus Professor of Microbiology and Bioprocess Technology at the University of Leipzig. He received his PhD from the University of Heidelberg. Following post-doctoral studies at the University of Manchester and ETH Zürich, he held positions as a Professor of Biotechnology at Aalborg University and a Professor of Bioprocess Technology at Chemnitz University of Technology. His research interests include the application of biocatalysis to modify and deconstruct synthetic polymers and the development of enzymatic plastic recycling processes. |
Introduction
Since the 1950s, billions of tons of plastics made from a plethora of polymers have been produced from fossil feedstocks powered by the quest of the petrochemical industries to find proliferating markets alongside growing consumer demands for mass-produced versatile and affordable commodities. The level of global plastics pollution resulting from their overabundance is increasingly perturbing essential Earth system processes.1 The massive greenhouse gas emissions from the production of plastics are contributing to climate change and have been estimated to reach without intervention 15% of the global carbon budget by 2050.2 The vast majority of the waste generated from plastic products ends up in landfills, is incinerated or is released into the environment, and only a fraction is recycled for further use.3 For many years, this systematic mismanagement of plastic waste has not posed a major concern for plastic producers, governments, or even the consumers. However, the ever-growing pollution of land, rivers, lakes, and oceans with plastics and the ensuing formation of micro- and nano-plastics has increased awareness of a major threat to our ecosystems and to human health.4,5
Efforts driven by more stringent legislative mandates and consumer demands to support a circular economy of plastics are aiming to substantially increase the recycling rates of plastic wastes and to extend the lifetime of plastic products. In this context, biocatalytic approaches for plastic recycling have developed into a burgeoning area of research.6–9 The biocatalytic recycling of polyesters has reached industrial scale with the potential to become a relevant complementary technology for plastic waste management.
Microorganisms and biocatalysts reported to be able to degrade plastics have received considerable attention in the scientific community and media, with news outlets portraying “plastic-eating” microbes and enzymes as a solution for the global plastic crisis. A closer look at some of the reports on plastic-degrading enzymes suggests that claims of new results and achievements should not be overestimated to prevent public and peer wrong expectations and misconceptions about enzymatic plastic degradation and recycling.
Plastic waste recycling
The global annual volume of plastic waste generated has been estimated to reach without intervention a staggering 700 million metric tons in 2050 (ref. 10) (Fig. 1). Plastic waste often contains several types of synthetic polymers in complex mixtures, additives and contaminants complicating their potential recycling.11,12 Single-use plastics, for example bags, bottles, and food packaging to be used once and then discarded represent a large share on the total plastic waste produced. Polyolefins (polyethylene, PE, and polypropylene, PP) and polyvinyl chloride (PVC) are the main types of plastic waste and represented almost 58% of the global plastics production of 400 million metric tons in 2022 while polyesters such as polyethylene terephthalate (PET) and polyurethanes (PU) contributed about 6.2% and 5.2%, respectively.13
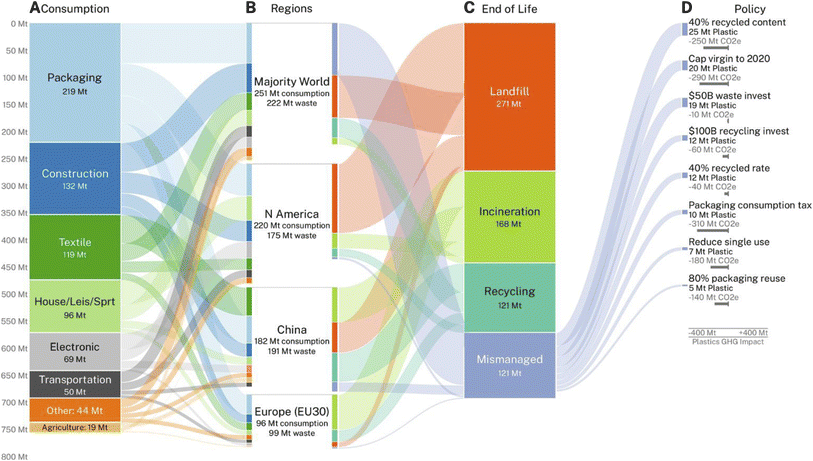 |
| Fig. 1 Global plastics projections. Overall mass of plastics (million metric tons) predicted in 2050 to (A) be consumed in eight global sectors, (B) in four world regions and (C) in four end-of-life fates. (D) Estimated impact of policy interventions on reducing mass of mismanaged plastic waste and greenhouse gas emissions (million metric tons CO2e) in 2050 (reproduced with permission from ref. 10). | |
To mitigate the impact of accumulating global plastic waste, the development of advanced recycling technologies is one of the key strategies to increase the reuse of plastics and to diminish the demand for petrochemical feedstocks.14 Plastic waste recycling is mainly performed by thermo-mechanical or chemical methods.15,16 For the thermo-mechanical recycling of polyesters, for example post-consumer PET bottles, the sorted plastic waste is melted and extruded using high temperatures. The secondary materials produced from the recycled PET are typically of reduced quality and economic value. While the majority of plastic recycling is performed with this technology, the final disposal of the plastic waste is only postponed and hardly affecting virgin plastic production volumes from petrochemicals.17,18 By chemical recycling of PET, for example by glycolysis or methanolysis methods, the polymer is converted to its building blocks which can be used for the re-synthesis of virgin-grade plastics enabling a closed-loop recycling.19,20 Significant innovation and expanded PET recycling capacities using chemical methods can be expected in the future. For example, a PET recycling plant using a low-temperature glycolysis process with a small environmental impact and a production capacity of 280 metric tons recycled PET per day is planned to be in operation in India in 2025.21
Enzymatic recycling of different plastic waste streams
Plastics exhibit a vast diversity of chemical structures with different susceptibility to biological degradation. The recalcitrant properties of PE, PP and PVC due to the lack of functional groups in their homochain polymer backbone requiring carbon–carbon bond cleavage and dehalogenation reactions provide these hydrophobic synthetic polymers with a high resistance to enzymatic degradation.22 In contrast, heterochain polyesters such as PET and PU contain hydrolysable bonds enabling an enzymatic deconstruction. A growing number of enzymes with activity against PET has been described, some of them suitable for applications in industrially relevant polyester recycling processes.7,9,23 Similar to chemical recycling, PET materials are deconstructed in a biocatalytic process to produce the constituent monomers which are recovered and used for the manufacture of new polyester comparable to PET made from petrochemicals.24–27 Different from chemical recycling methods, the biocatalytic process is performed with high selectivity under mild reaction conditions avoiding high temperatures and pressure. For example, the chemical depolymerization of PET by glycolysis, a transesterification reaction between PET and ethylene glycol, may require reaction temperatures of 160–300 °C and ≥1.1 MPa pressure.14
Post-consumer PET has been the main plastic waste targeted for biocatalytic recycling.6,28 The recycling of post-consumer PET bottles and fibres has been scaled up and a processing plant using engineered polyester hydrolases with a capacity of 50.000 metric tons per year is scheduled to be commissioned in France in 2026.29
The predominant types of plastic waste are not efficiently deconstructed by enzymes
