DOI:
10.1039/D4RA08798D
(Review Article)
RSC Adv., 2025,
15, 2334-2346
Research on transition metals for the multicomponent synthesis of benzo-fused γ-lactams
Received
16th December 2024
, Accepted 13th January 2025
First published on 23rd January 2025
Abstract
Benzo-fused γ-lactams are fundamental in medicinal chemistry, acting as essential elements for various therapeutic agents due to their structural adaptability and capability to enhance biological activity. In their synthesis, transition metals play a pivotal role as catalysts, offering more efficient alternatives to traditional methods by facilitating C–N bond formation through mechanisms like intramolecular coupling. Recent advances have especially spotlighted transition-metal-catalyzed C–H amination reactions for directly converting C(sp2)–H to C(sp2)–N bonds, streamlining the creation of these compounds. Furthermore, biocatalytic approaches have emerged, providing asymmetric synthesis of lactams with high yield and enantioselectivity. This review examined the transition metal-catalyzed synthesis techniques for producing benzo-fused γ-lactams, marking a significant leap in organic synthesis by proposing more effective, selective, and greener production methods. It serves as a valuable resource for researchers in the fields of transition metal catalysts and those engaged in synthesizing these lactams.
 Mosstafa Kazemi | Mosstafa Kazemi was born in Ilam, Iran. He has received MS degree in organic chemistry from Ilam University in 2013, his PhD degree in organic chemistry from Ilam University in 2018. Dr Kazemi is interested in the development of novel synthetic methods, nanocatalysts and particularly involving the application of magnetic nanocatalysts in chemical reactions. |
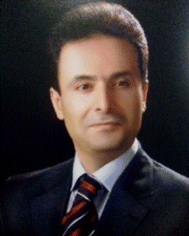 Ramin Javahershenas | Ramin Javahershenas was born in Urmia, Iran, in 1971. He received his BSc in applied chemistry from Tabriz University, Tabriz, Iran in 1993, his MSc in organic chemistry from Urmia University, Urmia, Iran under the supervision of Professor Naser Ardabilchi in 1999, and his PhD in organic chemistry from Urmia University, Urmia, Iran under the supervision of Professor Jabbar Khalafy in 2017. His research interests canter around organic synthesis and include heterocyclic synthesis, asymmetric synthesis, natural products synthesis, synthetic methodology, and applications of various catalysts in multicomponent reactions. |
1. Introduction
1.1. Catalysis
The synthesis of benzo-fused γ-lactams has emerged as a focal point in organic chemistry due to their significant presence in a variety of pharmaceutically active compounds.1,2 These structures, which include prominent derivatives such as isoindolin-2-ones and 2-oxindoles, are integral to the development of drugs targeting neurodegenerative disorders, cancer, and infectious diseases.3,4 The structural and functional versatility of benzo-fused γ-lactams makes them valuable scaffolds in medicinal chemistry, often leading to the development of compounds with enhanced biological activity and specificity.5,6
Multicomponent reactions (MCRs) involving transition metal catalysts have gained significant attention as powerful strategies for the rapid assembly of benzo-fused γ-lactams.3 These reactions, which combine three or more reactants in a single operation to form a complex product, are highly atom-economical and offer the flexibility to introduce diverse functional groups into the lactam framework.7–9 The catalytic role of transition metals in these MCRs is crucial, not only enhancing reaction efficiency but also providing control over regio- and stereoselectivity. This control is particularly important in pharmaceutical applications, where the spatial arrangement of atoms can vastly influence the activity and selectivity of the resulting compounds.10–12
Catalysts indeed play a pivotal role in chemical reactions, acting as agents that significantly increase the rate of reaction without being consumed in the process.13,14 They function by providing an alternative reaction pathway with a lower activation energy, which allows reactants to convert into products more efficiently.15,16 This characteristic is crucial in both industrial applications and biological systems, where catalysts facilitate complex reactions at lower temperatures and with greater precision than would otherwise be possible.17 In industry, catalysts are essential for the production of various chemicals, pharmaceuticals, and fuels, optimizing processes to be more energy-efficient and cost-effective.18,19 Moreover, in biological systems, enzymes, which are nature's catalysts, are fundamental for sustaining life, driving the myriad of biochemical reactions necessary for organisms to function.20,21 The study and application of catalysts are therefore a cornerstone of chemistry and a key factor in advancing technology and medicine.22
Transition metal catalysis has revolutionized the multicomponent synthesis of benzo-fused γ-lactams, offering pathways that are more efficient, selective, and environmentally friendly compared to traditional methods. Transition metals such as palladium, copper, and ruthenium have unique electronic and geometric properties that facilitate key transformations, including intramolecular C–N bond formation, which is pivotal for constructing γ-lactam rings. Recent advancements in transition metal-catalyzed methodologies have underscored their ability to streamline synthesis processes, reduce the need for protection–deprotection steps, and allow for direct functionalization of complex intermediates. Heterogeneous catalysts indeed play a pivotal role in chemical reactions, particularly in industrial processes.23
Their importance stems from their ability to facilitate reactions at the interface of different phases, typically solid catalysts and gas or liquid reactants.24 This allows for a more efficient and selective production process, which is crucial for large-scale manufacturing.25 The versatility and robustness of heterogeneous catalysts make them favorable for continuous processes, contributing significantly to the global economy with approximately 35% of the world's GDP being influenced by catalysis.26 Moreover, they are integral in the production of a vast majority of chemicals, with solid catalysts assisting in about 90% of chemical production by volume.27 The ease of separation from the product stream and the potential for catalyst reuse further underscore their economic and environmental benefits.28
Transition metals serve as pivotal catalysts in synthesizing benzo-fused lactams, a class of compounds with significant pharmaceutical applications. Transition metals' unique electronic and geometric configurations facilitate various bond-forming reactions, including the crucial C–N bond formation necessary for lactam synthesis. Recent advancements have highlighted the efficiency of transition-metal-catalyzed reactions in forming benzo-fused lactams through intramolecular coupling processes. These methods offer more reliable and convenient access to the desired lactam structures compared to traditional multistep synthetic approaches.29,30 For instance, μ-oxo-hypervalent-iodine-catalyzed oxidative C–H amination has been employed for the synthesis of benzo-fused γ-lactams derivatives, showcasing the ability to introduce various functional groups into the lactam ring. Additionally, the development of biocatalytic methods for the asymmetric synthesis of enantioenriched lactams via intramolecular C–H amidation of dioxazolones represents a significant leap forward, providing high yields and enantioselectivity. These methodologies not only streamline the synthesis process but also enhance the diversity of accessible benzo-fused lactam derivatives. The strategic use of transition metals in these catalytic processes underscores their indispensable role in modern synthetic chemistry, particularly in the construction of complex molecules with high precision and efficiency.31,32
Moreover, the versatility of transition metals allows for a wide range of catalytic activities, including oxidation, reduction, and complex formation, all of which are beneficial in the formation of benzo-fused lactams. Their catalytic cycles often involve the formation of intermediate complexes where the metal temporarily bonds with reactants, bringing them into close proximity and the correct orientation to facilitate the reaction.33,34 This can lead to increased reaction rates and improved yields. Furthermore, the reusability of transition metal catalysts presents an economic advantage, as they can often be recovered and recycled, minimizing waste and environmental impact. In the context of benzo-fused lactams, transition metals can catalyze key steps such as the formation of carbon–nitrogen bonds, which is a critical aspect of lactam synthesis.35,36 They can also enable cross-coupling reactions that form carbon–carbon bonds between aromatic rings and other components, a crucial step in constructing the benzo-fused structure. The ability to carry out these reactions with high precision and control over stereochemistry is another benefit, as it allows for the synthesis of complex molecules with the desired spatial arrangement, which can significantly affect the biological activity of the resulting compounds.37
This manuscript delves into the current advances and methodologies involving transition metals for the synthesis of benzo-fused γ-lactams. By evaluating the mechanistic pathways, substrate scope, and catalytic efficiencies of these processes, we aim to provide a comprehensive overview of the state-of-the-art in this field. Moreover, this work seeks to highlight the potential for future research, exploring new catalysts, reaction conditions, and applications in drug development, ultimately emphasizing the indispensable role of transition metals in modern synthetic chemistry.
