DOI:
10.1039/D4NA00393D
(Review Article)
Nanoscale Adv., 2025,
7, 934-962
Extracellular vesicles: from intracellular trafficking molecules to fully fortified delivery vehicles for cancer therapeutics
Received
10th May 2024
, Accepted 22nd December 2024
First published on 15th January 2025
Abstract
Extracellular vesicles (EVs) are emerging as viable tools in cancer treatment due to their ability to carry a wide range of theranostic activities. This review summarizes different forms of EVs such as exosomes, microvesicles, apoptotic bodies, and oncosomes. It also sheds the light onto isolation methodologies, characterization techniques and therapeutic applications of all discussed EVs. Evidence indicates that EVs are particularly effective in delivering chemotherapeutic medications, and immunomodulatory agents. However, the advancement of EV-based therapies into clinical practice is hindered by challenges including EVs heterogeneity, cargo loading efficiency, and in vivo stability. Overall, EVs have the potential to change cancer therapeutic paradigms. Continued research and development activities are critical for improving EV-based medications and increasing their therapeutic impact.
Introduction
Extracellular Vesicles (EVs) are lipid-bound structures released by all cell types, acting as essential mediators of intercellular communication across diverse organisms.1–4 Initially thought to be mere cellular waste disposal mechanisms,5 the understanding of EVs has evolved dramatically over the past three decades. They are now recognized as crucial players in intracellular trafficking and the transportation of various biomolecules between cells.5–8
EVs represent a captivating class of nanosized, membrane-enclosed particles that act as cellular messengers.9 These dynamic structures, primarily encompassing exosomes and microvesicles (MVs), serve as potent communication tools, shuttling a diverse cargo of molecules between cells.10 Notably, specific cell types and conditions can further diversify EVs, leading to subtypes such as apoptotic bodies shed during programmed cell death and large oncosomes secreted by cancer cells.11,12 This review will delve into the distinct biogenesis, release mechanisms, size, composition, and functional roles of these multifunctional EVs subtypes.1,13,14
EVs act as versatile communication hubs, transporting a meticulously selected cargo that includes DNA, various RNA species like messenger RNA (mRNA)15 and non-coding RNAs (ncRNAs) such as microRNAs (miRNAs),16–18 long non-coding RNAs (lncRNAs),19–22 and circular RNAs (circRNAs).23–25 Additionally, proteins, metabolites, lipids, and glycoconjugates derived from the parent cell can be packaged within EVs.26 The protective lipid bilayer surrounding EVs shields their cargo from degradation by extracellular enzymes, allowing for long-distance travel through the bloodstream to reach distant target tissues.26 The precise mechanisms governing cargo selection and packing remain an active area of research, as the cargo composition within EVs often differs significantly from the parent cell's internal environment.26
The impact of EVs on recipient cells is multifaceted and depends on several factors. These factors include the specific cell types and tissues interacting with the EVs, the cargo content and quantity, and the recipient cell's ability to process and utilize the cargo.26 While the details of how EVs interact with recipient cells and how their cargo is delivered inside remain under investigation, various mechanisms are being actively explored.27
In the context of cancer, EVs emerge as intriguing players. They exhibit a selective packaging and delivery of cargo, fostering a dynamic communication network between tumors and the host organism.28 This intricate interplay significantly influences all aspects of tumor biology, impacting tumor initiation, progression, and metastasis.29–32 EVs, released by both cancerous and non-cancerous cells, can exert local and long-distance effects, influencing various cell types within the tumor microenvironment and potentially affecting all biological systems of the patient.33
The unique properties of EVs, including low toxicity, excellent biocompatibility, low immunogenicity, and inherent targeting capabilities, make them highly promising candidates for drug delivery systems in various diseases, including cancer.34 Research efforts are directed towards engineering therapeutic EVs by modifying normal cells to optimize their cargo and targeting properties.24 While initial studies explored cancer cell lines like HeLa cells for proof-of-concept, the oncological properties of cancer cell-derived EVs are now recognized.35 Consequently, the focus has shifted towards utilizing intact normal cells as sources for therapeutic EVs production.
Types of EVs and their composition
Exosomes
Among the diverse EVs subtypes, exosomes stand out for their diminutive size, typically ranging 30–150 nm in diameter as shown in Table 1.58 These nano-messengers are meticulously produced through the endosomal pathway. This intricate process involves inward budding of the limiting membrane of early endosomes, leading to the formation of multivesicular bodies (MVBs) (Fig. 1).59 Once mature, MVBs can either fuse with the cell membrane and release their exosome cargo into the extracellular space, or they can fuse with lysosomes for degradation. Exosomes play a vital role in a multitude of biological processes.1,13,36,37 They serve as crucial mediators of intercellular and intracellular communication, facilitating the exchange of proteins, lipids, and nucleic acids between cells.59 Their involvement extends to various physiological functions such as immune response stimulation, myelin sheath formation, tissue repair, and neural survival.1,13,37
Table 1 Classification of extracellular vesicles (EVs)
Extracellular vesicles (EVs) |
Size |
Composition |
Biogenesis |
Production and release stimuli |
Markers |
Functions |
Ref. |
Exosomes |
30–150 nm |
Nucleic acids: DNA, mRNA, miRs, other non-coding RNAs |
Endosomal pathway (ESCRT-dependent or independent) |
↑ Intracellular Ca2+, hypoxia |
Alix, CD63, CD9, CD81 TSG101, HSC70, and HSP90β |
Intercellular communications, immune response stimulation, tumor progression, myelin sheath formation, neural survival, tissue repair, others |
1, 2, 6, 13 and 36–46 |
Lipids: ↓ phosphatidylcholine diacylglycerol, phosphatidylinositol, phosphatidylethanolamines |
Terminus (GIPC) depletion |
↑ Glycolipids, free fatty acids, phosphatidylserine |
Presence of TNF-α, heparanase, glutamate |
Some are enriched with cardiolipins and sphingomyelin |
Proteins: CD81, ALix, TSG101, glycoproteins |
Microvesicles |
100–1000 nm |
Nucleic acids: DNA, mRNA, non-coding RNAs |
Outward budding of plasma membrane initiated by translocases and ARF6 |
↑ Intracellular Ca2+, gamma-radiation |
Integrins, selectins, CD40 ligand, ARF6, VCAMP3 and MMP |
Intercellular communications, thrombosis, regulation of inflammatory response and angiogenesis, increasing apoptosis rate, autophagy promotion, cartilage regeneration |
1, 2, 6, 13, 38, 39, 41 and 47–50 |
Lipids: ↓ phosphatidyl-glycerols, phosphatidyl-inositol, phosphatidyl-ethanolamines |
↑ Peptidyl-arginine deiminases (PAD2 & PAD4) induced by BzATP, activation of Rho & ROCK |
Some act as anti-inflammatory modulators |
↑ Ceramides, sphingomyelins |
Some are enriched with cholesterol esters and phosphatidylserine |
Proteins: integrins, selectins, CD40 ligand, ARF6, VCAMP3 adhesion proteins |
Apoptotic bodies |
50–5000 nm |
Cellular organelles |
Outward budding of the plasma membrane of apoptotic cells via caspase-dependent and independent pathways |
Heat, ROS, radiation, ↑ intracellular Ca2+, hypoxia |
Thrombospondin, annexin V, C3b, phosphatidyl-serine |
Debris clearance |
1 and 51–53 |
Nucleic acid: DNA, mRNA, non-coding RNAs |
Immunomodulatory functions |
Lipids: ↑ translocated phosphatidylserine |
Proteins: thrombospondin, annexin V, C3b, TIM4 |
Oncosomes |
Small: 100–400 nm |
Nucleic acid: DNAs, RNAs and non-coding RNAs |
Outward budding of the plasma membrane of tumor cells |
Activation of EGFR & AKT pathways |
ARF6, CK18, GAPDH, MMP, Annexin A1, Annexin A2, oncogenic proteins complexes |
Development, growth and metastasis of tumors |
12 and 54–57 |
Large: 1–10 μm |
Lipids: ↑ phosphatidylserine exposure and flipping |
Silencing DIAPH3 by EKR |
↑ Content of cholesterol |
Proteins: ARF6, MMP, Annexin A1, Annexin A2, oncogenic proteins complexes |
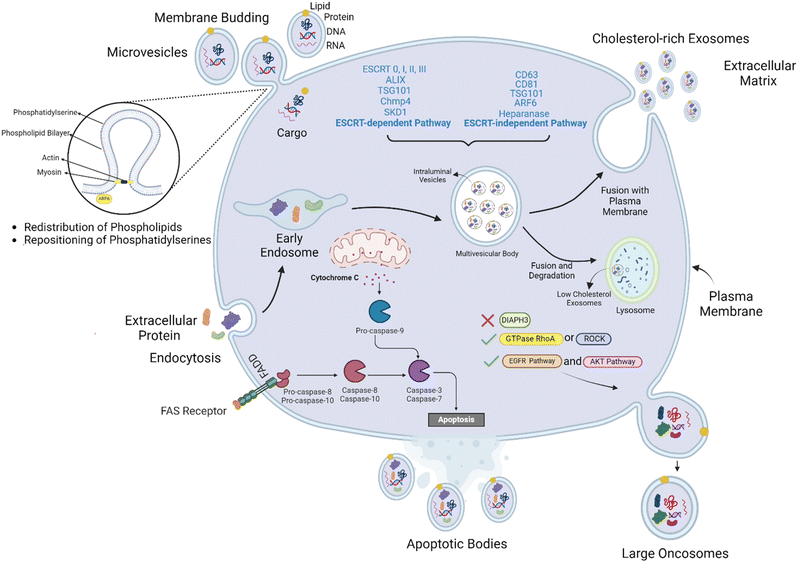 |
| Fig. 1 Biogenesis and secretion of different types of extracellular vesicles. | |
Biogenesis of exsomes is a complex process, exosomes are generated through intricate cellular mechanisms. While the endosomal sorting complex required for transport (ESCRT) machinery plays a pivotal role in their biogenesis, alternative pathways exist. The ESCRT pathway, comprising proteins like ALIX, TSG101, Chmp4, and SKD1, is instrumental in forming multivesicular bodies (MVBs) and subsequent exosome release.1,38,60 However, ESCRT-independent mechanisms involving proteins such as CD63, CD81, TSG101 (note: TSG101 participates in both pathways), ARF6, and heparanase also contribute to exosome production (Fig. 1).38 These complex pathways underscore the diverse mechanisms governing exosome biogenesis and highlight its intricate relationship with cellular processes.