With 105 and 76 million metric tons produced globally in 2022, respectively, the polyolefins PE and PP significantly contribute to global plastic waste generation.13 PE is widely used for packaging, construction, automotive and industrial applications. Flexible, low-density PE is found in insulations, plastic bags, and food packaging while rigid high-density PE is used inter alia in detergent bottles and water pipes. PP has a wide range of applications in plastic products with high strength and stiffness and displays an even higher chemical resistance and biological recalcitrance compared to PE. Although a large number of publications have reported biodegrading effects of microorganisms on polyolefins, only limited information is available on potential enzymes involved and their mode of action.30–32 Most studies estimated degradation effects based on weight losses, polymer surface modifications or Fourier-transform infrared (FTIR) spectroscopic analysis while few reports used methods such as carbon isotope labelling33 to unequivocally prove and quantify a microbial mineralization by microorganisms. Polyolefin samples used as substrates for enzymes are often pre-treated by UV-irradiation or oxidizing agents to introduce functional groups and to achieve an initial reduction in the chain length of the polymer to promote their enzymatic degradation.34,35 The degradation effects observed following incubation with enzymes could thereby be limited to the shorter segments of the PE chains created by the abiotic pre-treatment or even originated from the degradation of non-polymeric additives often present in commercial polymer samples.36 Most studies used branched PE of low molecular weight and crystallinity as enzyme substrates which is more susceptible to an enzymatic attack. Typically, slow degradation rates, changes in the molecular mass distribution and modifications of the surface of PE films are observed by treatment with microbial redox enzymes such as multicopper oxidases including laccases and peroxidases for the introduction of functional groups and polymer chain cleavages.37 However, conclusive evidence for significant degradation of unadulterated high-molecular-weight polymer samples by these enzymes, and their biochemical mechanism, is lacking.38 The degradation of PE to low molecular weight products for recycling following the initial introduction of functional groups and chain cleavages will require additional enzymes such as alcohol dehydrogenases, monooxygenases, and hydrolases which complicates the enzymatic deconstruction process.37 A combination of chemical and enzymatic methods might provide a more viable strategy for biological polyolefin recycling. For example, a chemically pre-treated low-molecular weight PE was partially degraded to medium-sized carboxylic acids after incubation with an enzyme cascade composed of a catalase-peroxidase, an alcohol dehydrogenase, a Baeyer–Villiger monooxygenase, and a lipase.39
The homochain polymer PVC with a C–C backbone is resistant to many chemicals and highly recalcitrant to biological degradation. In 2022, it was the third-most widely produced plastic with about 51 million metric tons (ref. 13) and is applied in construction, automotive, and consumer goods. While certain degradation effects of mostly pre-treated PVC materials by various fungi and bacteria have been observed,40–43 convincing evidence for a substantial enzymatic degradation of PVC has not been demonstrated yet. PVC contains a high proportion of plasticizers potentially susceptible to microbial degradation which could result in an overestimation of an observed polymer degradation. A degradation of a PVC sample by a bacterial catalase-peroxidase indicated by FTIR spectroscopy and size exclusion chromatography (SEC) has been reported.44 In a follow-up study, the detected changes in molecular mass distribution following the incubation of PVC with the enzyme could not be confirmed. A surface oxidation of the polymer was also not validated by FTIR spectroscopy suggesting that the FTIR spectroscopic analysis of the PVC sample by Zhang et al.44 was influenced by protein adsorption on the polymer surface.45
None of the previously reported enzymes show sufficient activities against polyolefins and PVC which could make them suitable for applications in plastic recycling processes on any larger scale.
Hydrolases degrading synthetic polyesters are promiscuous enzymes unlikely to have evolved since the 1950s
Polyester hydrolases have been detected in numerous fungi and bacteria, prevalently Actinomycetota from soil and compost habitats containing decaying plant materials where they play a crucial role in the recycling of plant biomass.46 Among a wide range of plant polymer-degrading enzymes, many thermotolerant and thermophilic Actinomycetota produce ester-hydrolysing cutinases degrading the plant polyesters cutin and suberin.47 Actinomycetota have become an import source of thermostable synthetic polyester-hydrolysing enzymes and their cutinases have been served as scaffolds for the construction of engineered variants suitable for biocatalytic recycling processes.7,9,48 The ability of cutinases to degrade structurally similar synthetic polyesters such as PET is likely the result of the low substrate specificity of these naturally occurring hydrolases enabling the hydrolysis of a broad spectrum of aliphatic and aromatic polyesters.49,50
A mesophilic polyester hydrolase-producing bacterium capable of assimilating PET was isolated from environmental samples containing plastic waste.51 Following this report, it has been speculated that the ability of microorganisms and their enzymes to degrade synthetic plastics is a result of their exposure to plastics in the environment.29,52–54 However, a mineralisation of PET by other polyester hydrolase-producing bacteria, for example Actinomycetota, has not been demonstrated using robust methodology such as stable carbon isotope labelling. Furthermore, microorganisms with PET-hydrolysing enzymes have been detected universally in many natural environments and metagenomes including pristine regions devoid of plastic contaminations.55–57 It is therefore debatable whether polyester hydrolases have evolved to degrade synthetic plastics since the introduction of plastic waste into the environment in the 1950s.
High-crystallinity PET is not deconstructed by polyester hydrolases
The thermoplastic polyester PET is a heterochain polymer composed of terephthalic acid and ethylene glycol. PET has numerous applications in the manufacture of bottles and food packaging as well as textile fibers and is worldwide the most recycled type of post-consumer plastics. The polymer is composed of amorphous and crystalline regions with distinct differences in susceptibility to enzymatic degradation. Amorphous and low-crystallinity PET waste, for example thermoform food packaging, is readily deconstructed by thermostable polyester hydrolases and their engineered variants.24,26,58 Important other PET waste streams such as beverage bottles and textile fibres composed of high-crystallinity PET are not directly hydrolysable by enzymes and require a high-temperature pre-treatment step to convert the crystalline PET to amorphous material amenable to enzymatic hydrolysis.26,59–61 The detection of hydrolysis products obtained by treatment of high-crystallinity PET samples with polyester hydrolases62–66 is not a definitive proof of their ability to degrade crystalline PET since the products could originate from the hydrolysis of non-crystalline parts of the sample.7
Large volumes of textile waste containing polyester fibres are generated which are mostly landfilled or incinerated causing growing environmental issues.67,68 The biocatalytic recycling of PET in post-consumer textiles has gained interest due to the tolerance of the enzymatic process to additives, colorants and other types of fibres in mixed textiles. However, like PET beverage bottles, this process necessitates a thermomechanical pre-treatment with a high energy demand for the amorphization of the high-crystallinity PET fibres.69–71
The recalcitrant properties of high-crystallinity PET make significant direct deconstruction in a recycling process impossible with previously reported enzymes.