1.2. Transition metal catalysts
Transition metals play a pivotal role in the field of catalysis, which is essential for numerous chemical reactions, particularly in industrial processes.38 These metals, characterized by their d-orbitals which are not fully occupied, exhibit a unique ability to exist in multiple oxidation states.39 This versatility allows them to facilitate reactions by transferring electrons to or from the reactant molecules, effectively lowering the activation energy required for the reaction to proceed.40,41 The variable oxidation states of transition metals enable them to form complex ions with reactants, providing an alternative pathway for the reaction that is more energetically favorable.42,43
Moreover, transition metals can form coordination compounds with a wide variety of ligands, which can significantly alter the reactivity of the metal and thus influence the catalytic process.44 This ability to bind with other atoms and molecules in specific geometries allows for the precise control over the reaction mechanism and the formation of desired products.45,46 For instance, the catalytic properties of transition metals are exploited in the Haber process for ammonia synthesis, where iron acts as a catalyst, and in the hydrogenation of unsaturated fats, where nickel is used.47
The catalytic activity of transition metals is not only limited to their electron transfer capabilities but also includes their potential to facilitate bond breaking and formation, which is crucial in many organic transformations.48,49 This is particularly evident in processes like olefin metathesis, where metals like ruthenium are used to rearrange alkenes, and in cross-coupling reactions, where palladium catalysts are employed to form carbon–carbon bonds.50,51
In environmental applications, transition metals such as vanadium and titanium are used in the catalytic converters of automobiles to reduce harmful emissions, showcasing their importance in pollution control.52 In the pharmaceutical industry, transition metal-catalyzed reactions are instrumental in the synthesis of complex molecules, including drugs and active pharmaceutical ingredients.53
The significance of transition metals in catalysis is underscored by the fact that they are at the heart of many Nobel Prize-winning discoveries in chemistry, reflecting their profound impact on the advancement of science and technology.54 Their continued study and application hold the promise of more efficient, sustainable, and cost-effective chemical processes, which is vital for meeting the growing demands of modern society and industry.55 Transition metals, therefore, are not just elements on the periodic table; they are the workhorses of catalysis, driving reactions that are fundamental to both life and industry.56
In summary, the extraordinary properties of transition metals as catalysts stem from their electronic structure and the ability to participate in various chemical interactions.57,58 These properties have been harnessed in countless applications, from industrial manufacturing to the biological processes within our own bodies, making transition metals indispensable in the realm of chemistry.59,60
1.3. Benzo-fused γ-lactams
Benzo-fused lactams are a significant class of compounds with a wide array of applications across various fields due to their unique structural features.61 Biologically, these compounds are integral in drug design and discovery, particularly for their therapeutic potential in treating diseases such as cancer, diabetes, and infectious diseases.62,63 Their role in pharmaceuticals is equally crucial, with many benzo-fused lactams forming the backbone of drugs with anxiolytic, anticancer, anti-inflammatory, and antibiotic properties.64 Industrially, the synthesis of these lactams, often through multicomponent reactions, is valued for its efficiency and the ability to save raw materials and time, which is paramount in high-throughput chemical production.65 Chemically, lactams serve as building blocks for novel amino acids, alkaloids, and peptidomimetic compounds, showcasing their versatility and importance in synthetic chemistry.66 The biological applications of benzo-fused lactams are rooted in their bioactivity profile.67 They have been identified as key components in the design of drugs due to their variety of potential therapeutic applications.68 For instance, lactams have been used to improve the potency, selectivity, and metabolic stability of peptide-based drugs, with specific lactams acting as HIV-1 integrase inhibitors, opioid receptor agonists, as well as antitumoral and antidepressant agents.69,70 In the pharmaceutical realm, these lactams are part of many active molecules, including those used as anxiolytics and anticancer agents.70,71 Notably, lenalidomide, a derivative of thalidomide, is a prominent example of a benzo-fused lactam with significant anticancer activity.4 In industrial settings, the synthesis of benzo-fused lactams is often achieved through multicomponent reactions, which are advantageous for their efficiency in saving raw materials and working time.72 This is particularly beneficial in combinatorial chemistry and diversity-oriented synthesis, where structurally diverse compound libraries can be rapidly synthesized for high-throughput screening.73 The chemical applications of these lactams are extensive, as they are considered significant heterocycles in medicinal chemistry and drug discovery.74 They are conformationally restricted scaffolds with peptidomimetic features, which are used to enhance the design and synthesis of novel compounds.75 Moreover, their structural diversity allows for the targeting of specific biological activities, making them valuable in the development of new therapeutic agents.76 The structure of several bioactive benzo-fused γ-lactam derivatives are shown in Fig. 1.
 |
| Fig. 1 Several bioactive benzo-fused γ-lactam derivatives. | |
Overall, benzo-fused lactams are multifaceted compounds that play a pivotal role in advancing biological research, pharmaceutical development, industrial synthesis, and chemical innovation.77,78 Their broad spectrum of applications underscores their importance in science and industry, making them a focus of ongoing research and development efforts.72–79 The continued exploration of their potential will likely yield new discoveries and advancements in various fields of study.
2. Transition metal catalysts to benzo-fused γ-lactams
Transition metals serve as pivotal catalysts in the synthesis of benzo-fused lactams, a class of compounds with significant pharmaceutical applications.60–65 The unique electronic and structural properties of transition metals facilitate various bond-forming reactions, including the crucial C–N bond formation necessary for lactam synthesis. Recent methodologies exploit these catalysts for intramolecular coupling reactions, which efficiently yield benzo-fused lactams through the activation of aryl halides and amide moieties.66–69 This approach not only streamlines the synthetic process but also enhances the functional group tolerance, allowing for the incorporation of diverse substituents into the lactam framework. Moreover, the use of transition metals in catalysis has been extended to oxidative C–H amination, providing a direct route to C–N bond formation and enabling the synthesis of benzolactams with various functional groups.70–74 Such advancements underscore the versatility and efficiency of transition metals in the realm of organic synthesis, particularly in the construction of complex molecules like benzo-fused lactams. Recently, a number of methods have been described for using transition metal catalysts to produce benzo-fused γ-lactam molecules. In this review, we studied the methods reported for the synthesis of benzo-fused γ-lactams based on using transition metals as the catalyst. It is noteworthy that the review paper can be useful for researchers working on transition metal catalysts and chemists synthesizing benzo-fused γ-lactams.76–79
2.1. Copper catalysts for the synthesis of benzo-fused γ-lactams
Copper is a highly valued catalyst in chemical reactions due to its excellent conductive properties, favorable redox potential, and ability to exist in multiple oxidation states.80–82 These characteristics enable copper to facilitate a wide range of chemical transformations, making it a versatile catalyst.83,84 Particularly, copper-based nanoparticles have gained attention for their role in coupling reactions, where they offer a cost-effective and sustainable alternative to noble metals like palladium.85,86 The natural abundance and low toxicity of copper further contribute to its appeal in organic synthesis, where it's used to catalyze multicomponent synthesis of heterocycles.87–89 This not only underscores the importance of copper in modern chemistry but also highlights its potential in advancing eco-friendly and economically viable catalytic processes.90–92 Copper's catalytic efficiency and the ease with which it can be reduced make it a subject of extensive study, especially in the context of producing hydrogen from chemical storage materials.93 The ongoing research and development in copper-catalyzed reactions reflect the metal's significant role in the future of catalysis and its contribution to sustainable industrial practices.94
In this respect, research group of lee reported an attractive and efficient procedure for the synthesis of isoindolin-1-ones via CuI-catalyzed one-pot three-component reaction of 2-iodobenzoic acids, alkynylcarboxylic acids and ammonium acetate in the presence of cesium carbonate as base (Scheme 1).16,95 To find the optimized conditions, the model reaction of 2-iodobenzoic acid, phenylpropiolic acid and ammonium acetate catalyzed by copper salts using different bases in several solvents. Using 10 mol% of CuI and cesium carbonate, a series of aryl alkynylcarboxylic acids afforded the corresponding isoindolin-1-ones with good yields in DMSO at 120 °C. Authors suggested a rational pathway for the synthesis of isoindolin-1-ones under the described conditions which is shown in Scheme 2. Based on presented mechanism, the coupled alkyne moiety is activated by Cu(I) and then base-promoted cyclization occurs.