Exosome release is influenced by various factors, including intracellular calcium levels, hypoxia, growth factors, TNF-α, heparanase, and glutamate.61 Once released, exosomes interact with recipient cells through multiple mechanisms, including clathrin-mediated endocytosis, caveolin-mediated endocytosis, macropinocytosis, and direct fusion with the plasma membrane.38,39 These uptake processes determine the intracellular fate of exosomes and their cargo, ultimately influencing cellular responses.
Exosomes are heterogeneous nanovesicles characterized by a lipid bilayer encapsulating a diverse cargo of biomolecules.9,62 This cargo encompasses proteins, lipids, and nucleic acids, including DNA, mRNA, various ncRNAs (miRNAs, lncRNAs, circRNAs), and metabolites.24,58,63–66 The specific composition of exosomes is influenced by their cell of origin, highlighting their potential as biomarkers for distinct cellular states and pathologies.67,68
Lipid composition is a critical determinant of exosome structure and function. While sphingomyelin is enriched in some exosomes, particularly those derived from brain cells, other lipids like cardiolipins can also be abundant.6,40 Notably, exosomal lipid profiles often differ from their parent cells, with elevated levels of glycolipids, free fatty acids, and phosphatidylserine, and decreased levels of phosphatidylcholine, diacylglycerol, phosphatidylinositol, and phosphatidylethanolamines.6,40
Protein content is another key aspect of exosome characterization. Exosomes carry a variety of proteins, including transmembrane and cytoplasmic proteins, with functions spanning cell adhesion, immune response, and intracellular signaling.69 Specific protein markers like CD63, CD9, CD81, ALIX, TSG101, HSC70, and HSP90β are commonly used to identify and isolate exosomes (Table 1). However, the protein composition is highly diverse and reflects the origin and functional state of the parent cell.70
Microvesicles
Microvesicles (MVs) are a heterogeneous population of EVs ranging in size 100–1000 nm.14 Unlike exosomes, which originate from the endosomal pathway, MVs are directly shed from the plasma membrane via outward budding (Fig. 1).70 This process is influenced by a complex interplay of factors, including phospholipid redistribution, cytoskeletal dynamics, and the involvement of specific proteins such as SNAREs, tethering factors, molecular motors, and components of the ESCRT machinery.47,71
MVs carry a diverse cargo, including proteins, lipids, and nucleic acids similar to exosomes.72 Their lipid composition differs significantly from the parental cell membrane, with increased levels of phosphatidylserine and decreased levels of phosphatidylcholine, phosphatidylinositol, and phosphatidylethanolamine.73 Sphingomyelin and ceramides are enriched in MV membranes, while cholesterol ester content varies significantly.40
The protein cargo of MVs is equally complex, encompassing proteins involved in various cellular functions, including signaling, adhesion, and structural maintenance.71 Notably, MVs are enriched in specific marker proteins such as integrins, selectins, CD40 ligand, ARF6, and VCAMP3, facilitating their identification and characterization (Table 1).41,47,48
Apoptotic bodies
Apoptotic bodies, the largest of the EVs subtypes, are generated during programmed cell death or apoptosis.74 These membrane-bound structures, ranging from 50–5000 nm in diameter, encapsulate cellular debris, including organelles and nuclear fragments70 (Fig. 1). Apoptosis, a tightly regulated process, is initiated by various stimuli such as heat, radiation, or oxidative stress and involves distinct pathways, including caspase-dependent and independent mechanisms.74,75
Beyond their role in cellular clearance, apoptotic bodies actively participate in several biological processes. They serve as immunomodulatory agents, influencing immune responses, and are recognized by phagocytes through “eat-me” signals, such as phosphatidylserine exposed on their outer membrane.47
The lipid composition of apoptotic bodies is distinct from that of living cells, with increased phosphatidylserine and oxidized lipids, facilitating their recognition and clearance.47 Protein content is also characteristic, including heat shock proteins and other molecules involved in apoptotic processes.51
In contrast to exosomes and MVs, oxidative modifications to the apoptotic bodies' cell surface create binding sites for proteins like thrombospondin and complement component C3b, further facilitating phagocytosis as summarized in Table 1.47 These markers, along with annexin V and T cell immunoglobulin mucin-4 (TIM4), are commonly used to identify apoptotic bodies.47,75
Oncosomes
Oncosomes, a distinct subtype of EVs, were first described by Rak's group in 2008 as large vesicles shed from glioma cells.12 These abnormally large EVs, ranging from 1–10 μm in diameter, are characterized by their aberrant cargo, including oncogenic proteins.2
They are non-apoptotic blebs originating from the plasma membrane of invasive cancer cells. The loss of the cytoskeletal regulator diaphanous-related formin-3 (DIAPH3) promotes the creation and release of the oncosomes. Subsequently, this causes a shift in the cells' phenotype from mesenchymal to a more invasive, fast, and metastatic phenotype called “the amoeboid phenotype”. The formation of these oncosomes also requires various proteins such as GTPase RhoA or its effector ROCK.12,54 Additionally, it has been reported that oncosomes can be released and formed by activating EGFR and AKT pathways (Fig. 1).2
In fact, only malignant cells produce a detectable quantity of lipo-oligosaccharide (LOs), which appears to correlate with tumor aggressiveness. Conversely, the production of LOs by benign cells is insignificant. Many tumor forms, such as those of the prostate, breast, bladder, lung cancer, and others, have the trait of tier shedding.76 They play a big role in the development, growth, and metastasis of tumors as a result of oncogenic protein complexes and molecules being overexpressed and exported between tumor cells and stroma through the oncosomes.55
Similar to other EVs, oncosomes possess a lipid bilayer encapsulating a diverse cargo of proteins, lipids, and nucleic acids as summarized in Table 1.54,76 However, they exhibit distinct compositional features, including elevated levels of phosphatidylserine and cholesterol compared to the parental cell membrane.12,54 Moreover, oncosomes are enriched in specific proteins, such as ARF6, CK18, GAPDH, MMPs, annexins, and oncogenic protein complexes.41,54,56 Notably, these large EVs like oncosomes carry substantial amounts of extracellular DNA, distinguishing them from smaller EVs subtypes such as exosomes.57
Miscellaneous types
There are other types and classifications of EVs. For example, autophagic EVs, produced from the autophagy process are involved in the degradation of cellular components, contributing to cellular homeostasis.77 Another type is stressomes (called damaged EVs) which emerge in response to cellular damage or stress, serving as messengers during challenging conditions.78 Additionally, matrix vesicles, migrasomes, and others are recognized as distinct types of EVs.77
EVs as drug delivery vehicles
EVs have emerged as promising platforms for drug delivery due to their intrinsic properties.79 Possessing low toxicity, excellent biocompatibility, and inherent targeting capabilities, EVs offer significant advantages over traditional synthetic carriers.80 Their ability to encapsulate a diverse array of therapeutic cargos, including nucleic acids, proteins, and small molecules, further enhances their potential as drug delivery vehicles.81 To harness the full therapeutic potential of EVs, meticulous preparation is essential.80,82 The development of EVs as drug delivery vehicles requires a systematic approach encompassing three key stages: EVs isolation, cargo loading and EVs engineering that will be discussed in the coming sections.
Isolation of EVs
EVs are heterogeneous populations as described earlier that are characterized by their size, density, and surface composition. To isolate these nanosized particles, scientists employ a variety of techniques, each with its own advantages and limitations.83 Conventional methods include: ultracentrifugation, membrane filtration, chromatography and microfluidics as an emerging promising approach, as microfluidics enables high-throughput EVs isolation with potential for automation.84 The choice of isolation technique depends on factors such as the desired EVs purity, sample volume, available resources, and downstream applications. It is often necessary to combine multiple methods to achieve optimal EVs isolation and characterization.83
Size-based methods.
Size-exclusion chromatography (SEC).
SEC separates EVs based on their size by utilizing a porous matrix column. Larger EVs elute first, while smaller particles are retained within the column.87 This technique preserves EV biophysical properties, offering advantages over ultracentrifugation as shown in Table 2. According to a study performed by Carso et al., EVs may be reasonably purified to 70–80% purity levels using a commercially available blind-elute-SEC, which is dependable and scalable.98 Accordingly, challenges such as low recovery rates and potential contamination with larger particles may arise (Fig. 3).