Highly active and stable polyester hydrolases are required in industrial biocatalytic PET recycling processes
The enzymatic hydrolysis of the amorphous parts of the semi-crystalline polyester PET is most effective at reaction temperatures ∼70 °C near its glass transition temperature (Tg).72,73 This temperature offers a trade-off between polymer mobility required for enzyme accessibility and polymer aging occurring at higher temperatures decreasing enzyme efficiency.60 Mesophilic polyester hydrolases are able to partially hydrolyse amorphous PET at temperatures around 30 °C. Due to their low stability, they do not catalyse the degradation of the bulk polymer at reaction temperatures required for a substantial deconstruction of the polymer.28 The complete enzymatic hydrolysis of amorphous PET in a large-scale industrial process with high substrate loadings within short reaction times at ∼70 °C is requiring highly active biocatalysts with thermostable properties. Only a limited number of polyester hydrolases and their variants have been identified to perform adequately under these conditions.23
Dual enzyme systems composed of a polyester hydrolase and a carboxylesterase to facilitate the degradation of intermediate PET hydrolysis products74–78 are unlikely to be required for large-scale PET deconstruction, which can be efficiently achieved with single, highly active polyester hydrolases.23
Potential for the enzymatic recycling of other types of polyester plastic waste
Thermoplastic or thermoset PU containing polyester, polyether, and urethane bonds are versatile materials with a wide range of applications in insulations, coatings, footwear, automotive and construction. PU is another major contributor to global plastic waste accumulation, despite recycling opportunities.79 Considering their prospects for a biocatalytic recycling of PU, polyester hydrolases are able to partially hydrolyse ester bonds in some types of PU materials. For a conversion of the polymer to low molecular weight compounds, further enzymes such as urethanases and amidases to cleave the different types of bonds in PU would be required in an efficient biocatalytic degradation process.80 The inherent structural diversity of PU waste may however necessitate a combination of chemical pretreatments and biocatalytic depolymerization to obtain high-value reusable compounds in innovative PU recycling strategies.79
Plastic wastes from polyesters such as poly(ethylene furanoate) (PEF) as a biobased alternative to PET, the biodegradable thermoplastic polyester polylactic acid (PLA), polybutylene adipate terephthalate (PBAT), and polybutylene succinate (PBS) which are efficiently degraded by polyester hydrolases could also provide feedstocks for future biocatalytic recycling processes.7,81,82 Due to the small market shares, their recovery has currently not a large impact on total plastic recycling rates.
Biocatalytic PET recycling needs to become competitive
A process-based life cycle analysis indicated that an enzymatic recycling process using high-crystallinity post-consumer PET waste such as beverage bottles has a higher environmental impact compared to PET from petrochemical sources83 (Fig. 2). The thermomechanical melt extrusion treatment to convert high-crystallinity PET to amorphous materials suitable for enzymatic hydrolysis was identified as a major factor contributing to the high energy consumption and greenhouse gas emissions of the modelled process.70 A transformation to renewable energy sources for electricity generation and the development of low-energy pre-treatment methods could reduce greenhouse gas emissions and costs of enzymatic high-crystallinity PET recycling. Post-consumer clear PET beverage bottles for which cost-efficient mechanical recycling methods with lower environmental impact already exist may represent a less suitable waste stream for biocatalytic recycling.84
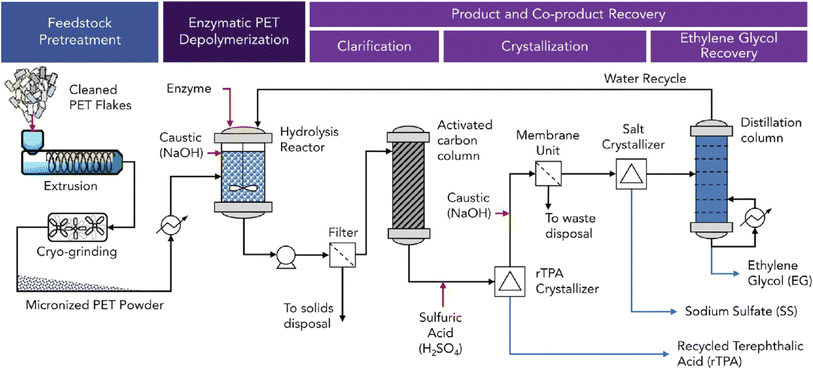 |
| Fig. 2 Schematic PET recycling process with polyester hydrolases. An amorphization and micronization pre-treatment is shown for the enzymatic recycling of high-crystallinity PET feedstock. The enzymatic hydrolysis is performed in a stirred-tank reactor with pH control using NaOH to neutralize released terephthalic acid (rTPA). The recovery of the hydrolysis products rTPA by crystallisation and of ethylene glycol (EG) by distillation in the downstream process is depicted. Red arrows indicate raw material inputs, and blue arrows product and co-product streams (reproduced with permission from ref. 92). | |
A minimum selling price of about USD 2 per kg for terephthalic acid produced with an enzymatic recycling process using high-crystallinity PET feedstock based on a post-consumer bale price of about USD 0.7 per kg has been estimated.83 Considering the continuing low market price for terephthalic acid and PET from petrochemical sources (USD 0.66 to USD 1.01 per kg for terephthalic acid and USD 0.67 to USD 1.52 per kg for PET in March 2025 (ref. 85 and 86)), it is evident that enzymatic recycling processes must significantly reduce costs to compete effectively with established market prices.