 |
| Scheme 1 Synthesis of isoindolin-1-ones via CuI-catalyzed one-pot three-component reaction of 2-iodobenzoic acids, alkynylcarboxylic acids and ammonium acetate. | |
 |
| Scheme 2 Rational pathway for synthesis of isoindolin-1-ones via CuI-catalyzed one-pot three-component reaction of 2-iodobenzoic acids, alkynylcarboxylic acids and ammonium acetate. | |
At the same time, research group of Sarkar have reported the fabrication of cubic cuprous oxide nanoparticles as a novel and efficient nanocatalyst for the synthesis of isoindolinones.96 After comprehensive experimental, 10 mol% of Cu2O in the presence of cesium carbonate was considered as the optimized conditions for the multi-component one-pot reaction involving 2-iodo-N-phenylbenzamides, terminal alkyne and substituted indoles/pyrroles in aqueous medium (Scheme 3).4 Authors suggested a rational pathway for the synthesis of 3-alkyl/aryl-3-(pyrrole/indole-2/3-yl)-2-phenyl-2,3-dihydro-isoindolinones under the described conditions which is shown in Scheme 4.
 |
| Scheme 3 Synthesis of 3-alkyl/aryl-3-(pyrrole/indole-2/3-yl)-2-phenyl-2,3-dihydro-isoindolinones via Cu2O-catalyzed multi-component one-pot reaction involving 2-iodo-N-phenylbenzamides, terminal alkyne and substituted indoles/pyrroles. | |
 |
| Scheme 4 Rational pathway for synthesis of 3-alkyl/aryl-3-(pyrrole/indole-2/3-yl)-2-phenyl-2,3-dihydro-isoindolinones via Cu2O-catalyzed multi-component one-pot reaction involving 2-iodo-N-phenylbenzamides, terminal alkyne and substituted indoles/pyrroles. | |
In another publication, Sun and coworkers have reported the preparation of isoindolinones derivatives via copper-catalyzed one-pot three-component reactions of 2-formylbenzoate, primary amines and terminal alkynes in toluene (Scheme 5).97 It is noteworthy that the presence of electron-withdrawing groups on the meta or para position can reduce the yield compared with electron-donating groups.
 |
| Scheme 5 Synthesis of isoindolinones via Cu(OTf)2-catalyzed one-pot three-component reactions of 2-formylbenzoate, primary amines and terminal alkynes. | |
A series of biologically active 2,3-diarylisoindolin-1-one derivatives have been synthesized with moderate to high yields through copper-catalyzed one-pot three-component annulations of 2-formylbenzonitriles, arenes, and diaryliodonium salts.98 The presence of a catalyst was very vital for the performance of reactions; the model reaction failed in the absence of a catalyst. After comprehensive experiments, the utilization of Cu(OAc)2 (5 mol%) in DCE was considered as the optimal system for the synthesis of 2,3-diarylisoindolin-1-one derivatives under reflux conditions (Scheme 6).4 Bases on tentative mechanism presented by authors, the target product is generated through Friedel–Crafts reaction between cation intermediate and mesitylene (Scheme 7).
 |
| Scheme 6 Synthesis of 2,3-diarylisoindolin-1-one derivatives through copper-catalyzed one-pot, three-component annulations of 2-formylbenzonitriles, arenes, and diaryliodonium salts. | |
 |
| Scheme 7 Rational pathway for synthesis of 2,3-diarylisoindolin-1-one derivatives through copper-catalyzed one-pot, three-component annulations of 2-formylbenzonitriles, arenes, and diaryliodonium salts. | |
In two similar publication, Li and coworkers have reported the utilization of copper catalysts for the synthesis of isoindolinone derivatives. A diverse range of pentacyclic iso-indolinone derivatives were synthesized with good yields through Cu-catalyzed three-component cascade cyclization among 2-formylbenzonitrile, cyclopropyl ketones, and diary liodonium salts in DCE under reflux conditions (Scheme 8).99 The proposed mechanism involved a ring expansion of cyclopropyl ketones/formation of N-acyliminium/hetero-[4 + 2]-cycloaddition process (Scheme 9). This research group also reported that one-pot three-component cascade cyclization among 2-formylbenzonitriles, alkyl aryl ketones/prop-1-en-2-ylbenzene and diaryliodonium salts in the presence of Cu(OTf)2 in DCM is an efficient system for the synthesis of poly-substituted isoindolinone derivatives (Scheme 10).100 Bases on tentative mechanism presented by authors, the synthesis of dihydroisoindolo[2,1-a]quinolin-11(5H)-ones was achieved via C–N bond and a cascade intermolecular C–C bond formation (Scheme 11).
 |
| Scheme 8 Synthesis of pentacyclic iso-indolinone derivatives through Cu-catalyzed three-component cascade cyclization among 2-formylbenzonitrile, cyclopropyl ketones, and diary liodonium salts. | |
 |
| Scheme 9 Rational mechanism for synthesis of pentacyclic iso-indolinone derivatives through Cu-catalyzed three-component cascade cyclization among 2-formylbenzonitrile, cyclopropyl ketones, and diary liodonium salts. | |
 |
| Scheme 10 Synthesis of dihydroisoindolo[2,1-a]quinolin-11(5H)-ones through Cu(OTf)2-catalyzed one-pot three-component cascade cyclization among 2-formylbenzonitriles, alkyl aryl ketones/prop-1-en-2-ylbenzene and diaryliodonium salts. | |
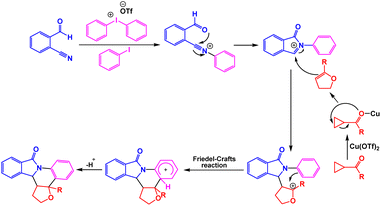 |
| Scheme 11 Rational mechanism for synthesis of dihydroisoindolo[2,1-a]quinolin-11(5H)-ones through Cu(OTf)2-catalyzed one-pot three-component cascade cyclization among 2-formylbenzonitriles, alkyl aryl ketones/prop-1-en-2-ylbenzene and diaryliodonium salts. | |
In order to synthesize sulfonated oxindoles, Cheng and coworkers reported one-pot three-component reaction of N-(arylsulfonyl)-acrylamides, DABSO, and aryldiazonium tetrafluoroborates in the presence of copper as the catalyst.101 Only 48% of the model product was observed in the absence of copper catalyst. Using catalytic amount of Cu(OAc)2 in the presence of HOAc, one-pot three-component reactions of N-(arylsulfonyl)-acrylamides, DABSO, and aryldiazonium tetrafluoroborates were accomplished under nitrogen conditions (Scheme 12). Based on rational mechanism presented by authors, the in situ generated arylsulfonyl radical via addition of an aryl radical to sulfur dioxide and the subsequent single electron transfer served to be the key steps for the reaction (Scheme 13).