Table 2 Isolation methods of extracellular vesicles (EVs)
Principle of separation |
Isolation method |
Assay principle |
Advantages |
Disadvantages |
References |
Density |
Ultracentrifugation |
Several centrifugation steps |
Easy, very common |
Costly, time-consuming, and less efficient with viscous body fluids |
85
|
Density gradient centrifugation |
Pre-loaded centrifugal tubes, with different densities |
Useful in isolating low-density EVs |
Costly, time-consuming |
88 and 89 |
Size |
Size-exclusion chromatography |
Chromatography columns packed with porous beads |
Precise separation, maintain the structure of EVs |
Time-consuming |
87
|
Polymer-based precipitation |
Polymers decrease the solubility of EVs |
Simple, easy, inexpensive, has mild effects on EVs |
Co-precipitation of the polymer during separation |
90 and 91 |
Filtration |
Ultrafiltration porous membranes |
Eliminates small molecules, can sort out large and small EVs, is fast, more effective and has a higher recovery rate than centrifugation |
EVs can clog the membrane, and releasing them jeopardizes their integrity |
92 and 93 |
Immune-affinity |
ELISA |
Antibodies on microplates and EVs' antigens |
Very specific, selective, can be used to identify the EVs |
Not applicable for larger volumes, activity and function of EVs may be lost |
94
|
Magneto-immunoprecipitation |
Antibodies on magnetic beads and EVs' antigens |
Very specific, selective, has an increased surface area |
Aptamer-based method |
Oligonucleotide aptamers and EVs' proteins |
Easier to use, aptamers cost less and have higher stability than antibodies |
Less specific than antibodies |
95
|
Lipid-based probe method |
Lipid probes and EVs' lipids |
Can quickly isolate and retrieve highly pure EVs |
Low specificity |
82
|
Others |
Electrophoretic isolation |
Using electric field |
Specific, quick |
Inaccurate due to the presence of contaminants |
96
|
Microfluidics |
Based on physical and biological features |
Fast, efficient, small initial volumes |
Low isolation capacity, needs high technical expertise |
97
|
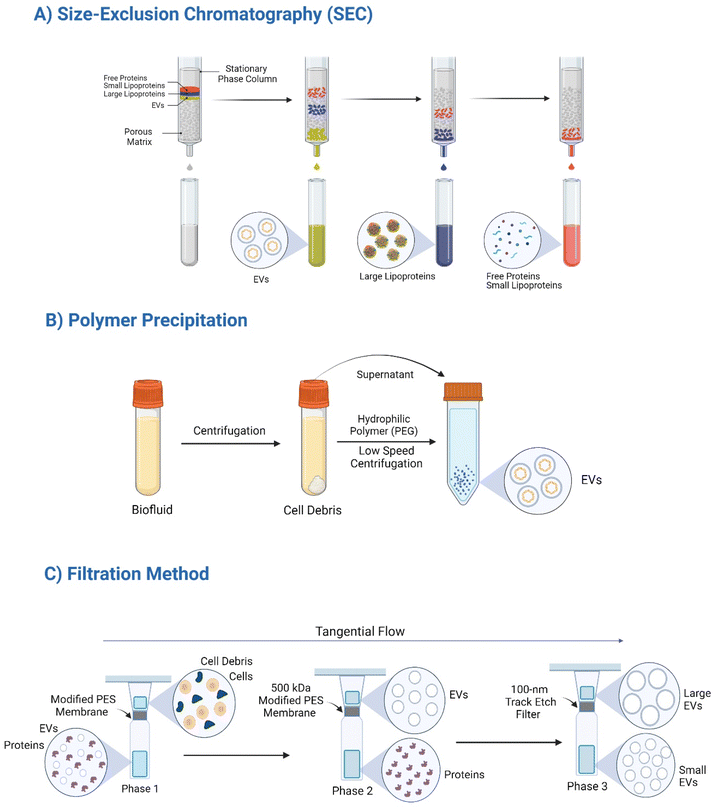 |
| Fig. 3 Isolation of extracellular vesicles using size-based methods (A) size exclusion chromatography using columns packed with a porous matrix to retain smaller particles, (B) polymer precipitation where EVs are consequently precipitated at low-speed centrifugation, (C) filtration method employing several filtration membranes. | |
Polymer precipitation.
Polymer precipitation involves the addition of a polymer, such as polyethylene glycol (PEG), to induce EVs aggregation and subsequent precipitation (Fig. 3). PEG was employed to separate EVs from cell culture media quickly and inexpensively. A PEG/dextran aqueous two-phase system was also used, with a recovery efficiency of about 70%; four times higher than ultracentrifugation.90,91 This method is rapid and cost-effective but often results in low purity due to co-precipitation of contaminants as summarized in Table 2.
Filtration.
Filtration is a highly facile and efficient method for separating EVs based on their size.82 Filtration leverages pore-sized membranes to retain EVs, offering a relatively fast and scalable approach.99 Sequential filtration using membranes with decreasing pore sizes can enhance EVs purity.92 To separate tiny EVs from bigger EVs, a 100 nm track etch filter is employed in the third phase (Fig. 3),92 and a filtration-based chip was also employed to increase the recovery rate.93 The filtration-based techniques for EVs isolation are faster, more effective, automated, and exhibit superior recovery rates compared to ultracentrifugation. However, membrane clogging and potential EVs damage are still limitations of this technique as shown in Table 2.82 Collectively, each isolation method exhibits distinct advantages and disadvantages as summarized in Table 2. The optimal choice of isolation method depends on the specific research question, desired EVs purity, and available resources. In many cases, a combination of techniques is employed to achieve optimal EVs isolation and characterization.
Affinity-based and immunoaffinity approach.
Affinity-based and immunoaffinity capture methods leverage the specific recognition between target molecules and ligands to isolate EVs as previously reviewed in ref. 100. Antibodies, aptamers, and lipid-based probes are commonly employed to bind to specific surface antigens on EVs, enabling their capture and enrichment as briefly presented below.
Enzyme-linked immunosorbent assay (ELISA).
ELISA is a commonly employed affinity-based method for EVs capture and quantification.79 This technique involves immobilizing antibodies specific to EVs surface antigens on a microplate. When an EVs sample is added, antigen–antibody interactions facilitate EVs capture onto the plate surface.94 Subsequent steps involve washing to remove unbound components and the use of a labeled secondary antibody to detect and quantify the captured EVs (Fig. 4). While ELISA offers a relatively simple approach for EVs detection, it suffers from limitations. The technique is often restricted to small sample volumes and may compromise EV integrity due to the immobilization process, potentially affecting their biological activity.101
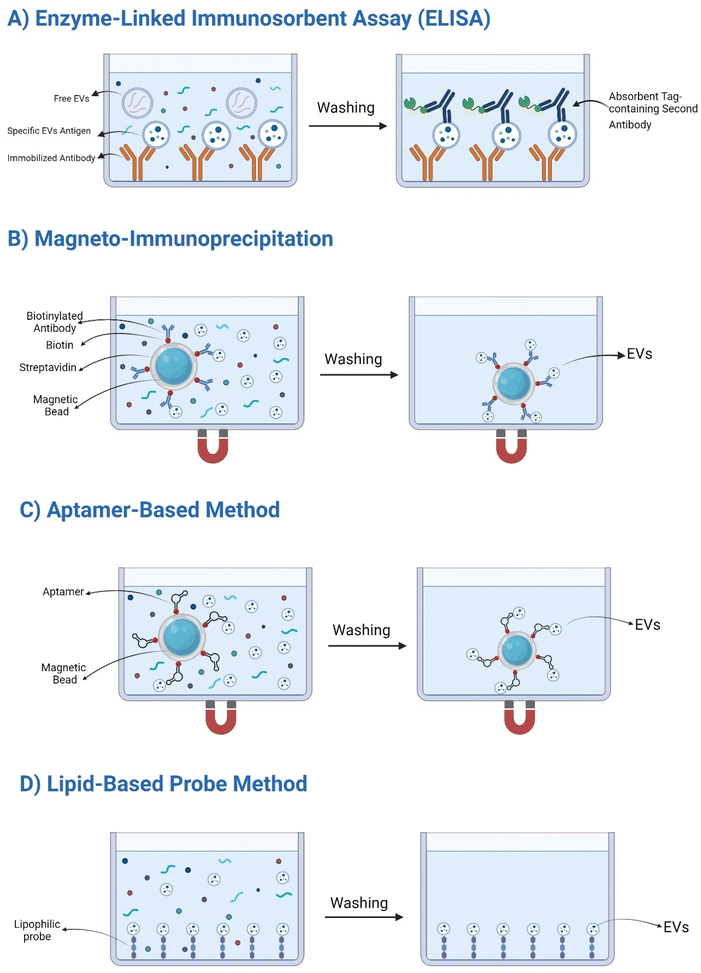 |
| Fig. 4 Affinity-based and immunoaffinity approach methods (A) enzyme-linked immunosorbent assay (ELISA) where antibodies are used to first capture antigen-expressing EVs then tag the immobilized EVs, (B) magneto-immunoprecipitation using biotinylated antibodies on streptavidin magnetic beads, (C) aptamer-based method to bind specific protein-expressing EVs, (D) lipid-based probe method exploiting EVs' lipid membrane. | |
Magneto-immunoprecipitation.
Magneto-immunocapture is an alternative affinity-based method for EVs isolation.97 This approach involves the use of magnetic beads coated with antibodies specific to EVs surface antigens. Upon incubation with the sample, EVs bind to the antibody-coated beads, forming magnetic complexes.102 These complexes can then be readily isolated using a magnetic field, allowing for efficient recovery of the target EVs (Fig. 4).
Compared to ELISA, magneto-immunocapture offers advantages in terms of sample volume and potential for higher capture efficiency due to the increased surface area of the magnetic beads as summarized in Table 2. However, similar to ELISA, this method relies on the specificity of the antibodies and may be susceptible to non-specific binding.103
Aptamer-based method.
Aptamers, synthetic oligonucleotides selected for their high affinity and specificity to target molecules, offer a promising alternative to antibody-based EVs isolation.104 Unlike antibodies, aptamers possess several advantages, including ease of handling, low production costs, and superior stability as summarized in Table 2. By targeting specific protein markers on the EVs surface, aptamers enable selective capture and enrichment of EVs subpopulations (Fig. 4). However, the development of aptamers with high affinity and specificity for EVs antigens remains a challenge, limiting their widespread application.95 Yet, despite this limitation, aptamer-based EVs isolation holds significant potential for advancing EVs research and clinical applications.