The price of post-consumer PET feedstock is strongly affecting the operating costs of a biocatalytic recycling plant.87 PET waste streams containing multilayer plastics or low-crystallinity thermoform PET packaging which do not require a high-energy pre-treatment for enzymatic deconstruction are typically mostly landfilled or incinerated. The bale prices for these post-consumer plastics are significantly lower compared to clear post-consumer PET bales from beverage bottles.20 These plastic waste streams have been shown suitable for enzymatic recycling and could be considered as alternative feedstocks for biocatalytic processes integrating into existing recycling schemes.6,9 However, inadequate plastic waste collection and inefficient separation presently significantly limit the recycling of these feedstocks in many countries. The extent of their utilisation will depend on the establishment of suitable waste collection infrastructure and the development of advanced sorting technologies.88,89
Standardized plastic samples and appropriate analytical methods are important for accurate assessment of plastic-degrading enzyme activities
Results on enzymatic plastic-degrading activities obtained from different studies are often difficult to compare. In many reports, non-standardised and poorly characterised plastic samples were used as enzyme substrates, lacking essential details about their exact formulation, molecular mass, and crystallinity. To add to the complexity of the composition of plastic substrates used for enzymatic degradation studies, the presence of additives such as stabilizers, fillers and plasticizers common in commercial samples further complicates an interpretation and comparison of the results.90
The use of gravimetric analyses to demonstrate weight losses of the treated polymer materials can lead to an overestimation of enzymatic activity in the presence of easily degradable non-polymer compounds in the plastic sample.91 FTIR spectroscopy is often used to detect a biological oxidation of homochain polymer samples evidenced by the formation of C
O, C–O and O–H bonds. However, analyses based on FTIR can lead to ambiguous results due to misinterpretations of the spectra, for example in the presence of additives or contaminants in the sample.92,93 The oxidation and depolymerisation of PE powder by hexamerin proteins with phenoloxidase activity in the saliva of Galleria mellonella larvae has been claimed based on analysis by FTIR spectroscopy and SEC.94 In a later study, an enzymatic oxidation and degradation of additive-free PE films could not be confirmed by FTIR spectroscopic analysis and SEC indicating that the peaks in the FTIR spectra observed by Sanluis-Verdes et al.94 likely originated from protein bound to the film and not from an enzymatic modification of the PE sample.45
Recycling alone will not solve the plastic crisis
Projections on global plastics based on current trends for production, consumption, and end-of-life management suggest that by 2050, without significant intervention, less than 20% of the total amount of globally produced plastic waste will be recycled, while about 120 million metric tons will contribute to mismanaged plastic pollution10 (Fig. 1). While supporting the transition to a circular economy of plastics, recycling alone is insufficient to address the plastic pollution crisis.
An essential step forward will be globally implemented mandates such as proposed in the Environment Programme of the United Nations to limit plastic production from petrochemical feedstocks.95 This demand is however continuously met with stiff opposition from a number of oil-producing countries. A model to forecast the impact of different policy interventions indicated that a 40% minimum recycled plastic content mandate and a cap to global virgin plastic production at 2020 levels are among the most effective measures to substantially reduce mismanaged plastic waste.10 The development of novel types of plastics designed for circularity represents another important strategy for reducing the accumulation of recalcitrant plastic waste.96 Implementing robust extended producer responsibility schemes and a policy-driven phasing out of single-use products supported by fostering public awareness to change consumer behavior constitute additional critical measures to significantly reduce global plastic pollution.97,98
Conclusions
The potential of biocatalysis for synthetic polymer deconstruction and its application in plastic waste recycling processes has garnered high attention. While plastic degradation using enzymes offers an appealing green alternative to conventional plastic recycling methods, their potential to deconstruct important types of waste plastics is presently limited. A large-scale enzymatic recycling of polyolefins or PVC waste is currently not feasible. Reports on enzymes degrading these major plastic waste polymers must use rigorous analytical methods and thorough biochemical characterisation of the enzymes to obtain reliable data on their catalytic potential.
Biocatalytic PET deconstruction employing advanced polyester hydrolases with high performance and stability has emerged as an early-stage plastic recycling technology. The process costs and environmental impact of enzymatic recycling must be addressed to compete with advanced chemical and mechanical recycling methods. The implementation of decarbonized energy in the recycling process and the improvement of pre-treatment methods for important plastic waste streams like PET bottles and fibres will significantly enhance the cost-efficiency and environmental performance of biocatalytic recycling. The demand for recycled PET is expected to increase in response to regulatory recycled content targets creating opportunities for biocatalytic methods to process currently unutilised plastic waste streams. Increased plastic recycling capacity and binding global policies to limit petroleum-based plastic production will be essential for a circular economy of plastics and solving the global plastic crisis.
Data availability
No primary research results, software or code have been included and no new data were generated or analysed as part of this review.
Author contributions
WZ conceived and wrote the manuscript.
Conflicts of interest
The author has filed the European patent application EP4455200A2 on enzyme-based degradation of plastics.
References
- P. Villarrubia-Gomez, B. C. Almroth, M. Eriksen, M. Ryberg and S. E. Cornell, Plastics pollution exacerbates the impacts of all planetary boundaries, One Earth, 2024, 7, 2119–2138 Search PubMed.
- J. Zheng and S. Suh, Strategies to reduce the global carbon footprint of plastics, Nat. Clim. Change, 2019, 9, 374–378 Search PubMed.
-
R. Geyer, Production, use, and fate of synthetic polymers, in Plastic Waste and Recycling, ed. T. M. Letcher, Academic Press, 2020, vol. 2, pp. 13–32 Search PubMed.
- M. MacLeod, H. P. H. Arp, M. B. Tekman and A. Jahnke, The global threat from plastic pollution, Science, 2021, 373, 61–65 CAS.
- N. Chartres, C. B. Cooper, G. Bland, K. E. Pelch, S. A. Gandhi, A. BakenRa and T. J. Woodruff, Effects of microplastic exposure on human digestive, reproductive, and respiratory health: a rapid systematic review, Environ. Sci. Technol., 2024, 58, 22843–22864 CAS.
- W. Zimmermann, Biocatalytic recycling of polyethylene terephthalate plastic, Phil. Trans. R. Soc. A., 2020, 378, 20190273 CrossRef CAS.
- V. Tournier, S. Duquesne, F. Guillamot, H. Cramail, D. Taton, A. Marty and I. André, Enzymes' power for plastics degradation, Chem. Rev., 2023, 123, 5612–5701 CrossRef CAS PubMed.
- F. Kawai, R. Iizuka and T. Kawabata, Engineered polyethylene terephthalate hydrolases: perspectives and limits, Appl. Microbiol. Biotechnol., 2024, 108, 404 CrossRef CAS.
- W. Zimmermann, Polyester-degrading enzymes in the circular economy of plastics, Nat. Rev. Bioeng. Search PubMed , in press..
- A. S. Pottinger, R. Geyer, N. Biyani, C. C. Martinez, N. Nathan, M. R. Morse, C. Liu, S. Hu, M. de Bruyn, C. Boettiger, E. Baker and D. J. McCauley, Pathways to reduce global plastic waste mismanagement and greenhouse gas emissions by 2050, Science, 2024, 386, 1168–1173 CrossRef CAS PubMed.