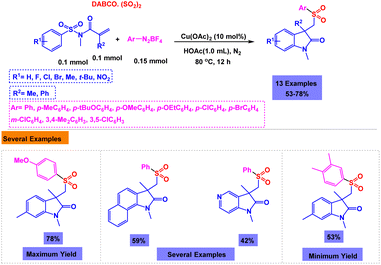 |
| Scheme 12 Synthesis of sulfonated oxindoles through copper-catalyzed one-pot three-component reaction of N-(arylsulfonyl)-acrylamides, DABSO, and aryldiazonium tetrafluoroborates. | |
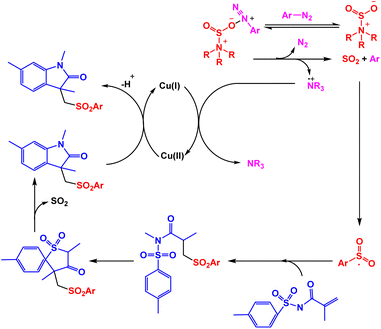 |
| Scheme 13 Rational mechanism for synthesis of sulfonated oxindoles through copper-catalyzed one-pot three-component reaction of N-(arylsulfonyl)-acrylamides, DABSO, and aryldiazonium tetrafluoroborates. | |
2.2. Palladium catalysts for the synthesis of benzo-fused γ-lactams
Palladium is a highly valued catalyst in chemical reactions due to its unique ability to accelerate a variety of organic transformations.102 Its significance is highlighted by its role in cross-coupling reactions, which are crucial for forming carbon–carbon bonds, an essential process in organic synthesis.103 The versatility of palladium catalysis is evident in its application across multiple reactions such as Suzuki–Miyaura, Stille, and Heck couplings, which are instrumental in the production of pharmaceuticals, agricultural chemicals, and organic materials.102–106 One of the key advantages of using palladium as a catalyst is its selectivity,107 which ensures the formation of the desired product without unwanted by-products.61,89 Additionally, palladium catalysts can operate under milder conditions than other metals, leading to more efficient and environmentally friendly processes.59,108,109 The importance of palladium-catalyzed reactions was recognized with the awarding of the Nobel Prize in Chemistry in 2010, underscoring their profound impact on the field of organic chemistry.110,111
In 2014, Mancuso and coworkers have reported that palladium iodide is an efficient catalyst for the synthesis of biologically active 3-[(dialkylcarbamoyl)methylene]isoindolin-1-ones through the intermediate formation of the corresponding 2-ynamide derivatives followed by intramolecular nucleophilic attack by the nitrogen of the benzamide moiety on the conjugated triple bond (Scheme 14).112 PdI2-catalyzed oxidative carbonylation conditions were accomplished in acetonitrile under reflux conditions. Authors suggested a rational pathway for the PdI2-catalyzed oxidative carbonylation conditions which is shown in Scheme 15.
 |
| Scheme 14 Synthesis of 3-[(dialkylcarbamoyl)methylene]isoindolin-1-ones through PdI2-catalyzed oxidative carbonylation conditions. | |
 |
| Scheme 15 Rational pathway for synthesis of 3-[(dialkylcarbamoyl)methylene]isoindolin-1-ones through PdI2-catalyzed oxidative carbonylation conditions. | |
An general, attractive and highly efficient approach for the synthesis of isoindolo[2,1-a]quinazoline derivatives was developed by research group of Guo via palladium-catalyzed one-pot three-component cascade reaction of 2-aminobenzamides with 2-bromobenzaldehydes and carbon monoxide under atmospheric pressure.113 The model reaction was failed in the absence of catalyst or ligand. Under the standardized conditions (Scheme 16), a broad range of 6,6a-dihydroisoindolo[2,1-a]quinazoline-5,11-diones were synthesized with good yields. Based on rational pathway presented in Scheme 17, the intramolecular nucleophilic attack of NH on the carbonyl group of acyl-palladium complex II gives the target product and the active Pd(0) species.
 |
| Scheme 16 Synthesis of 6,6a-dihydroisoindolo[2,1-a]quinazoline-5,11-diones via palladium-catalyzed one-pot three-component cascade reaction of 2-aminobenzamides with 2-bromobenzaldehydes and carbon monoxide. | |
 |
| Scheme 17 Rational pathway for synthesis of 6,6a-dihydroisoindolo[2,1-a]quinazoline-5,11-diones via palladium-catalyzed one-pot three-component cascade reaction of 2-aminobenzamides with 2-bromobenzaldehydes and carbon monoxide. | |
Bengali and coworkers have synthesized a diverse range of polysubstituted β-lactams via one-pot three-component reaction of chloro-quinolinecarbaldehydes with isocyanides and aromatic amines using Pd(PtBu3)2 as the catalyst in the presence of NEtiPr2 and Bu4NCl in acetonitrile under mild conditions (Scheme 18).114 A number of ligands and palladium catalysts were tested to find the best conditions, but the best results were seen using 10 mol% of Pd(PtBu3)2 under ligand-free conditions. A tentative mechanism for the palladium catalyzed one-pot ligand-free three-component reaction of chloro-quinolinecarbaldehydes with isocyanides and aromatic amines is shown in Scheme 19.
 |
| Scheme 18 Synthesis of isoindoles through palladium catalyzed one-pot three-component ligand-free reaction of chloro-quinolinecarbaldehydes with isocyanides and aromatic amines. | |
 |
| Scheme 19 Tentative mechanism for synthesis of isoindoles through palladium catalyzed one-pot three-component ligand-free reaction of chloro-quinolinecarbaldehydes with isocyanides and aromatic amines. | |
In another publication, a library of N-substituted isoindolinones were synthesized with good yields have been reported by Grigg and coworkers, through palladium catalyzed three-component arbonylation–amination–intramolecular Michael addition cascade process.115 Under the optimized conditions (Scheme 20), reaction tolerates a wide variety of N-nucleophiles and delivers a diverse range of isoindolinones.
 |
| Scheme 20 Synthesis of N-substituted isoindolinones through palladium catalyzed three-component arbonylation–amination–intramolecular Michael addition cascade process. | |
Shiri and coworkers have found that the utilization of 1 mol% of palladium acetate and cesium carbonate is an efficient catalytic system for the synthesis of 3-aminoisoindolinone derivatives through one-pot three-component reactions of chloro-quinolinecarbaldehydes with isocyanides and aromatic amines in non-polar medium (Scheme 21).116 The presence of palladium catalyst very vital for these reactions, because the model reaction was not accomplished in the absence of catalyst.