Lipid-based probe method.
Exploiting the lipidic nature of EVs membranes, lipid-based probes offer a unique approach to EVs isolation.105 These probes, designed to integrate into the EVs lipid bilayer, enable rapid and efficient EVs capture. Unlike antibody-based methods, which rely on specific surface antigens, lipid probes offer a more universal approach, capable of capturing a broader range of EVs subpopulations (Fig. 4). This innovative technique provides several advantages, including high capture efficiency, speed, and the potential to preserve EVs integrity. However, the development of lipid probes with optimal selectivity and specificity remains an ongoing challenge as shown in Table 2. Collectively, it is important to note that no single isolation method is universally superior. The choice of technique often depends on the specific research question, desired EVs purity, and available resources.
Other methodologies.
Electrophoretic isolation.
Electrophoretic methods exploit the inherent charge of EVs to achieve their separation.106 One approach involves the use of membrane filters, where an electric field is applied to expel proteins while retaining EVs.82,107 However, this method suffers from limitations in terms of specificity and recovery as shown in Table 2.
To address these challenges, tunable alternating current electrohydrodynamic (ac-EHD) technology has emerged. This method, also known as nanoshearing, utilizes a microfluidic system to generate high shear forces that disrupt EVs aggregates and enable their capture.82,96 While promising, the presence of contaminants such as cell debris remains a hurdle for achieving high-purity EVs isolates (Table 2).
Microfluidics.
Microfluidic platforms offer precise control over fluid flow and particle manipulation, making them attractive for EVs isolation.108 These systems can incorporate various physical forces, including acoustic, electrophoretic, and hydrodynamic forces, to separate EVs based on size, charge, and other properties as shown in Table 2. Microfluidic devices often demonstrate high efficiency and rapid processing times, but challenges related to sample loading, clogging, and integration with downstream analysis persist.109
Cargo loading strategies
EVs serve as versatile carriers for a diverse array of therapeutic agents, including chemotherapeutics, nucleic acids (mRNA, miRNA, siRNA, snoRNA), and proteins.110 Cargo loading strategies can be categorized into pre-isolation and post-isolation approaches as shown in Table 3. Optimizing cargo loading strategies is crucial for developing effective EV-based therapeutics.118 Cargo loading phase might be divided into two main approaches: pre-isolation loading and post-isolation loading. Pre-isolation loading is also sub-divided into several loading strategies such as endogenous loading during EVs biogenesis, cellular components, including drugs and biomolecules, can be naturally incorporated into the forming EVs.114 On the other hand, transfection-based loading where donor cells are transfected with the desired cargo, allowing for its incorporation into newly formed EVs can also be another pre-loading strategy as previously reviewed in ref. 111 and summarized in Table 3.
Table 3 Cargo-loading strategies
Isolation strategy |
Method |
Nature of cargo |
Advantages |
Disadvantages |
References |
Pre-isolation method |
Transfection of donor cells |
Proteins, peptides, nucleic acids |
High repeatability, stability, simple |
Limited transfection efficiency, strong reliance on cell viability, potential toxicity, time-consuming |
5 and 80 |
Co-incubation |
Drugs, nanoparticles |
Very simple |
Modest loading efficiency, applicable only for drugs that readily cross the plasma membrane, depends on parent cell type and drug concentration gradients |
111
|
Post-isolation method |
Electroporation |
Nucleic acids, protein, peptides, drugs, nanomaterials |
High loading efficiency |
Require optimization, cause cargo aggregation |
112
|
Sonication |
Drugs, proteins, nanoparticles |
High loading efficiency |
Cause aggregation of cargo and EVs, may damage EVs, not suitable for nucleic acids |
113
|
Incubation of EVs |
Nucleic acids, protein, peptides, drugs, nanomaterials |
Easy, practical |
Low efficiency, applicable only for drugs that readily cross the plasma membrane |
114
|
Extrusion |
Nucleic acids, protein, peptides, drugs, nanoparticles |
High loading efficiency |
Causes membrane integrity damage, makes EVs more cytotoxic |
115
|
Saponin as a surfactant |
Proteins, peptides nanoparticles |
High loading efficiency |
Concentration must be regulated, requires further purification, and risk of hemolysis |
116 and 117 |
Freezing and thawing |
Proteins, peptides |
Simple, easy |
Low efficiency of loading and low potency of the cargo, can cause vesicle degradation |
116
|
While the post-isolation loading approach are sub-categorized into passive loading which includes hydrophobic drugs that can spontaneously integrate into the lipid bilayer of isolated EVs. However, this method is limited by cargo hydrophobicity and loading efficiency.112 While the other category is known as active loading that enhance cargo loading, various techniques are employed, including electroporation, incubation, sonication, freeze–thaw cycles, and saponin-assisted incubation as shown in Table 3. These methods temporarily increase EVs membrane permeability, facilitating cargo entry.119 Yet, the choice of loading method depends on the physicochemical properties of the cargo, desired loading efficiency, and potential impact on EVs integrity.
Modifications and engineering of EVs
Modification and engineering strategies for enhancing the distribution efficiency, targeting capability, and therapeutic efficacy of EVs involve various approaches. These include targeting ligands, stimuli-responsive elements, immune evasion properties as presented in Table 4, as well as hybridized membrane modifications and other modifications. These strategies have been shown to have promising applications not only in general EVs research but also in the specific context of cancer treatment.80,120
Table 4 Engineering strategies of extracellular vesicles (EVs)
Engineering strategy |
Type |
Principle |
Pros and cons |
References |
Direct method (EVs modification) |
Lipid insertion |
Lipids and lipid-tagged molecules are hydrophobically inserted by mixing and incubation |
Quick, highly efficient, does not affect EV morphology or biological properties |
116
|
Chemical ligation (click chemistry) |
Requires the presence of reactive groups in EV lipids/proteins that can interact with reactive fragment-tagged peptides |
Reliable; change the characteristics of EVs, disrupt protein–protein interactions, costly |
120
|
Affinity binding |
Molecules that have an affinity to EVs lipids/proteins are mixed and incubated with EVs |
Does not affect the structure of the EV membrane; not as reliable as chemical or covalent bonding-based techniques |
121
|
Enzyme ligation |
Enzymatic ligation between the targeted protein/peptide and the EV membrane protein |
Produces permanent covalent modification without the need for chemical or genetic modification |
122
|
Hybridization |
Combination of EVs with other lipid nanovesicles like liposomes |
Improved colloidal stability, increased half-life, low immunogenicity; loss of biological functions of integral EVs |
123
|
Biomimetic EV production |
Synthetic EV components that possess homing capabilities and cargo delivery effectiveness |
Better pharmacokinetic and biocompatibility properties; require extra purification steps |
Indirect method (producing cell modification) |
Genetic manipulation of the producing cells |
Transgene production of proteins or chimeric proteins |
Dual-targeting ability, low immunogenicity, low systemic toxicity; some peptides require protection against degradation |
69
|
Metabolic labeling |
Cultivating donor cells in a medium containing saccharides or amino acids bearing reactive groups attaches them to EV membrane proteins |
Reliable, effective; expensive |
73
|
41
|
76
|
EVs can be modified through two primary methods: direct and indirect. The EVs themselves are modified in the direct method, while in the indirect method, modifications are made to the EV-producing cells.121 It is important to note that no single optimum approach or standardized strategy exists for altering and modifying EVs. Each technique has its own benefits and applications as summarized in Table 4.121
Characterization of the isolated EVs and their cargos
Following isolation, comprehensive characterization of EVs is essential to validate their identity, purity, and biological relevance.124 A combination of techniques is often employed to assess various EVs properties, as discussed in the following section.
Charaterization of EVs
Nanoparticle tracking analysis (NTA).
NTA is a widely adopted technique for characterizing EVs based on their brownian motion.125,126 This method provides information on particle size distribution, concentration, and enables direct visualization of individual EVs in solution. NTA's ability to analyze particles within a size range of 10–1000 nm makes it particularly suitable for EVs characterization.125
Despite its advantages, NTA has certain limitations. The accuracy of size determination can be influenced by factors such as particle shape, refractive index, and the presence of contaminants.127 Additionally, differentiating EVs from other nanoparticles in complex biological samples can be challenging, necessitating careful sample preparation and data analysis.127 Accordingly, in an attempt to enhance the specificity of NTA, fluorescence-based approaches have been developed.128,129 Labeling EVs with specific markers makes it possible to differentiate target EVs from non-EVs particles as shown in Table 5 and as recently reviewed in ref. 130. However, this method is limited by fluorophore bleaching and the availability of suitable antibodies or probes.129,131 Overall, NTA is a valuable tool for EVs characterization but should be complemented with other techniques to comprehensively understand EVs properties.
Table 5 Comparison between nanoparticle tracking analysis (NTA) and dynamic light scattering (DLS)
Point of comparison |
Nanoparticle tracking analysis (NTA) |
Dynamic light scattering (DLS) |
Principle |
Characterizing EVs based on their brownian motion |
Type of detection |
Size, concentration and distribution of EVs |
Size of the EVs |
Advantages |
Ability to analyze particles within a size range of 10–1000 nm |
Rapid |
Can detect relatively low concentartions |
Sensitive |
Can measure EVs (1 nm–6 μm) without needing pre-treatment |
Disadvantages |
Limited size detaction |
Accurate size detection is limited to monodispersed samples |
Differentiating EVs from other nanoparticles in complex biological samples can be challenging |
Susceptible to interference from larger particles and contaminants |
Dynamic light scattering (DLS).