- M. Roosen, N. Mys, M. Kusenberg, P. Billen, A. Dumoulin, J. Dewulf, K. M. Van Geem, K. Ragaert and S. De Meester, Detailed analysis of the composition of selected plastic packaging waste products and Its implications for mechanical and thermochemical recycling, Environ. Sci. Technol., 2020, 54, 13282–13293 CAS.
- S. Stevens, M. McPartland, Z. Bartosova, H. S. Skåland, J. Völker and M. Wagner, Plastic food packaging from five countries contains endocrine- and metabolism-disrupting chemicals, Environ. Sci. Technol., 2024, 58, 4859–4871 CAS.
-
Plastics Europe, https://plasticseurope.org/knowledge-hub/plastics-the-fast-facts-2023, accessed March 2025.
- R. A. Clark and M. P. Shaver, Depolymerization within a circular plastics system, Chem. Rev., 2024, 124, 2617–2650 CAS.
- I. Vollmer, M. J. F. Jenks, M. C. P. Roelands, R. J. White, T. Harmelen, P. Wild, G. P. Laan, F. Meirer, J. T. F. Keurentjes and B. M. Weckhuysen, Beyond mechanical recycling: giving new life to plastic waste, Angew. Chem., Int. Ed., 2020, 59, 15402–15423 CAS.
- D. Jubinville, E. Esmizadeh, S. Saikrishnan, C. Tzoganakis and T. Mekonnen, A comprehensive review of global production and recycling methods of polyolefin (PO) based products and their post-recycling applications, Sustainable Mater. Technol., 2020, 25, e00188 CrossRef CAS.
- T. Zink and R. Geyer, Recycling and the myth of landfill diversion, J. Ind. Ecol., 2018, 23, 541–548 CrossRef.
- Z. O. Schyns and M. P. Shaver, Mechanical recycling of packaging plastics: a review, Macromol. Rapid Commun., 2021, 42, 2000415 CrossRef CAS.
- H. Li, H. A. Aguirre-Villegas, R. D. Allen, X. Bai, C. H. Benson, G. T. Beckham, S. L. Bradshaw, J. L. Brown, R. C. Brown, V. S. Cecon, J. B. Curley, G. W. Curtzwiler, S. Dong, S. Gaddameedi, J. E. García, I. Hermans, M. S. Kim, J. Ma, L. O. M. Mavrikakis, O. O. Olafasakin, T. A. Osswald, K. G. Papanikolaou, H. Radhakrishnan, M. O. Sanchez Castillo, K. L. Sánchez-Rivera, K. N. Tumu, R. C. Van Lehn, K. L. Vorst, M. M. Wright, J. Wu, V. M. Zavala, P. Zhoua and G. W. Huber, Expanding plastics recycling technologies: chemical aspects, technology status and challenges, Green Chem., 2022, 24, 8899 CAS.
- A. McNeeley and Y. A. Liu, Assessment of PET depolymerization processes for circular economy. 1. Thermodynamics, chemistry, purification, and process design, Ind. Eng. Chem. Res., 2024, 63, 3355–3399 CAS.
-
Revalyu Resources GmbH, https://www.revalyu.com/news/revalyu-resources-successfully-commissions-second-plant-of-worlds-largest-chemical-pet-plastic-recycling-site, accessed January 2025.
- R. Wei and W. Zimmermann, Microbial enzymes for the recycling of recalcitrant petroleum-based plastics: how far are we?, Microb. Biotechnol., 2017, 10, 1308–1322 CAS.
- G. Arnal,
et al., Assessment of four engineered PET degrading enzymes considering large-scale industrial applications, ACS Catal., 2023, 13, 13156–13166 CAS.
- C. Sonnendecker, J. Oeser, P. K. Richter, P. Hille, Z. Zhao, C. Fischer, H. Lippold, P. Blázquez-Sánchez, F. Engelberger, C. A. Ramírez-Sarmiento, T. Oeser, Y. Lihanova, R. Frank, H.-G. Jahnke, S. Billig, B. Abel, N. Sträter, J. Matysik and W. Zimmermann, Low carbon footprint recycling of post-consumer PET plastic with a metagenomic polyester hydrolase, ChemSusChem, 2022, 15, e202101062 CAS.
- V. Tournier, C. M. Topham, A. Gilles, B. David, C. Folgoas, E. Moya-Leclair, E. Kamionka, M.-L. Desrousseaux, H. Texier, S. Gavalda, M. Cot, E. Guémard, M. Dalibey, J. Nomme, G. Cioci, S. Barbe, M. Chateau, I. André, S. Duquesne and A. Marty, An engineered PET depolymerase to break down and recycle plastic bottles, Nature, 2020, 580, 216–219 CAS.
- H. Lu, D. J. Diaz, N. J. Czarnecki, C. Zhu, W. Kim, R. Shroff, D. J. Acosta, B. R. Alexander, H. O. Cole, Y. Zhang, N. A. Lynd, A. D. Ellington and H. S. Alper, Machine learning-aided engineering of hydrolases for PET depolymerization, Nature, 2022, 604, 662–667 CAS.
- Y. Cheng, Y. Cheng, S. Zhou, Y. Ruzha and Y. Yang, Closed-loop recycling of PET fabric and bottle waste by tandem pre-amorphization and enzymatic hydrolysis, Resour. Conserv. Recycl., 2024, 208, 107706 CAS.
- F. Kawai, T. Kawabata and M. Oda, Current state and perspectives related to the polyethylene terephthalate hydrolases available for biorecycling, ACS Sustain. Chem. Eng., 2020, 8, 8894–8908 CAS.
- B. Johnson, Plastic-eating bacteria boost growing business of bioremediation, Nat. Biotechnol., 2024, 42, 1481–1485 CrossRef CAS PubMed.
-
W. Zimmermann, Degradation of plastics by fungi, in Encyclopedia of Mycology, ed. O. Zaragoza, Elsevier, 2021, vol. 2, pp. 650–661 Search PubMed.
- N. Zhang, M. Ding and Y. Yuan, Current advances in biodegradation of polyolefins, Microorganisms, 2022, 10, 1537 CrossRef CAS.
- V. Gambarini, O. Pantos, J. M. Kingsbury, L. Weaver, K. M. Handley and G. Lear, PlasticDB: a database of microorganisms and proteins linked to plastic biodegradation, Database, 2022, 2022, 1–12 CrossRef.
- M. Goudriaan, V. Hernando Morales, M. T. J. van der Meer, A. Mets, R. T. Ndhlovu, J. van Heerwaarden, S. Simon, V. B. Heuer, K.-U. Hinrichs and H. Niemann, A stable isotope assay with 13C-labeled polyethylene to investigate plastic mineralization mediated by Rhodococcus ruber, Mar. Pollut. Bull., 2023, 186, 114369 CAS.