 |
| Scheme 21 Synthesis of 3-aminoisoindolinone derivatives through palladium catalyzed one-pot three-component reactions of chloro-quinolinecarbaldehydes with isocyanides and aromatic amines. | |
Skrydstrup and coworkers have developed a facile and efficient synthetic methodology for the preparation of fluorinated 3-methyleneoxindole derivatives via one-pot three-component reaction of 2-ethynylanilines, per-fluoroalkyliodides and carbon monoxide in the presence of Pd(PPh3)2Cl2 as the catalyst.117 The presence of catalyst was very important for the synthesis of fluorinated 3-methyleneoxindoles. Details of the one-pot three-component reactions of 2-ethynylanilines, per-fluoroalkyliodides and carbon monoxide under the standardized conditions are shown in Scheme 22.4 In another report, Dondas and coworkers have presented a catalytic system for the one-pot three-component reaction of acrylamide, CO and 1,4-benzodiazepine under palladium catalysis to give a 1
:
1 mixture of diastereoisomers of oxindole with good yield (Scheme 23).118
 |
| Scheme 22 Synthesis of fluorinated 3-methyleneoxindole derivatives through Pd(PPh3)2Cl2 catalyzed one-pot three-component reactions of 2-ethynylanilines, per-fluoroalkyliodides and carbon monoxide. | |
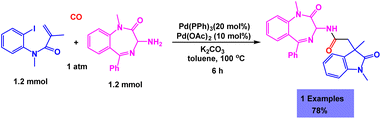 |
| Scheme 23 Synthesis of diastereoisomers of oxindole through palladium catalyzed one-pot three-component reaction of acrylamide, CO and 1,4-benzodiazepine. | |
Research group of Shin have established an attractive and highly efficient approach for the synthesis of biologically active 3-(1,3-diarylallylidene)oxindoles through palladium-catalyzed one-pot three-component reaction of N-arylpropiolamides, aryl iodides and boronic acids.119 This catalytic system allows a stereoselective approach to each (E)- and (Z)-isomer by ligand change and controlling the reaction temperature. Authors reported that the PPh3 ligand promoted the formation of the E-isomer as the main compound while the t-BuXPhos ligand induced the preferential formation of the Z-isomer (Scheme 24).
 |
| Scheme 24 Synthesis of 3-(1,3-diarylallylidene)oxindoles through palladium-catalyzed one-pot three-component reaction of N-arylpropiolamides, aryl iodides and boronic acids. | |
2.3. Other metal catalysts for the synthesis of benzo-fused γ-lactams
Ruthenium complexes have gained prominence in the field of synthetic and medicinal chemistry due to their effectiveness in catalyzing multicomponent reactions (MCRs).120 Ruthenium catalysts are particularly noted for their affordability and high activity, offering a versatile range of oxidation states that facilitate various organic transformations.121 Their application extends to the synthesis of complex pharmaceuticals and natural products, reflecting the significant impact of ruthenium chemistry on organic synthesis in recent years.122 In this respect, a series of biologically active sulfonated 1-isoindolinones have been synthesized with good yields through ruthenium-catalyzed one-pot four-component reaction of 2-vinylbenzoic acids, aryldiazonium tetrafluoroborates, the sulfur dioxide surrogate DABCO-(SO2)2, and nitriles.123 A number of ruthenium complexes and metal salts were tested to find the optimization conditions, the model reaction was not accomplished in the absence of catalyst. After comprehensive experiments, 5 mol% of Ru(bpy)3(PF6) and BF3·OEt2 under blue LED was considered as the optimal conditions for the synthesis of sulfonated 1-isoindolinones (Scheme 25).4 Bases on tentative mechanism presented by authors, intramolecular nucleophilic attack of the carboxy group in iminium-intermediate ion followed by rearrangement of intermediate delivered isoindolinone products (Scheme 26).
 |
| Scheme 25 Synthesis of sulfonated 1-isoindolinones via ruthenium-catalyzed one-pot four-component reaction of 2-vinylbenzoic acids, aryldiazonium tetrafluoroborates, the sulfur dioxide surrogate DABCO-(SO2)2, and nitriles. | |
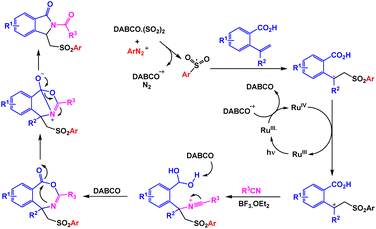 |
| Scheme 26 Rational mechanism for synthesis of sulfonated 1-isoindolinones via ruthenium-catalyzed one-pot four-component reaction of 2-vinylbenzoic acids, aryldiazonium tetrafluoroborates, the sulfur dioxide surrogate DABCO-(SO2)2, and nitriles. | |
Indium complexes have emerged as powerful catalysts in the synthesis of heterocycles, offering a versatile approach to these crucial compounds in pharmaceuticals and agrochemicals.124 Their unique properties allow for efficient and selective transformations, often under mild conditions.125 Recent studies have highlighted the potential of indium(III) complexes, not only as catalysts but also as precursors for various nanoparticles and materials in the industry.125 In 2017, research group of Cai have established a general and highly efficient methodology for the synthesis of biologically active 3-oxoisoindoline-1-difluoroalkyl derivatives via In(OTf)3-catalyzed one-pot, three-component Mannich/lactamization cascade reactions.126 To find the best conditions, the model reaction of methyl 2-formylbenzoate, p-methoxyaniline and difluoroenoxysilane was catalyzed by a number of metal salts in different solvents. As shown in Scheme 27, one-pot, three-component Mannich/lactamization cascade reactions were accomplished using 10 mol% indium catalyst in CH2Cl2 under mild conditions. Based on mechanism presented in Scheme 28, condensation of 2-formylbenzoic acid with amines afforded imines. Imines could be activated by In(OTf)3 and then reacted with difluoroenoxysilane to give the corresponding Mannich-type adduct, which could furnish the target isoindolinone via dehydration.
 |
| Scheme 27 Synthesis of 3-oxoisoindoline-1-difluoroalkyl derivatives via In(OTf)3-catalyzed one-pot, three-component Mannich/lactamization cascade reactions. | |
 |
| Scheme 28 Rational pathway for synthesis of 3-oxoisoindoline-1-difluoroalkyl derivatives via In(OTf)3-catalyzed one-pot, three-component Mannich/lactamization cascade reactions. | |
3. Conclusion
Benzo-fused γ-lactams, like isoindolin-2-ones and 2-oxindoles, are key structures in medicinal chemistry, underpinning a multitude of pharmaceutically active compounds. Their synthesis often utilizes multicomponent reactions to conserve materials and reduce labor, with a growing demand for catalytic systems that support enantioselective preparations. Transition metals play a crucial role, offering unique electronic and geometric properties that facilitate essential C–N bond formation. Recent techniques employing transition-metal-catalyzed processes allow reliable synthesis through intramolecular couplings, with advances like μ-oxo-hypervalent-iodine-catalyzed oxidative C–H amination introducing functional groups into lactams. Biocatalytic methods further enhance synthesis by providing enantioenriched lactams with high yield and selectivity. Transition metals enable oxidation, reduction, and complex formations, improving reaction rates and yields, while their reusability offers economic advantages. They catalyze C–N and C–C bond formations, crucial for constructing benzo-fused structures with precision, influencing the biological activity of target compounds.
Dara availability
No primary research results, software or code have been included and no new data were generated or analyzed as part of this review.
Conflicts of interest
The authors declare no conflict of interest.