DLS is another commonly used technique for EVs characterization.129,132 By measuring the fluctuations in light scattered by particles in suspension, DLS can determine particle size distribution based on Brownian motion.129 While DLS analysis is rapid and sensitive and can measure EVs (1 nm–6 μm) without needing pre-treatment, the results are only consistently accurate for monodisperse samples as shown in Table 5.133 Moreover, DLS is susceptible to interference from larger particles and contaminants, which can affect the accuracy of size distribution measurements, especially in complex biological samples.133 Therefore, the use of this approach for the investigation of varied heterogonous EVs populations is limited as previously reviewed in 134.
Transmission electron microscopy (TEM).
TEM is the gold standard for visualizing individual EVs.135 Its high resolution, capable of resolving particles smaller than 1 nm, allows for detailed morphological characterization of EVs size, shape, and internal structure.136 When combined with immunogold labeling, TEM enables the localization of specific proteins or other biomolecules within EVs, providing insights into their composition and function.137 However, TEM is associated with several limitations. The sample preparation process, which involves dehydration and embedding in resin, can introduce artifacts and distort EVs' true size and shape.138 Additionally, the high vacuum environment required for imaging can lead to sample damage and the formation of cup-shaped structures, complicating accurate size measurements.139 Despite these challenges, TEM remains an indispensable tool for obtaining high-resolution images of EVs, providing valuable information for their characterization and classification.70
Atomic force microscopy (AFM).
Atomic force microscopy (AFM) offers high-resolution imaging of EVs at the nanoscale.129 By scanning the sample surface with a sharp tip mounted on a cantilever, AFM generates detailed topographical images without the need for sample staining or fixation.140 This technique enables precise measurement of EVs size and shape distribution, providing valuable insights into EVs heterogeneity.141 Moreover, by functionalizing the AFM tip with specific antibodies or ligands, it is possible to differentiate EVs subpopulations based on surface markers.140,142 AFM offers versatility through various imaging modes, including contact, tapping, non-contact, and peak force modes, allowing for tailored analysis of EVs properties.141,142 However, factors such as tip condition, applied force, and environmental conditions can influence image quality and data accuracy.129,143 To address these challenges, meticulous sample preparation and instrument calibration are essential for obtaining reliable AFM data. Yet, AFM remains a powerful tool for characterizing EVs morphology and physical properties.
Resistive pulse sensing (RPS).
Resistive pulse sensing (RPS) uses the Coulter concept to assess the particles distribution and diameters in suspensions that roughly range between 50 and 100
000 nm.127,144 The RPS used in the field of EVs characterization is often performed with qNano (Izon Science Ltd, Christchurch, New Zealand). Two fluid cells divided by a non-conductive membrane make up the qNano.125,145 A single membrane pore is subjected to an electric current, and when particles flow through it, the signal experiences a transitory attenuation roughly proportionate to the volume of particles. The sample quantities used in the qNano can be as little as 10 μL.125 Nevertheless, there are numerous drawbacks to this method, such as: the need for various pore sizes; pores are prone to blockage; minimal phenotypic information on the EV's origins is obtained; and it is impossible to distinguish EVs from pollutants of comparable sizes.125
Flow cytometry (FCM).
FCM is another powerful technique for analyzing particle populations in suspension, including EVs.146 By measuring light scatter and fluorescence signals from individual particles, FCM provides information about particle size, complexity, and surface markers.129 Conventional FCM relies on forward scatter (FSC) to estimate particle size and side scatter (SSC) to assess particle complexity. However, the lower detection limit of conventional FCM is around 300 nm, limiting its ability to accurately characterize smaller EVs.129,147 To overcome such limitation, labeling strategies, such as coupling EVs with larger fluorescent beads, have been employed to enhance detection sensitivity.148 Additionally, nanoscale flow cytometry (nFCM) has emerged as a promising technique for analyzing particles as small as 100 nm.132,149 By combining high-resolution optics with sensitive detectors, nFCM enables more accurate characterization of EVs size and surface markers, facilitating in-depth studies of EVs heterogeneity.129,150 Despite these advancements, challenges remain in accurately differentiating EVs from other nanoparticles and achieving high-throughput analysis of large sample volumes. Further developments in flow cytometry instrumentation and sample preparation are necessary to fully realize the potential of this technique for EVs research.
Enzyme-linked immunosorbent assay (ELISA).
ELISA is also a widely employed technique for quantifying and characterizing EVs.129,151 By capturing EVs through specific antibody–antigen interactions on a microplate surface, ELISA detects and measures EV-associated proteins. This method offers several advantages, including high sensitivity, specificity, and the potential for high-throughput analysis. However, ELISA is susceptible to variations in assay conditions, leading to potential inconsistencies in results.151
Zeta potential (ZP).
ZP is mainly concerned by the electrostatic repulsion among particles in colloidal solution.152 ZP can be affected by the surface charge, which can be assessed from the electrophoretic mobility in the colliodal system.153 The net surface charge of EVs,as shown by the ZP, is crucial in determining the stability of EVs.153,154 Higher absolute ZP values suggest that the colloid, containg EVs, is more stable.155 ZP can be conjugated with other characterization techniques including DLS (like zetasizer), NTA (like zetaview or Z-NTA), RPS, or by on-chip microcapillary electrophoresis combined with micrsocopy technique for visualization and identification of EVs.152,156,157
Characterization of EV content
EVs protein content characterization.
Proteins found in EVs might give information about biological activities and how they affect cell communication. As a result, several studies characterized EVs from the perspective of their protein content.124
Two simple approaches tare employed to quantify the total protein level, which are the Coomassie Brilliant Blue G-250 test and the bicinchoninic acid assay.48 Both rely on measuring the absorbance of a colored complex formed between a protein and a reagent, using a calibration curve with known protein concentrations to quantify the protein.158 While these tests are often employed, their applicability is restricted to assessing extremely pure EVs samples since protein impurities impair measurement accuracy.159
Western blot is another technique used for detecting, quantifying, and characterizing EVs protein, giving insight into EVs biology and discovering pathophysiological indicators of illnesses.160,161 Nevertheless, EVs are diverse s, thus it is challenging to provide a universal EV protein identifier or marker.162 As a result, the International Society for EVs suggests characterizing various transmembrane and cytosolic proteins found in EVs,163 following a set of guidelines (Minimal Information for Studies of EVs; MISEV2018) that should be followed for isolation, characterization, and functional studies of Evs. In this regards, there should be at least one cytosolic protein (such as TSG101 (tumour susceptibility gene 10), ALIX (ALG-2-interacting protein X), and syntenin) and one transmembrane protein (such as CD9, CD63, and CD81).48 This approach has been used to detect and characterize proteins of EVs produced by tumors. For instance, Yoshioka et al. observed that regardless of the EV origin, all examined EVs recovered from four human prostate cell lines were positive for CD9 and CD81, with equal quantity. Conversely, the detection of other EV marker proteins, such as TSG101 and CD63, was uneven due to the heterogeneity of the cancer.164
Moreoever, surface plasmon resonance (SPR) is another promising technique for detecting proteins in various EVs.165 SPR allows for the extremely sensitive label-free detection of EVs by their immunological capture to a SPR-active surface, such as silver or gold nanoparticles.166 Several of these methods have recently been used to quantify and characterize EVs produced from diseases, including cancer, using particular protein markers. For instance, it has been demonstrated that gold nanoparticles stabilized with DNA aptamers against particular surface proteins cause a noticeable color shift because EVs attach to these aptamers specifically.167 This method enables a multiplexed analysis of EVs' protein composition using visual and spectrophotometric methods.167
The field of EVs has significantly benefited from mass spectrometry proteomics technologies, which have made it possible to create extensive protein profiles of EVs.124,168 Proteomics has been utilized in a number of studies to measure the presence of specific peptides and find changes in the EVs of biological samples from cancer patients compared to healthy people.169 Notably, one study has shown that EVs generated from the serum of breast cancer patients have different protein profiles according to the cell line they derived from and so can be used to distinguish between the molecular subtypes of breast cancer, such as triple-negative or HER2 subtypes.170
Fluorescence-based techniques offer a versatile approach for detecting and quantifying EVs.171. Fluorescently labeled antibodies targeting EV surface markers, such as CD9, CD63, and CD81, can be used to visualize and track EVs.148 For instance, quenching and relabeling techniques have been employed to identify unique EVs marker clusters.172 Integerated microfluidic-based approaches have also emerged as powerful tools for EVs isolation and quantification. ExoDEP chips, for example, utilize antibody-functionalized beads to capture EVs, followed by electrochemical detection.173 Thermophoresis-assisted fluorescence detection is another promising method that enables the isolation and quantification of EVs based on size and specific markers.174,175 The authors developed a novel approach called HOLMES-ExoPD-L1, which combines tumor-associated PD-L1 aptamers with thermophoresis to detect circulating PD-L1-positive EVs with high sensitivity and specificity, achieving an impressive limit of detection of 17.6 pg ml−1.174
EVs RNA content characterization.