- A. C. Albertsson, S. O. Andersson and S. Karlsson, The mechanism of biodegradation of polyethylene, Polym. Degrad. Stab., 1987, 18, 73–87 CAS.
- H. Inderthal, S. L. Tai and S. T. L. Harrison, Non-hydrolyzable plastics – an interdisciplinary look at plastic bio-oxidation, Trends Biotechnol., 2020, 39, 12–23 Search PubMed.
- A. A. Cuthbertson, C. Lincoln, J. Miscall, L. M. Stanley, A. K. Maury, A. S. Asundi, C. J. Tassone, N. A. Rorrer and G. T. Beckham, Characterization of polymer properties and identification of additives in commercially available research plastics, Green Chem., 2024, 26, 7067–7090 CAS.
- J. Jin, J. Arciszewski, K. Auclair and Z. Jia, Enzymatic polyethylene biorecycling: confronting challenges and shaping the future, J. Hazard. Mater., 2023, 460, 132449 CAS.
- G. Lear, S. D. M. Maday, V. Gambarini, G. Northcott, R. Abbel, J. M. Kingsbury, L. Weaver, J. A. Wallbank and O. Pantos, Microbial abilities to degrade global environmental plastic polymer waste are overstated, Environ. Res. Lett., 2022, 17, 043002 Search PubMed.
- T. Oiffer, F. Leipold, P. Süss, D. Breite, J. Griebel, M. Khurram, Y. Branson, E. de Vries, A. Schulze, C. A. Helm, R. Wei and U. T. Bornscheuer, Chemo-enzymatic depolymerization of functionalized low-molecular-weight polyethylene, Angew. Chem., Int. Ed., 2024, 63, e202415012 CAS.
- J. S. Webb, M. Nixon, I. M. Eastwood, M. Greenhalgh, G. D. Robson and P. S. Handley, Fungal colonization and biodeterioration of plasticized polyvinyl chloride, Appl. Environ. Microbiol., 2000, 66, 3194–3200 CAS.
- L. Giacomucci, N. Raddadi, M. Soccio, N. Lotti and F. Fava, Biodegradation polyvinyl chloride plastic films by enriched anaerobic marine consortia, Mar. Environ. Res., 2020, 158, 104949 CAS.
- Č. Novotný, J. Fojtík, M. Mucha and K. Malachová, Biodeterioration of compost-pretreated polyvinyl chloride films by microorganisms isolated from weathered plastics, Front. Bioeng. Biotechnol., 2022, 10, 832413 CrossRef PubMed.
- I. Nyamjav, Y. Jang, Y. E. Lee and S. Lee, Biodegradation of polyvinyl chloride by Citrobacter koseri isolated from superworms (Zophobas atratus larvae), Front. Microbiol., 2023, 14, 1175249 CrossRef.
- Z. Zhang, H. Peng, Y. Dongchen, G. Zhang, J. Zhang and J. Feng, Polyvinyl chloride degradation by a bacterium isolated from the gut of insect larvae, Nat. Commun., 2022, 13, 5360 CAS.
- A. A. Stepnov, E. Lopez-Tavera, R. Klauer, C. L. Lincoln, R. R. Chowreddy, G. T. Beckham, V. G. H. Eijsink, K. Solomon, M. Blenner and G. Vaaje-Kolstad, Revisiting the activity of two poly(vinyl chloride)- and polyethylene-degrading enzymes, Nat. Commun., 2024, 15, 8501 CAS.
- M. Goodfellow and S. T. Williams, Ecology of actinomycetes, Rev. Microbiol., 1983, 37, 189–216 CAS.
-
P. E. Kolattukudy, Polyesters in higher plants, Adv. Biochem. Engin. Biotechnol., 2002, vol. 71, pp. 1–49 Search PubMed.
-
R. Wei, T. Oeser and W. Zimmermann, Synthetic polyester-hydrolyzing enzymes from thermophilic Actinomycetes, in Adv. Appl. Microbiol., ed. S. Sariaslani and G. M. Gadd, Academic Press, 2014, vol. 89, pp. 267–305 Search PubMed.
- O. Khersonsky and D. S. Tawfik, Enzyme promiscuity: a mechanistic and evolutionary perspective, Annu. Rev. Biochem., 2010, 79, 471–505 CAS.
- M. Martínez-Martínez, C. Coscolín, G. Santiago, J. Chow, P. J. Stogios, R. Bargiela, C. Gertler, J. Navarro-Fernández, A. Bollinger, S. Thies, C. Méndez-García, A. Popovic, G. Brown, T. N. Chernikova, A. García-Moyano, G. E. K. Bjerga, P. Pérez-García, T. Hai, M. V. Del Pozo, R. Stokke, I. H. Steen, H. Cui, X. Xu, B. P. Nocek, M. Alcaide, M. Distaso, V. Mesa, A. I. Peláez, J. Sánchez, P. C. F. Buchholz, J. Pleiss, A. Fernández-Guerra, F. O. Glöckner, O. V. Golyshina, M. M. Yakimov, A. Savchenko, K.-E. Jaeger, A. F. Yakunin, W. R. Streit, P. N. Golyshin, V. Guallar and M. Ferrer, Determinants and prediction of esterase substrate promiscuity patterns, ACS Chem. Biol., 2018, 13, 225–234 Search PubMed.
- S. Yoshida, K. Hiraga, T. Takehana, I. Taniguchi, H. Yamaji, Y. Maeda, K. Toyohara, K. Miyamoto, Y. Kimura and K. Oda, A bacterium that degrades and assimilates poly(ethylene terephthalate), Science, 2016, 351, 1196–1199 CrossRef CAS PubMed.
- U. T. Bornscheuer, Feeding on plastic. A bacterium completely degrades poly(ethylene terephthalate), Science, 2016, 351, 1154–1155 CrossRef CAS PubMed.
- E. J. Okal, G. Heng, E. A. Magige, S. Khan, S. Wu, Z. Ge, T. Zhang, P. E. Mortimer and J. Xu, Insights into the mechanisms involved in the fungal degradation of plastics, Ecotoxicol. Environ. Saf., 2023, 262, 115202 CrossRef CAS.
- S. S. Ibrahim, D. Ionescu and H.-P. Grossart, Tapping into fungal potential: Biodegradation of plastic and rubber by potent fungi, Sci. Total Environ., 2024, 934, 173188 CrossRef CAS PubMed.
- D. Danso, C. Schmeisser, J. Chow, W. Zimmermann, R. Wei, C. Leggewie, X. Li, T. Hazen and W. R. Streit, New insights into the function and global distribution of polyethylene terephthalate (PET)-degrading bacteria and enzymes in marine and terrestrial metagenomes, Appl. Environ. Microbiol., 2018, 84, e0277317 Search PubMed.