References
- J. Lalut, S. Hocine, G. Maertens, M. A. Vilchis-Reyes and S. Hanessian, J. Mol. Struct., 2023, 14, 137104 Search PubMed.
- Z. Tang, Y. Y. Tan, H. Chen and Y. Wan, Curr. Med. Chem., 2022, 30, 372–389 CrossRef PubMed.
- S. G. Pharande, Synthesis, 2021, 53, 418–446 CrossRef CAS.
- E. Martinez de Marigorta, J. M. de los Santos, A. M. Ochoa de Retana, J. Vicario and F. Palacios, Beilstein J. Org. Chem., 2019, 15, 1065–1085 CrossRef PubMed.
- B. Parrino, C. Ciancimino, A. Carbone, V. Spanò, A. Montalbano, P. Barraja, G. Cirrincione and P. Diana, Chem. Inf., 2015, 94, 149 CAS.
- C. Dockendorff, P. W. Faloon, J. Pu, M. Yu, S. Johnston, M. Bennion, M. Penman, T. J. F. Nieland, S. Dandapani, J. Perez, B. Munoz, M. Palmer, S. L. Schreiber and M. Krieger, Bioorg. Med. Chem. Lett., 2015, 25, 2100–2105 CrossRef CAS.
- R. Javahershenas and S. Nikzat, RSC Adv., 2023, 13, 16619 RSC.
- Multicomponent Reactions in Organic Synthesis, ed. J. Zhu, Q. Wang and M. X. Wang, Wiley-VCH, Weinheim, 2015, p. 1 Search PubMed.
- T. J. J. Müller, in Multicomponent Reactions 1: Reactions Involving a Carbonyl Compound as Electrophilic Component, Science of Synthesis: Multicomponent Reactions, Georg Thieme Verlag KG, Stuttgart, 2014, vol. 1 Search PubMed.
- R. Javahershenas, J. Han, M. Kazemi and P. J. Jervis, ChemistrySelect, 2024, e202400185 CAS.
- R. Javahershenas, J. Han, M. Kazemi and P. J. Jervis, ChemistryOpen, 2024, 13, e202400185 CrossRef CAS.
- R. Javahershenas, V. A. Soloshonok, K. D. Klika and P. J. Jervis, Carbon Lett., 2024 DOI:10.1007/s42823-024-00818-x.
- W. Li, Y. Zheng, E. Qu, J. Bai and Q. Deng, Eur. J. Org Chem., 2021, 2021, 5151–5192 CrossRef CAS.
- M. Ghobadi, P. Pourmoghaddam Qhazvini, M. Eslami and M. Kazemi, Synth. Commun., 2021, 51, 325–350 CrossRef CAS.
- P. Du, W. Ran, C. Wang, L. Luo and W. Li, Adv. Mater. Interfaces, 2021, 8, 2100749 CrossRef CAS.
- Z. Chen, S. M. Nasr, M. Kazemi and M. Mohammadi, Mini-Rev. Org. Chem., 2020, 17, 352–362 CrossRef CAS.
- N. Mahato, P. Agarwal, D. Mohapatra, M. Sinha, A. Dhyani, B. Pathak, M. K. Tripathi and S. Angaiah, Processes, 2021, 9, 1544 CrossRef CAS.
- S. Sagadevan, J. A. Lett, G. K. Weldegebrieal, M. R. ud Dowla Biswas, W. C. Oh, S. F. Alshahateet, I. Fatimah, F. Mohammad, H. A. Al-Lohedan, S. Paiman, J. Podder and M. R. Johan, Chem. Phys. Lett., 2021, 780, 138897 CrossRef CAS.
- H.-F. Zhang and R. Jialon, Biol. Mol. Chem., 2023, 1, 82–93 Search PubMed.
- M. B. Chaudhari and B. Gnanaprakasam, Chem.–Asian J., 2019, 14, 76–93 CrossRef CAS.
- H. Lin, L. Wu and M. Kazemi, Synth. Commun., 2021, 1–27 CrossRef.
- M. Ibrahim, M. Labaki, J.-M. Giraudon and J.-F. Lamonier, J. Hazard. Mater., 2020, 383, 121139 CrossRef CAS.
- S. Awasthi, S. K. Pandey, E. Arunan and C. Srivastava, J. Mater. Chem. B, 2021, 9, 228–249 RSC.
- C. Negi, P. Kandwal, J. Rawat, M. Sharma, H. Sharma, G. Dalapati and C. Dwivedi, Appl. Surf. Sci., 2021, 554, 149553 CrossRef CAS.
- Z. Li, L. Wang, L. Qin, C. Lai, Z. Wang, M. Zhou, L. Xiao, S. Liu and M. Zhang, Chemosphere, 2021, 285, 131432 CrossRef CAS PubMed.
- S. Sultana, G. Borah and P. K. Gogoi, Appl. Organomet. Chem., 2019, e4595 CrossRef.
- S. Kanithan, N. Arun Vignesh, K. M. Katubi, P. S. Subudhi, E. Yanmaz, J. Arockia Dhanraj, N. S. Alsaiari, K. M. Abualnaja, M. Sukumar, M. Sundararajan, S. Baskar, S. Sahu and C. S. Dash, J. Mol. Struct., 2022, 1265, 133289 CrossRef CAS.
- Y. Sun, W. Jin and C. Liu, Molecules, 2019, 24, 3838 CrossRef CAS.
- M. N. M. Milunovic, L. M. D. R. S. Martins, V. B. Arion, A. J. L. Pombeiro, in Synthesis and Applications in Chemistry and Materials, ed. A. J. L. Pombeiro, K. T. Mahmudov and M. F. C. Guedes da Silva, World Scientific, 2024, vol. 13, ch. 26, pp. 77–130 Search PubMed.
- T. Kojima, in Redox-based Catalytic Chemistry of Transition Metal Complexes, RSC, 2024, 10.1039/9781837676484-FP001.
- Y. Li, X. Li, Z. An, Y. Chu and X. Wang, Chem.–Asian J., 2023, e202300814 CrossRef CAS.
- T. Sarkar, S. Kar, P. K. Maharana, T. A. Shah and T. Punniyamurthy, in Transition-Metal- Catalyzed C-H Functionalization of Heterocycles, John Wiley & Sons, Inc., 2023, pp. 319–356 Search PubMed.
- V. Tomar, P. Kumar, M. Nemiwal and R. K. Joshi, Inorg. Chem. Commun., 2022, 145, 109982 CrossRef.
- R. Giovanelli, G. Monda, S. Kiriakidi, C. S. López, G. Bertuzzi and M. Bandini, Chem.–Eur. J., 2024, 30, e202401658 CrossRef CAS PubMed.
- H. Cheng, S. Jia and Q.-Q. Zhou, Acc. Chem. Res., 2023, 56, 573–591 CrossRef CAS.
- X. Yang and R. Sun, Adv. Synth. Catal., 2023, 365, 124–141 CrossRef CAS.
- C. Liu, X. Tan, L.-Z. Zhan, Y. Jing, W.-S. Wu, Z. Ke and H. Jiang, Angew. Chem., 2022, 61, e202115221 CrossRef.
- J. N. H. Reek, B. de Bruin, S. Pullen, T. J. Mooibroek, A. M. Kluwer and X. Caumes, Chem. Rev., 2022, 122, 12308–12369 CrossRef CAS PubMed.
- H. Yorimitsu, M. Kotora and N. T. Patil, Chem. Rec., 2021, 21, 3335–3337 CrossRef CAS PubMed.