The presence of RNA (mRNAs, miRNAs, long noncoding RNAs and/or others) inside EVs is an essential tool in confirming the successful synthesis/isolation of Evs.176,177 Their identification and characterization are crucial because they can provide information about the biological activities of EVs, their cell origin, and how they alter cell communication in both normal and pathological conditions.124
One of the widely used characterization assays for Evs' RNA profile is quantitative reverse transcriptase-PCR (qRT-PCR).178,179 Several studies employed qRT-PCR to quantify and characterize the RNA content of EVs in malignancies, including pancreatic cancer,180 breast cancer,181 endometrial cancer,182 and many other cancers.183 However, it has a major limitation: the low RNA yield, particularly from clinical samples, which may restrict the identification and analysis.184
Digital droplet PCR (ddPCR) is a relatively recent technique that enables the absolute measurement of gene expression. This technology enables the susceptible measurement of RNA expression levels and DNA variations without standard curves.125 Recently, ddPCR was used to analyze plasma-derived exosomal RNA from prostate cancer patients,185 and EVs produced from blood and CSF of glioma patients.186
Furthermore, next-generation sequencing (NGS) has been used to characterize the RNA content of EVs from various sources, allowing for a more complete examination of the EV-RNA repertoire.187,188 Many research have employed NGS to characterize EVs' RNA content in malignancies including bladder cancer,189 ovarian cancer,190 colorectal cancer,191 pancreatic cancer,192 and many malignancies. However, it suffers from some limitations, including, library preparation concerns and adapter dimers that could hinder the analysis.187
EVs lipid content characterization.
Lipids are an essentialcomponent of EVs as they contain markers from their original cell and act as a protective barrier for their load.193 They are also involved in the transportation of biomolecules and membrane fusion events.193 Mass spectrometry-based lipidomics approaches are among the most widely utilized methods for characterizing and quantifying the lipid composition of EVs. Moreover, these methodologies provide information regarding the lipid profile of EVs from different malignancies.194,195
For instance, using an MS-based lipomic approach, it was discovered that EVs derived from high metastatic breast cancer had a different lipid profile than EVs from low metastatic breast cancer.196 It was also discovered that the EVs from high metastatic breast cancer had more unsaturated diacylglycerols (DGs) than those from low metastatic breast cancer, which means they have a greater capacity to promote angiogenesis.196 Another study discovered that whereas sterol lipids, sphingolipids, and glycerophospholipids were more highly abundant in EVs from tumorigenic and metastatic prostate cancer cells, fatty acids, glycerolipids, and prenol lipids were more highly abundant in EVs from non-tumourigenic prostate cancer cells.197
EVs metabolic content characterization.
Metabolites, such as steroid hormones, amino acids, or metabolic intermediates of lipid and nutrients, are a class of tiny molecules that result from different biological events.198 Two primary analytical methods are used to characterize metabolites in EVs: nuclear magnetic resonance (NMR) spectroscopy and high-resolution mass spectrometry.199,200 Although on the scarcity of information regarding EVs' metabolome, several studies highlighted the relevance of EVs as carriers of key metabolome fingerprints that may be utilized to define particular changes in cellular homeostasis that occur in both physiological and pathological conditions.201,202 For instance, Čuperlović-Culf et al. (2020) studied the metabolome of sEVs generated from several glioblastoma cells using NMR spectroscopy.203 When comparing EVs from different glioblastoma subtypes, there was a noticeable variation in their metabolic characteristics.203 Another study that used MSbased methods found that the urine EVs from prostate cancer and benign prostatic hyperplasia had different metabolic profiles, with around 76 compounds different.199 It was also detected that the steroid hormone, 3 beta-hydroxyandros-5-en-17-one-3-sulphate is higher in prostate cancer than in benign prostate hyperplasia so urine EVs can be used as a non-invasive biomarker for prostate cancer.199 Furthermore, another study examined the metabolomic profile of EVs produced from pancreatic cancer cells (PANC-1) cultivated at various oxygen concentrations, as hypoxia contributes to the malignant activity of these cells.200 This study also found that the metabolite composition of EVs differed depending on the cell of origin. A total of 140 hydrophilic metabolites were discovered in small EVs, and it was revealed that the metabolomic profile of small EVs altered during hypoxic stress, with an increase in the metabolites implicated in angiogenesis, growth, and metastasis of cancer200
EVs: a novel frontier in cancer therapeutics
Cancer remains a formidable global health challenge, with conventional treatments often yielding suboptimal outcomes.20,64,65 The emergence of EVs as versatile nanoparticulate carriers has sparked significant interest in their potential as therapeutic agents.70 Unlike traditional drug delivery systems, EVs possess intrinsic advantages such as biocompatibility, low immunogenicity, and the ability to cross biological barriers.79
EVs can be harnessed for delivering a wide range of therapeutic payloads, including chemotherapeutics,68 nucleic acids,81,204 and immunomodulatory molecules.61 Their capacity to target specific tissues and cells, coupled with their ability to evade immune clearance, positions EVs as promising candidates for overcoming challenges associated with conventional drug delivery systems.120,121
To fully realize the potential of EVs in cancer therapeutics, meticulous engineering and optimization are imperative. Researchers can develop highly effective EV-based therapeutics by carefully selecting cargo molecules, modifying EVs surface properties, and understanding the complex interactions within the tumor microenvironment. This review highlights the significant potential of EVs as a platform for delivering cancer treatments and emphasizes the need for continued research to overcome existing challenges and translate these promising findings into clinical applications.
EVs as drug delivery vehicles for chemotherapeutic agents
Conventional chemotherapy regimens often exhibit limited efficacy due to systemic toxicity and drug resistance.63,66 EVs have emerged as promising platforms for delivering chemotherapeutic agents to cancer cells, addressing these limitations.205 By encapsulating chemotherapeutic drugs within their lipid bilayer, EVs can enhance drug delivery vehicles to tumor sites, prolong drug circulation time, and reduce off-target effects.120
Several studies have demonstrated the potential of EV-based chemotherapy.206 For instance, LipHA-modified EVs loaded with doxorubicin (DOX) have shown enhanced efficacy in overcoming drug resistance in breast cancer cells by inhibiting P-glycoprotein.120,207 Similarly, CC8-modified EV-like vesicles carrying Imperialine have demonstrated anti-tumor activity in non-small cell lung cancer (NSCLC).208 Furthermore, a research study demonstrated that exosomes that are produced from human fibrosarcoma cells are able to carry DOX, and have the potential to target fibrosarcoma efficiently, increasing therapeutic retention and inhibiting cancer growth (Fig. 5).120,209 It has also been reported that mimic or chimeric exosomes derived from red blood cells (RBCs) carrying DOX can enhance drug accumulation, decrease drug clearance, and prevent or reduce the growth of breast cancer cells.120,210
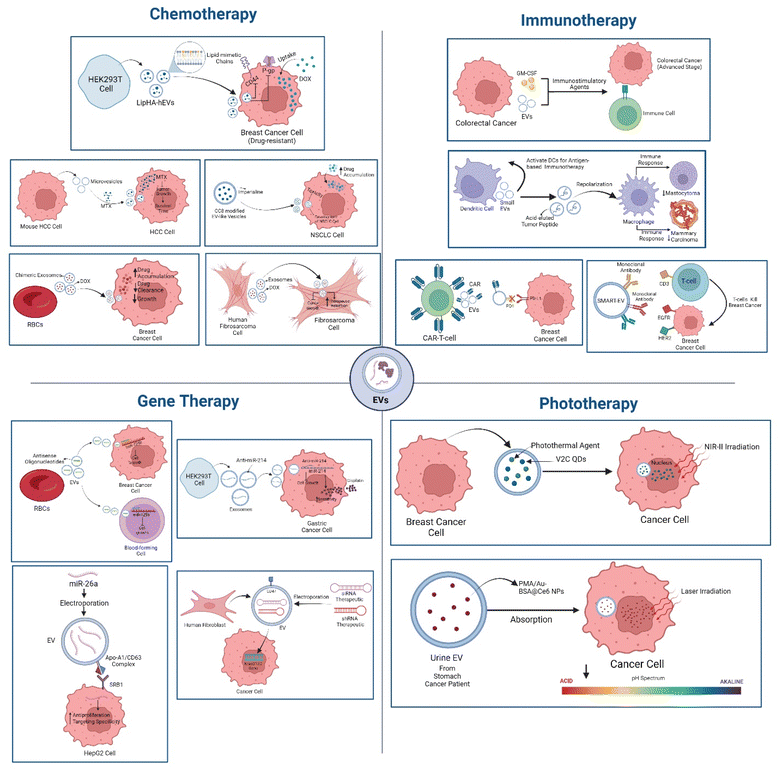 |
| Fig. 5 Multifunctional role of extracellular vesicles in cancer therapeutics. | |
Moreover, EVs derived from tumor cells or healthy cells, such as red blood cells, have been employed to deliver chemotherapeutic agents like methotrexate, cisplatin, and paclitaxel, resulting in improved therapeutic outcomes211–213(Fig. 5 and Table 6). Collectively, these findings highlight the versatility of EVs as drug-delivery vehicles and their potential to improve cancer treatment by overcoming challenges associated with conventional chemotherapy.