- P. C. F. Buchholz, G. Feuerriegel, H. Zhang, P. Perez-Garcia, L.-L. Nover, J. Chow, W. R. Streit and J. Pleiss, Plastics degradation by hydrolytic enzymes: The plastics-active enzymes database—PAZy, Proteins, 2022, 90, 1443–1456 CAS.
- I. Karunatillaka, L. Jaroszewski and A. Godzik, Novel putative polyethylene terephthalate (PET) plastic degrading enzymes from the environmental metagenome, Proteins, 2022, 90, 504–511 CAS.
- L. Shi, P. Liu, Z. Tan, W. Zhao, J. Gao, Q. Gu, H. Ma, H. Liu and L. Zhu, Complete depolymerization of PET wastes by an evolved PET hydrolase from directed evolution, Angew. Chem., Int. Ed., 2023, 135, e202218390 Search PubMed.
- S. W. Schubert, T. B. Thomsen, K. S. Clausen, A. Malmendal, C. J. Hunt, K. Borch, K. Jensen, J. Brask, A. S. Meyer and P. Westh, Relationships of crystallinity and reaction rates for enzymatic degradation of poly (ethylene terephthalate), PET, ChemSusChem, 2024, 17, e202301752 CAS.
- R. Wei, D. Breite, C. Song, D. Gräsing, T. Ploss, P. Hille, R. Schwerdtfeger, J. Matysik, A. Schulze and W. Zimmermann, Biocatalytic degradation efficiency of postconsumer polyethylene terephthalate packaging determined by their polymer microstructures, Adv. Sci., 2019, 6, 1900491 Search PubMed.
- N. E. Wallace, M. C. Adams, A. C. Chafin, D. D. Jones, C. L. Tsui and T. D. Gruber, The highly crystalline PET found in plastic water bottles does not support the growth of the PETase-producing bacterium Ideonella sakaiensis, Environ. Microbiol. Rep., 2020, 12, 578–582 CrossRef CAS.
- H. P. Austin, M. D. Allena, B. S. Donohoe, N. A. Rorrer, F. L. Kearns, R. L. Silveira, B. C. Pollard, G. Dominick, R. Duman, K. El Omari, V. Mykhaylyk, A. Wagner, W. E. Michenerc, A. Amore, M. S. Skaf, M. F. Crowley, A. W. Thorne, C. W. Johnson, L. Woodcock, J. E. McGeehan and G. T. Beckham, Characterization and engineering of a plastic-degrading aromatic polyesterase, Proc. Natl. Acad. Sci. U. S. A., 2018, 115, E4350–E4357 CrossRef CAS.
- D. Gercke, C. Furtmann, I. E. P. Tozakidis and J. Jose, Highly crystalline post-consumer PET waste hydrolysis by surface displayed PETase using a bacterial whole-Cell biocatalyst, ChemCatChem, 2021, 13, 3479–3489 CrossRef CAS.
- E. Erickson, J. E. Gado, L. Avilán, F. Bratti, R. K. Brizendine, P. A. Cox, R. Gill, R. Graham, D.-J. Kim, G. König, W. E. Michener, S. Poudel, K. J. Ramirez, T. J. Shakespeare, M. Zahn, E. S. Boyd, C. M. Payne, J. L. DuBois, A. R. Pickford, G. T. Beckham and J. E. McGeehan, Sourcing thermotolerant poly(ethylene terephthalate) hydrolase scaffolds from natural diversity, Nat. Commun., 2022, 13, 7850 CAS.
- M. E. Sevilla, M. D. Garcia, Y. Perez-Castillo, V. Armijos-Jaramillo, S. Casado, K. Vizuete, A. Debut and L. Cerda-Mejía, Degradation of PET bottles by an engineered Ideonella sakaiensis PETase, Polymers, 2023, 15, 1779 CAS.
- Y. Liu, H. Lin, Z. Wei, S. Bai, S. Chen, J. Wu and Z. Liu, Efficient mild depolymerization of polyester plastics accomplished by engineered PETase via directed evolution of flexible loops, Cell Rep. Phys. Sci., 2024, 5, 102295 CAS.
- S. Bianchi, F. Bartoli, C. Bruni, C. Fernandez-Avila, L. Rodriguez-Turienzo, J. Mellado-Carretero, D. Spinelli and M.-B. Coltelli, Opportunities and limitations in recycling fossil polymers from textiles, Macromol., 2023, 3, 120–148 CAS.
-
Textile exchange, Materials market report 2024, https://textileexchange.org/knowledge-center/reports/materials-market-report-2024, accessed January 2025.
-
A. Marty, M. Chateau and M. Aloui Dalibey, Carbios, Process for degrading plastic products, WO2021/123299A1, 2021.
- T. Uekert, A. Singh, J. S. DesVeaux, T. Ghosh, A. Bhatt, G. Yadav, S. Afzal, J. Walzberg, K. M. Knauer, S. R. Nicholson, G. T. Beckham and A. C. Carpenter, Technical, economic, and environmental comparison of closed-loop recycling technologies for common plastics, ACS Sustain. Chem. Eng., 2023, 11, 965–978 CAS.
- Y. Cheng, Y. Cheng, S. Zhou, Y. Ruzha and Y. Yang, Closed-loop recycling of PET fabric and bottle waste by tandem pre-amorphization and enzymatic hydrolysis, Resour. Conserv. Recycl., 2024, 208, 107706 CAS.
- E. Marten, R.-J. Mueller and W.-D. Deckwer, Studies on the enzymatic hydrolysis of polyesters I. Low molecular mass model esters and aliphatic polyesters, Polym. Degrad. Stab., 2003, 80, 485–501 CAS.
- Å. M. Ronkvist, W. Xie, W. Lu and R. A. Gross, Cutinase-catalyzed hydrolysis of poly(ethylene terephthalate), Macromolecules, 2009, 42, 5128–5138 Search PubMed.
- M. Barth, A. Honak, T. Oeser, R. Wei, M. R. Belisário-Ferrari, J. Then, J. Schmidt and W. Zimmermann, A dual enzyme system composed of a polyester hydrolase and a carboxylesterase enhances the biocatalytic degradation of polyethylene terephthalate films, Biotechnol. J., 2016, 11, 1082–1087 CAS.
- A. Carniel, É. Valoni, J. Nicomedes, A. C. Gomes and A. M. Castro, Lipase from Candida antarctica (CALB) and cutinase from Humicola insolens act synergistically for PET hydrolysis to terephthalic acid, Process Biochem., 2017, 59, 84–90 CrossRef CAS.