- S. Sahoo, K. Y. Wickramathilaka, E. Njeri, D. Silva and S. L. Suib, Front. Chem., 2024, 12, 1374878 CrossRef CAS.
- M. Kargar Razi, R. Javahershenas, M. Adelzadeh, M. Ghobadi and M. Kazemi, Synth. Commun., 2020, 50, 3739 CrossRef CAS.
- U. Bin Kim, D. J. Jung, H. J. Jeon, K. Rathwell and S. Lee, Chem. Rev., 2020, 120, 13382–13433 CrossRef PubMed.
- H. Narimani, J. Synth. Chem., 2022, 1, 62–83 Search PubMed.
- S. J. Geier, C. M. Vogels, J. A. Melanson and S. A. Westcott, Chem. Soc. Rev., 2022, 51, 8877–8922 RSC.
- Y. Park, Y. Kim and S. Chang, Chem. Rev., 2017, 117, 9247–9301 CrossRef CAS.
- M. Ghobadi, P. Pourmoghaddam Qhazvini and M. Kazemi, J. Synth. Chem., 2022, 1, 163–170 Search PubMed.
- R. Tamatam, S.-H. Kim and D. Shin, Front. Chem., 2023, 11, 1140562 CrossRef CAS PubMed.
- S. Changmai, S. Sultana and A. K. Saikia, ChemistrySelect, 2023, 8, e202203530 CrossRef CAS.
- D. Mandal, S. Roychowdhury, J. P. Biswas, S. Maiti and D. Maiti, Chem. Soc. Rev., 2022, 51, 7358–7426 RSC.
- A. Trowbridge, S. M. Walton and M. J. Gaunt, Chem. Rev., 2020, 120, 2613–2692 CrossRef CAS.
- T. Mandal, J. Synth. Chem., 2022, 1, 8–15 Search PubMed.
- X. Wan, Y. Zhang, Y. Nie, K. Zhang, Z. Jin, Z. Zhang, L. Gan, X. Liu and J. He, Transl. Cancer Res., 2023, 12, 2181–2196 CrossRef CAS.
- E. P. Beaumier, A. J. Pearce, X. Y. See and I. A. Tonks, Nat. Rev. Chem., 2018, 3, 15–34 CrossRef.
- K. Osakada, R. Soc. Chem. Adv., 2021, 228–255 CAS.
- S. Tabassum, A. F. Zahoor, K. G. Ali, M. Irfan, S. M. Hussain and R. Akhtar, J. Iran. Chem. Soc., 2022, 19, 3285–3315 CrossRef.
- D. Geng, Chin. J. Org. Chem., 2019, 39, 301 CrossRef CAS.
- H.-H. Zhang, H. Chen, C. Zhu and S. Yu, Sci. China:Chem., 2020, 63, 637–647 CrossRef CAS.
- K. W. Barnett and S. React, Inorg. Met. Chem., 1972, 2, 345–346 Search PubMed.
- M. Mustafa and A. Younes, Nanomater. Chem., 2024, 1, 120–130 Search PubMed.
- N. Kaplaneris and L. Ackermann, Beilstein J. Org. Chem., 2022, 18, 86–88 CrossRef CAS.
- C. Chen, C. Ni, J.-H. Song, L.-Y. Ding, X.-X. Zhang, H. Guo, K. Wang, Z. Chen and B. Zhu, ACS Catal., 2024, 12181–12191 CrossRef CAS.
- T. Ghosh, D. Biswas and S. Bhakta, Chem.–Asian J., 2022, 17, e202200725 CrossRef CAS PubMed.
- C. Golding and H. Lee, Biol. Mol. Chem., 2023, 1, 35–44 Search PubMed.
- N. G. Alves, A. J. S. Alves, M. I. L. Soares and T. M. V. D. Pinho e Melo, Adv. Synth. Catal., 2021, 363, 2464–2501 CrossRef CAS.
- Y. Shi, X. Tan, S. Gao, Y. Zhang, J. Wang, X. Zhang and Q. Yin, Org. Lett., 2020, 22, 2707–2713 CrossRef CAS PubMed.
- X.-C. Yu, Y.-N. Zheng, J.-H. Zhang, J. Zhang, F.-L. Liu, K. Tang, T. Li and W.-T. Wei, ACS Sustain. Chem. Eng., 2022, 10, 6057–6062 CrossRef CAS.
- P. Bhardwaj and N. Kaur, Curr. Org. Chem., 2023, 27, 282–296 CrossRef CAS.
- N.-Q. Shao, Y.-H. Chen, C. Li and D.-H. Wang, Org. Lett., 2020, 22, 7141–7146 CrossRef CAS PubMed.
- H. S. S. Chan, Y. Lu and J.-Q. Yu, Nat. Synth., 2024, 3, 752–762 CrossRef CAS.
- B.-H. Li, K.-L. Li and Q.-Y. Chen, J. Fluorine Chem., 2012, 133, 163–166 CrossRef CAS.
- S. Gao, Y. Zhou, W. Zhang, W. Wang, Y. Yu, Y. Mu, H. Wang, X. Gong, G. Zheng and Y. Feng, Sci. Rep., 2017, 7, 44542 CrossRef PubMed.
- D. Song, X. Cao, J. Wang and S. Ke, Bioorg. Med. Chem. Lett., 2020, 30, 126826 CrossRef CAS.
- E. M. Mekky, A. A. M. Ahmed and A. H. M. Elwahy, Helv. Chim. Acta, 2013, 96, 1290–1297 CrossRef.
- Y. Yamamoto, K. Kinpara, T. Saigoku, H. Nishiyama and K. Itoh, Org. Biomol. Chem., 2004, 2, 1287–1294 RSC.
- J. Xuan, C. G. Daniliuc and A. Studer, Org. Lett., 2016, 18, 6372–6375 CrossRef CAS.
- A. López-Francés, X. del Corte, Z. Serna-Burgos, E. Martínez de Marigorta, F. Palacios and J. Vicario, Molecules, 2022, 27, 3624 CrossRef PubMed.
- B. Li, Y. Park and S. Chang, J. Am. Chem. Soc., 2014, 136, 1125–1131 CrossRef CAS.
- F. He, L. Hou, X. Wu, H. Ding, J. Qu and Y. Chen, CCS Chem., 2023, 5, 341–349 CrossRef CAS.
- S. N. Smith and S. J. Connon, Eur. J. Org Chem., 2021, 2021, 5540–5544 CrossRef CAS.
- S. Ichie and H. Andre, Nanomater. Chem., 2023, 1, 32–45 Search PubMed.
- V. Nakhate and G. D. Yadav, ChemistrySelect, 2017, 2, 7150–7159 CrossRef.
- M.-S. Shafik, J. Synth. Chem., 2023, 1, 163–170 Search PubMed.
- J. Broggi, N. Joubert, V. Aucagne, T. Zevaco, S. Berteina-Raboin, S. P. Nolan and L. A. Agrofoglio, Nucleosides, Nucleotides Nucleic Acids, 2007, 26, 779–783 CrossRef CAS PubMed.
- B. Kaboudin, R. Mostafalu and T. Yokomatsu, Green Chem., 2013, 15, 2266 RSC; E. Soleimani, M. M. Khodaei, N. Batooie and S. Samadi, J. Heterocycl. Chem., 2012, 49, 409–412 CrossRef CAS.