Table 6 Overview of EV-based cancer therapeutics
Methods |
Source |
EVs type |
Drug/Cargo |
Treated tumor |
Outcomes |
Ref. |
Monotherapy/targeted/combined chemotherapy |
Mouse HCC cells |
Microvesicles |
MTX |
HCC |
Inhibited tumor growth without side effects |
120
|
RBCs |
Mimitcs exosomes |
DOX |
Breast cancer |
↑ Drug accumulation, ↓ clearance of drug, inhibited cancer growth |
121
|
Hek293t Cells |
Lipha-modified EVs |
DOX |
MDR breast cancer |
↑ Drug accumulation and drug sensitivity, prevented cancer growth |
268
|
Plasma |
CC8 modified EV-like vesicles |
Imperialine |
NSCLC |
↑ Drug accumulation, inhibited tumor growth with reduced systemic toxicity |
269
|
Mouse macrophages |
Exosomes |
PTX |
Lung metastatic cancer |
↑ Cytotoxicity, prevented lung metastasis |
209
|
Mouse macrophages |
Exosomes mimitics |
DM4 |
Lung metastatic breast cacner |
Prevented lung cancer metastasis |
210
|
— |
Folate-engineered microvesicles |
Bcl-2 sirna and paclitaxel |
Breast cancer squamous cell carcinoma |
Significantly ↑ synergistic antitumor efficacy of chemotherapy and gene therapy |
235
|
Hek293t cells |
HER2-binding affibody, LAMP2, and GFP modified exosomes |
FU and mir-21 inhibitor oligonucleotide |
Colon cancer |
Improved chemosensitivity and inhibited growth of colon cancer |
236
|
Human macrophages |
RAG-modified exosomes |
DOX and gold nanorods |
Cervical cancer |
Promote drug release and inhibit cervical cancer growth |
237
|
Monotherapy/targeted/combined immuno-therapy |
Colorectal cancer cells |
EVs |
Granulocyte-macrophage colony-stimulating factor |
Colorectal cancer |
Utilized as immunostimulatory agents to treat patients with advanced CRC |
120
|
Mature dendritic cells |
EVs |
Loaded with acid-eluted tumour peptide |
Mastocytoma and mammary carcinoma |
Stimulate DCs for tumor antigen-based cancer immunotherapy, prevention of tumor growth |
121
|
CAR-T-cell |
CAR-T-cell-derived EVs with surface-expressed CAR |
— |
Breast cancer |
Directly target tumor cells, safe, do not express programmed cell death protein 1 (PD1) |
210
|
Expi293F cells |
Exosomes modified with CD3 and EGFR antibodies |
CD3 and EGFR antibodies |
Breast cancer |
Exhibit strong anti-cancer immunity against EGFR-positive breast cancer cells |
221
|
Expi293F cells |
Exosomes modified with CD3 and HER2 antibodies |
CD3 and HER2 antibodies |
Breast cancer |
Redirected the T cells to kill HER2-positive breast cancer cells |
230
|
Tumor cells |
Irradiated tumor cell-released microvesicles |
— |
Malignant pleural effusion |
Repolarized tumor-associated macrophages, resulted in immunogenic death |
270
|
Mouse melanoma cells |
Exosomes modified with cpg DNA |
SAV-LA fusion protein |
Melanoma |
Prevented tumor growth |
231
|
BM-MSCs (bone marrow mesenchymal stem cells) |
Exosomes modified with oxaliplatin |
Galectin-9 siRNAs and surface modified with oxaliplatin |
Pancreatic ductal adenocarcinoma (PDAC) |
Improved tumor targeting and enhanced drug accumulation in cancer site |
233
|
CT26 cells |
Hybrid-modified exosomes fused with liposomes |
ICG, immune adjuvant R837, overexpressed CD47 |
Colorectal cancer |
Reduced tumors in tumor-bearing mice |
232
|
NK cells |
Exosomes |
Mirna, such as let 7a |
Breast cancer and NB cells |
Target tumors effectively and prevent their growth |
238
|
239
|
240
|
Monotherapy/targeted/combined gene-therapy |
RBCs |
EVs |
miR-125b ASOs |
Breast cancer and acute myelocytic leukemia |
Inhibit tumor growth without observable cytotoxicity |
120
|
HEK293T cells |
Exosomes |
Anti-mir-214 |
Cisplatin-resistant gastric cancer |
Enhanced chemosensitivity and prevented tumor growth |
221
|
HEK293T cells |
SMA or EGFR aptamer, folate modified EV |
Survivin siRNA |
Prostate, breast, and colorectal cancer |
Prevented tumor growth |
218
|
Breast cancer |
EVs |
miR-134 |
Breast cancer |
Reduced migration and invasion of cancer cells, and enhanced sensitivity to anti-Hsp90 drugs |
220
|
HEK 293T cells |
EVs decorated with the Apo-A1/CD63 |
miR-26a |
Liver cancer |
It has a strong antiproliferative impact and great targeting specificity |
271
|
Human fibroblast |
EVs enriched in CD47 |
siRNA or shRNA against KrasG12D |
Pancreatic cancer cell |
Suppressed tumor development and ↑ survival rate |
241
|
Brain metastatic cancer |
Apoptotic bodies (sabs) |
Anti-TNF-α antisense oligonucleotide (ASO) paired with cationic konjac glucomannan (ckgm) |
— |
Extremely high brain-delivery efficiency due to the CD44v6 expressed on the apoptotic bodies helping them cross the BBB |
Mouse M1 macrophages |
QDs modified exosomes |
DOX and miR21- responded hairpin DNA |
Breast cancer |
Inhibited growth of the cancer |
Monotherapy/targeted/combined photo-therapy |
Urine from gastric cancer patients |
Exosomes |
PMA/Au-BSA@Ce6 nanovehicle |
Gastric cancer |
Enhanced penetration and retention, prevented growth |
120
|
Breast cancer cells |
RGD-modified exosomes |
TAT peptide-modified V2C QDs |
Breast cancer |
Penetrate the nucleus and perform low-temperature PTT with increased antitumor activity |
226
|
Mouse macrophages |
NRP-1 targeted peptide-modified exosomes |
3-Curcumin and SPIONs |
Orthotopic glioma |
Give decent results for imaging and therapy, penetrate the BBB, and prevent tumor growth |
227
|
Mouse HCC cells |
Mps (microvesicles) |
DOX and Bi2Se3 nanodots |
HCC |
Prevented and inhibited cancer growth |
239
|
Blood |
Exosomes with chimeric peptides produced |
Photosensitizers and nuclear translocation peptide |
Breast cancer |
Destroyed the membrane and the nucleus of breast cancer cells in mouse models |
272
|
EVs as drug delivery vehicles for gene therapy
EVs have emerged as promising platforms for delivering nucleic acid-based therapeutics, such as siRNA, shRNA, and miRNA, to target specific genes involved in cancer progression.214,215 By encapsulating these therapeutic molecules within EVs, it is possible to overcome challenges associated with traditional gene delivery methods, such as limited cellular uptake and rapid degradation.216,217
Several studies have demonstrated the efficacy of EV-based gene therapy for cancer treatment. For example, EVs loaded with anti-miR-214 have shown anti-tumor effects in gastric cancer models.218 A notable example is the use of RBC-derived EVs loaded with antisense oligonucleotides targeting miR-125b, which demonstrated anti-tumor effects in breast cancer and acute myeloid leukemia without inducing systemic toxicity219 (Fig. 5). In parallel, EVs decorated with the Apo-A1/CD63 complex, a known target of scavenger receptor class B type 1, were electroporated directly with miR-26a, which is downregulated in liver cancer. When applied to HepG2 liver cancer cells, the miR-26a enriched EVs had a strong antiproliferative impact and excellent targeting specificity (Fig. 5).220,221
It is also worth noting that EVs express CD47, a ‘don't eat me’ signal that protects them from immune clearance.222 This immune evasion property, combined with their ability to deliver therapeutic cargos, makes EVs attractive for cancer therapy. Studies have shown that CD47-enriched EVs can efficiently deliver siRNA or shRNA targeting oncogenic KrasG12D, leading to tumor growth inhibition and improved survival in preclinical models.223 This highlights the potential of EVs to overcome immune barriers and deliver therapeutic payloads directly to cancer cells. Collectively, These findings highlight the potential of EVs to deliver nucleic acid-based therapeutics with high specificity and efficacy, offering a promising approach for treating various types of cancer as shown in Table 6.
EVs as promising platforms for phototherapy
EVs have shown promise as carriers for phototherapeutic agents.224 By encapsulating photosensitizers or photothermal agents within their lipid bilayer, EVs can deliver these compounds to tumor sites, enhancing their therapeutic efficacy.225 For instance, EVs loaded with vanadium carbide quantum dots have demonstrated effective low-temperature photothermal therapy,226 while EVs carrying multifunctionalized nanoparticles have shown improved tumor accumulation and ROS generation upon laser irradiation225,226 (Fig. 5). These approaches leverage the unique properties of EVs, such as biocompatibility and prolonged circulation time, to enhance the therapeutic potential of phototherapy as presented in Table 6.
In another study, high-purity urine EVs were collected from stomach cancer patients and electroporated with multi-functionalized PMA/Au-BSA@Ce6 NPs. Due to the decreased macrophage endocytosis and longer blood retention time, the modified nanovehicles are effectively absorbed into cancer cells. The designed nanovehicles are shattered and release enormous NPs within in response to laser irradiation and acidic conditions (Fig. 5). Consequently, a significant amount of singlet oxygen is released, thereby limiting tumor cell proliferation.227
Novel EV-based immunotherapeutic strategies
Immunotherapy has shown to be a viable cancer treatment option, frequently exhibiting promising results when utilized in conjunction with conventional approaches such as radiation, chemotherapy, or surgery.16,228,229 A growing number of studies have shown that EVs impact immunological processes, including immune response activation, antigen presentation modification, and tumor microenvironment modulation.221,230
Studies have demonstrated the potential of EVs derived from immune cells, such as dendritic cells (DCs), to stimulate anti-tumor immunity.221 For instance, GM-CSF-loaded EVs derived from colorectal cancer (CRC) cells have shown efficacy in treating advanced CRC patients.171
In addition to utilizing immune cell-derived EVs, engineering EVs to express specific targeting and effector functions has been explored. CAR-T cell-derived EVs, expressing chimeric antigen receptors (CARs), have demonstrated the ability to directly target and kill tumor cells while evading immune checkpoint inhibition (Fig. 5).120,231 Similarly, synthetic antibody-targeted EVs (SMART-EVs) have been developed to redirect T cells towards cancer cells expressing specific antigens, such as EGFR and HER2.120,232 Nonetheless, by genetically expressing two different types of antibodies on the EV membrane, monoclonal antibodies specific for T cell CD3 and cancer cell-associated EGFR, synthetic antibody-targeted EVs (SMART-EVs) were created and exhibited strong anti-cancer immunity against EGFR-positive breast cancer cells.120,233
These studies highlight the versatility of EVs for immunotherapy and their potential to overcome challenges associated with traditional immune-based therapies. Table 6 provides a comprehensive overview of different EV-based immunotherapy strategies and their corresponding outcomes.