- B. C. Knott, E. Erickson, M. D. Allen, J. E. Gado, R. Graham, F. L. Kearns, I. Pardo, E. Topuzlu, J. J. Anderson, H. P. Austin, G. Dominick, C. W. Johnson, N. A. Rorrer, C. J. Szostkiewicz, V. Copiée, C. M. Payne, H. L. Woodcock, B. S. Donohoe, G. T. Beckham and J. E. McGeehan, Characterization and engineering of a two-enzyme system for plastics depolymerization, Proc. Natl. Acad. Sci. U. S. A., 2020, 117, 25476–25485 CrossRef CAS.
- L. Aer, Q. Jiang, L. Zhong, Q. Si, X. Liu, Y. Pan, J. Feng, H. Zeng and L. Tang, Optimization of polyethylene terephthalate biodegradation using a self-assembled multi-enzyme cascade strategy, J. Hazard. Mater., 2024, 476, 134887 CAS.
- D. Lu, J. Wu, S. Jin, Q. Wu, F. Wang, L. Deng and K. Nie, Dual-enzyme-cascade catalysis for PET biodegradation based on a variable-temperature program, Catalysts, 2024, 14, 543 CAS.
- B. Liu, Z. Westman, K. Richardson, D. Lim, A. L. Stottlemyer, T. Farmer, P. Gillis, V. Vlcek, P. Christopher and M. M. Abu-Omar, Opportunities in closed-loop molecular recycling of end-of-life polyurethane, ACS Sustain. Chem. Eng., 2023, 11, 6114–6128 CAS.
- A. Raczyńska, A. Góra and I. André, An overview on polyurethane-degrading enzymes, Biotechnol. Adv., 2024, 70, 108439 Search PubMed.
- A. K. Urbanek, A. M. Mirończuk, A. García-Martín, A. Saborido, I. de la Mata and M. Arroyo, Biochemical properties and biotechnological applications of microbial enzymes involved in the degradation of polyester-type plastics, Biochim. Biophys. Acta – Proteins Proteom., 2020, 1868, 140315 CAS.
- E. de Jong, H. A. Visser, A. S. Dias, C. Harvey and G.-J. M. Gruter, The road to bring FDCA and PEF to the market, Polymers, 2022, 14, 943 CAS.
- T. Uekert, J. S. DesVeaux, A. Singh, S. R. Nicholson, P. Lamers, T. Ghosh, J. E. McGeehan, A. C. Carpenter and G. T. Beckham, Life cycle assessment of enzymatic poly(ethylene terephthalate) recycling, Green Chem., 2022, 24, 6531–6543 CAS.
- U. R. Gracida-Alvarez, H. Xu, P. Thathiana Benavides, M. Wang and T. R. Hawkins, Circular economy sustainability analysis framework for plastics: application for poly(ethylene terephthalate) (PET), ACS Sustainable Chem. Eng., 2023, 11, 514–524 CAS.
-
Business Analytiq, https://businessanalytiq.com/procurementanalytics/index/terephthalic-acid-price-index, accessed March 2025.
-
Business Analytiq, https://businessanalytiq.com/procurementanalytics/index/pet-price-index, accessed March 2025.
- A. Singh, N. A. Rorrer, S. R. Nicholson, E. Erickson, J. S. DesVeaux, A. F. T. Avelino, P. Lamers, A. Bhatt, Y. Zhang, G. Avery, L. Tao, A. R. Pickford, A. C. Carpenter, J. E. McGeehan and G. T. Beckham, Techno-economic, life-cycle, and socioeconomic impact analysis of enzymatic recycling of poly(ethylene terephthalate), Joule, 2021, 5, 2479–2503 CAS.
- C. Lubongo and P. Alexandridis, Assessment of performance and challenges in use of commercial automated sorting technology for plastic waste, Recycling, 2022, 7, 11 Search PubMed.
- R. Pučnik, M. Dokl, Y. Van Fan, A. Vujanović, Z. Novak Pintarič, K. B. Aviso, R. R. Tan, B. Pahor, Z. Kravanja and L. Čuček, A waste separation system based on sensor technology and deep learning: A simple approach applied to a case study of plastic packaging waste, J. Clean. Prod., 2024, 450, 141762 Search PubMed.
- H. Wiesinger, Z. Wang and S. Hellweg, Deep dive into plastic monomers, additives, and processing aids, Environ. Sci. Technol., 2021, 55, 9339–9351 CrossRef CAS PubMed.
- D. Danso, J. Chow and W. R. Streit, Plastics: environmental and biotechnological perspectives on microbial degradation, Appl. Environ. Microbiol., 2019, 85, 1–14 CrossRef.
- C. Weber, S. Pusch and T. Opatz, Polyethylene bio-degradation by caterpillars, Curr. Biol., 2017, 27, R744–R745 CrossRef CAS.
- C. Sandt, J. Waeytens, A. Deniset-Besseau, C. Nielsen-Leroux and A. Réjasse, Use and misuse of FTIR spectroscopy for studying the bio-oxidation of plastics, Spectrochim. Acta, Part A, 2021, 258, 119841 CrossRef CAS PubMed.
- A. Sanluis-Verdes, P. Colomer-Vidal, F. Rodriguez-Ventura, M. Bello-Villarino, M. Spinola-Amilibia, E. Ruiz-Lopez, R. Illanes-Vicioso, P. Castroviejo, R. Aiese Cigliano, M. Montoya, P. Falabella, C. Pesquera, L. Gonzalez-Legarreta, E. Arias-Palomo, M. Solà, T. Torroba, C. F. Arias and F. Bertocchini, Wax worm saliva and the enzymes therein are the key to polyethylene degradation by Galleria mellonella, Nat. Commun., 2022, 13, 5568 CAS.
-
United Nations Environment Programme, https://wedocs.unep.org/bitstream/handle/20.500.11822/39812/OEWG_PP_1_INF_1_UNEA%20resolution.pdf, accessed January 2025.
- S. T. Schwab, M. Baur, T. F. Nelson and S. Mecking, Synthesis and deconstruction of polyethylene-type materials, Chem. Rev., 2024, 124, 2327–2351 CAS.
- J. Zheng and S. Suh, Strategies to reduce the global carbon footprint of plastics, Nat. Clim. Change, 2019, 9, 374–378 Search PubMed.
- W. Cowger, K. A. Willis, S. Bullock, K. Conlon, J. Emmanuel, L. M. Erdle, M. Eriksen, T. A. Farrelly, B. D. Hardesty, K. Kerge, N. Li, Y. Li, A. Liebman, N. Tangri, M. Thiel, P. Villarrubia-Gómez, T. R. Walker and M. Wang, Global producer responsibility for plastic pollution, Sci. Adv., 2024, 10, eadj8275 Search PubMed.
|
This journal is © The Royal Society of Chemistry 2025 |
Click here to see how this site uses Cookies. View our privacy policy here.