- M. Darroudi, S. Ranjbar, M. Esfandiar, M. Khoshneviszadeh, M. Hamzehloueian, M. Khoshneviszadeh and Y. Sarrafi, Appl. Organomet. Chem., 2020, 34, e5962 CrossRef CAS.
- M. Darroudi, S. Ranjbar, M. Esfandiar, M. Khoshneviszadeh, M. Hamzehloueian, M. Khoshneviszadeh and Y. Sarrafi, Appl. Organomet. Chem., 2020, 34, e5962 CrossRef CAS.
- D. Azarifar, R. Nejat-Yami, Z. Akrami, F. Sameri and S. Samadi, Lett. Org. Chem., 2012, 9, 128–132 CrossRef CAS.
- N. Fajer, H. A. Al-Bahrani, A. A. H. Kadhum and M. Kazemi, J. Mol. Struct., 2024, 1296, 136800 CrossRef.
- M. Krishnan, M. Kathiresan and C. Praveen, Eur. J. Org Chem., 2023, 26, e202201405 CrossRef CAS.
- V. Helan, A. V. Gulevich and V. Gevorgyan, Chem. Sci., 2015, 6, 1928–1931 RSC.
- L. Chen, A. Noory Fajer, Z. Yessimbekov, M. Kazemi and M. Mohammadi, J. Sulfur Chem., 2019, 40, 451–468 CrossRef CAS.
- N. Kazemi and M. Mahdavi Shahri, J. Inorg. Organomet. Polym. Mater., 2017, 27, 1264–1273 CrossRef CAS.
- M. Kazemi, Synth. Commun., 2020, 50, 2114–2131 CrossRef CAS.
- K. Khazenipour, F. Moeinpour and F. S. Mohseni-Shahri, J. Chin. Chem. Soc., 2021, 68, 121–130 CrossRef CAS.
- F. M. Irudayanathan, J. Noh, J. Choi and S. Lee, Adv. Synth. Catal., 2014, 356, 3433–3442 CrossRef CAS.
- S. Sarkar, N. Chatterjee, M. Roy, R. Pal, S. Sarkar and A. K. Sen, RSC Adv., 2014, 4, 7024 RSC.
- L. X. Sun, T. Zeng, D. Jiang, L.-Y. Dai and C.-J. Li, Can. J. Chem., 2012, 90, 92–99 CrossRef CAS.
- L. Liu, S.-H. Bai, Y. Li, L.-X. Wang, Y. Hu, H.-L. Sung and J. Li, J. Org. Chem., 2017, 82, 11084–11090 CrossRef CAS PubMed.
- J. Li, S. Bai, Y. Li, Z. Wang, X. Huo and L. Liu, J. Org. Chem., 2018, 83, 8780–8785 CrossRef CAS; L. Liu, S. Bai, Y. Li, X. Ding, Q. Liu and J. Li, Adv. Synth. Catal., 2018, 360, 1617–1621 CrossRef.
- L. Liu, S. Bai, Y. Li, X. Ding, Q. Liu and J. Li, Adv. Synth. Catal., 2018, 360, 1617–1621 CrossRef CAS.
- H. Wang, S. Sun and J. Cheng, Org. Lett., 2017, 19, 5844–5847 CrossRef CAS; J. Al Shakarji, B. al-Rabi'i, Nanomater. Chem. 2023, 1, 81–93 Search PubMed; P. Shroff and B. Panu, Nanomater. Chem., 2023, 1, 70–80 Search PubMed.
- J. Al Shakarji and B. al-Rabi'i, Nanomater. Chem., 2023, 1, 81–93 Search PubMed.
- P. Shroff and B. Panu, Nanomater. Chem., 2023, 1, 70–80 Search PubMed.
- T. Mandal, Nanomater. Chem., 2024, 1, 108–119 Search PubMed.
- P. So-ho, Y. Han-chul and J. Jae-chan, Nanomater. Chem., 2023, 1, 46–57 Search PubMed.
- A. M. Mustafa and A. Younes, Nanomater. Chem., 2023, 1, 12–23 Search PubMed.
- A. W. Augustyniak, W. Zawartka, J. A. R. Navarro and A. M. Trzeciak, Dalton Trans., 2016, 45, 13525–13531 RSC.
- Z. Moghadasi, A. Noory Fajer, A. M. H. Abudken and H. Ali Al-Bahrani, Nanomater. Chem., 2023, 1, 24–31 Search PubMed.
- P. Hu and M. Kazemi, J. Coord. Chem., 2024, 77, 1324 CrossRef CAS.
- B. Liu, Q. Kuang, Z. Xie and L. Zheng, CrystEngComm, 2015, 17, 6308–6313 RSC.
- C. C. Johansson Seechurn, M. O. Kitching, T. J. Colacot and V. Snieckus, Angew. Chem., Int. Ed., 2012, 51, 5062–5085 CrossRef CAS.
- R. Mancuso, I. Ziccarelli, D. Armentano, N. Marino, S. V. Giofrè and B. Gabriele, J. Org. Chem., 2014, 79, 3506–3518 CrossRef CAS.
- S. Guo, J. Zhai, F. Wang and X. Fan, Org. Biomol. Chem., 2017, 15, 3674–3680 RSC.
- G. M. Torres, M. De La Higuera Macias, J. S. Quesnel, O. P. Williams, V. Yempally, A. A. Bengali and B. A. Arndtsen, J. Org. Chem., 2016, 81, 12106–12115 CrossRef CAS.
- R. Grigg, X. Gai, T. Khamnaen, S. Rajviroongit, V. Sridharan, L. Zhang, S. Collard and A. Keep, Can. J. Chem., 2005, 83, 990–1005 CrossRef CAS.
- M. Shiri, M. Ranjbar, Z. Yasaei, F. Zamanian and B. Notash, Org. Biomol. Chem., 2017, 15, 10073–10081 RSC.
- H. Yin and T. Skrydstrup, J. Org. Chem., 2017, 82, 6474–6481 CrossRef CAS.
- H. A. Dondas, S. Belveren, S. Poyraz, R. Grigg, C. Kilner, M. Ferrándiz-Saperas, E. Selva and J. M. Sansano, Tetrahedron, 2018, 74, 6–11 CrossRef CAS.
- Y. Yu, K. J. Shin and J. H. Seo, J. Org. Chem., 2017, 82, 1864–1871 CrossRef CAS.
- L. Chen, K. G. Malollari, A. Uliana and J. F. Hartwig, J. Am. Chem. Soc., 2021, 143, 4531–4535 CrossRef CAS.
- J. R. Johansson, T. Beke-Somfai, A. Said Stålsmeden and N. Kann, Chem. Rev., 2016, 116, 14726–14768 CrossRef CAS.
- S. Thowfik, C. M. A. Afsina and G. Anilkumar, RSC Adv., 2023, 13, 6246–6263 RSC.
- J. Zhang, F. Zhang, L. Lai, J. Cheng, J. Sun and J. Wu, Chem. Commun., 2018, 54, 3891–3894 RSC.
- R. E. Millán, J. Rodríguez, L. A. Sarandeses, E. Gómez-Bengoa and J. P. Sestelo, J. Org. Chem., 2021, 86, 9515–9529 CrossRef.
- R. Pérez-Guevara, L. A. Sarandeses, M. M. Martínez and J. Pérez Sestelo, Org. Chem. Front., 2022, 9, 6894–6901 RSC.
- T. Chen and C. Cai, New J. Chem., 2017, 41, 2519–2522 RSC.
|
This journal is © The Royal Society of Chemistry 2025 |
Click here to see how this site uses Cookies. View our privacy policy here.