EVs in combination therapies: synergistic approaches
Combining EVs with other therapeutic modalities holds the potential to enhance treatment efficacy and overcome limitations associated with single-agent therapies.234 This approach, often referred to as combination therapy, aims to achieve synergistic effects and reduce the risk of drug resistance. By combining chemotherapy, immunotherapy, gene therapy, and phototherapy, EVs has emerged as promising platforms for delivering multiple therapeutic payloads and thus producing synergistic effects, enhance treatment efficacy, and improve patient outcomes.
EVs in chemotherapy-related combined therapies
EVs loaded with chemotherapeutic agents can be combined with other therapeutic cargos, such as siRNA or miRNA, to create multifunctional platforms.121 For example, folate-engineered microvesicles loaded with Bcl-2 siRNA and paclitaxel demonstrated enhanced anti-tumor efficacy compared to single-agent therapies by targeting both apoptotic pathways and chemotherapy resistance.235 Similarly, HER2-binding affibody-modified exosomes carrying 5-FU and miR-21 inhibitors have shown promising results in inhibiting colon cancer growth.120,236
Additionally, chemotherapy can be combined with phototherapy, such as RAG modified exosomes produced from human macrophages. These exosomes were loaded with DOX and gold nanorods as a source of hyperthermia. NIR irradiation was utilized to influence the permeability of the EVs membrane, promoting medication release, and limiting tumor recurrence in a controlled manner. This combination therapy inhibited cervical cancer growth120,237 as shown in Table 6.
EVs in immunotherapy-related combination therapy
EVs can be effectively combined with other therapeutic modalities to enhance anti-tumor responses and overcome treatment resistance.121 For example, EVs loaded with galectin-9 siRNA and conjugated with oxaliplatin have demonstrated synergistic effects in treating pancreatic ductal adenocarcinoma (PDAC) by combining immunotherapy and chemotherapy.121,238 This approach promotes tumor-suppressive macrophage polarization, recruits cytotoxic T lymphocytes, and induces immunogenic cell death (ICD), leading to improved tumor control.
Similarly, combining phototherapy (PDT or PTT) with EV-based therapies can enhance anti-tumor effects by inducing ICD and creating an immunogenic tumor microenvironment.8 Additionally, hybrid nanovesicles can be produced by mixing and fusing liposomes loaded with the photothermal agent ICG and immune adjuvant R837, along with exosomes with overexpressed CD47. This hybrid nanovesicle effectively reduced tumors in tumor-bearing mice by competitively connecting with SIRP alpha, prior to tumor cells, resulting in increased tumor cell phagocytosis by macrophages and to avoid immune clearance of the hybrid. They are also considered as an example of combining cancer immunotherapy with PTT.62,121,239
Furthermore, EVs can be used to deliver gene therapy cargos in combination with other therapeutic modalities as summarized in Table 6. Moreover, in an immunogenic mutli-modality approach, self-assembly of biomimetic core–shell NPs featuring a dendrimer core loaded with therapeutic miRNA, such as let-7a, a hydrophilic shell of NK-cell-derived EVs, showed highly effective targeting and therapeutic miRNA delivery to both neuroblastoma cells and breast cancer cells. This approach resulted in dual tumor growth inhibition effects.120,240 These findings highlight the potential of EVs to serve as versatile platforms for developing combination therapies with enhanced efficacy and reduced side effects.
EVs in gene therapy-related and phototherapy-related combination therapies
As shown in Table 6, it has been repeatedly reported that immunomodulatory EVs or photo-sensitive EVs are usually combined with immunotherapeutic and chemotherapeutic agents.9,10 It has been reported that the utilization of small apoptotic bodies (sABs) derived from brain metastatic cancer cells, loaded with anti-TNF-alpha antisense oligonucleotide and paired with cationic konjac glucomannan (cKGM), exhibits remarkable brain delivery efficiency. This is attributed to the presence of CD44v6 expressed on the apoptotic bodies, facilitating their crossing of the blood–brain barrier.241 Another study, which employed phototherapy combined with gene therapy approach, showed that exosomes with chimeric peptide produced from blood loaded with photosensitizers and nuclear translocation peptide destroyed the membrane and the nucleus of breast cancer cells in a mouse model.242 Collectively, these strategies elaborated in Table 6 demonstrate the versatility of EVs as platforms for developing complex therapeutic approaches. However, further research is needed to optimize EVs production, cargo loading, and delivery for clinical translation.
Challenges and obstacles with EVs-based cancer treatment
Despite the promising potential of EVs as therapeutic carriers, several challenges hinder their clinical translation.243 Ensuring consistent EVs quality and composition for reliable therapeutic outcomes has been considered one of the formidable barriers for the clinical translation of EVs-based onco-therapeutics.
EVs heterogeneity
A significant hurdle in the development of EV-based therapeutics is the inherent heterogeneity of EVs populations.244,245 EVs derived from the same cell type can exhibit variations in size, composition, and biological functions due to factors such as cellular origin, activation state, and extracellular environment.243,244
For instance, miRNAs, a common cargo of EVs as highlighted, exhibit differential distribution among EVs subpopulations.246 This heterogeneity is attributed to both passive and active loading mechanisms, with some miRNAs being enriched in specific EVs subtypes.247,248 According to earlier research, miRNAs in EVs are a loaded by two mechanisms into the EVs: (a) highly expressed cellular miRNAs that enter the EVs passively by an osmotic-like effect; or (b) selectively released miRNAs that actively pack into EVs according to the particular RNA molecule sequence.243,249,250 For instance, Pigati et al. discovered that, dependent on the amount of cytoplasmic miRNA, around 66% of the released miRNAs are passively secreted by EVs, whereas 30% of exosomal miRNAs do not match the cellular profile, indicating that they are released selectively.251
Similarly, protein composition can vary significantly among EVs subpopulations,252–254 as demonstrated by the identification of distinct protein profiles in low-density and high-density EVs derived from the same cell line (B16F10 melanoma cells). Accordingly, the authors have classified LD (low density)-Exo and HD (high density)-Exo.254 They found that both EVs contain the same proteins such as TSG101 and Alix. In addition to distinct protein species, LD-Exo has two unique proteins: cyclin Y and actinin alpha 4. In contrast, HD-Exo tightly encloses ephrin type-A receptor 2. Additionally, they found that there was some variation in the relative abundance of the some of common proteins. It has been partly explained that such protein-heterogeneity of EVs is related to the fact that EVs relies on ESCRT-dependent or -independent sorting machinery in sorting and packing some molecules including proteins.4,243 Communally, the heterogeneity of EVs poses challenges for isolating specific EVs subpopulations with desired properties and for understanding their precise biological functions. To address this issue, advanced characterization techniques and novel isolation methods are required.
Cargo loading efficiency
Optimizing the loading of multiple therapeutic agents into EVs without compromising their biophysical properties is a significant challenge.255 Therapeutic payloads of interest can be loaded into EVs in a variety of methods as explained earlier. However, EVs loading efficiency is comparatively lower than liposome loading efficiency for instance.255,256 There may not be enough room for exogenous medications to be loaded into EVs because EVs themselves retain some of the contents of their parent cells during creation.255,257 As such, loading of exogenous medicines into EVs is a significant obstacle.255,258
Since multiple techniques were employed to load onco-therapeutic agents into the same EVs as previously described, the techniques could be precisely compared.255 A comprehensive literature review was performed to identify and compare different loading strategies. By categorizing these methods into high, medium, and low loading efficiency, researchers can select the most appropriate approach based on the specific cargo and desired outcome. However, literature has been highly controversial at that point. Haney et al. discovered, for instance, that the loading amount of catalase into EVs was raised in the following order: incubation, freeze/thaw cycle, sonication, extrusion.259 According to Kim et al. there was an increase in the amount of PTX (paclitaxel) loaded into exosomes in the following order: incubation, electroporation, sonication.255,260 Another study, when compared to incubation, electroporation, and extrusion, it was revealed that the amount of loading pharmaceuticals of saponin or hypotonic dialysis was up to 11 times higher.115,255 However, the loading efficiency of EVs depends not only on loading techniques but also on the chemical composition of the EVs, the drug content, and lipophilicity.255 Therefore, further work is required to create novel loading strategies in future studies and to optimize existing loading technologies.255
Stability and storage
Preserving the integrity and bioactivity of EVs during storage is crucial for their therapeutic application.261 While low temperatures, typically −80 °C, are commonly employed, factors such as storage buffer, cryoprotectants, and lyophilization can influence EVs stability.243,262
Although EVs were typically resuspended in phosphate buffer saline.46 Yet, it has been reported that Trehalose has great advantage as a cryoprotectant, enhancing EVs stability during freezing and thawing cycles.263,264 Lyophilization, another potential storage method, can reduce the need for ultra-low temperature storage, although its impact on EVs integrity requires further investigation.265 Charoenviriyakul et al. reported that after lyophilization, the sample was held at room temperature and trehalose was added as a cryoprotectant to shield the exosomes from osmotic destruction. The outcomes demonstrate that lyophilization has minimal impact on the physical and biological properties of exosomes.265 Optimizing EVs storage conditions is essential for maintaining their therapeutic potential and ensuring consistency in downstream applications.
Lack of standardized isolation and purification method for EVs
A significant hurdle in advancing EV-based therapeutics is the lack of a standardized isolation method.255 The heterogeneity of EVs populations, coupled with the diverse range of isolation techniques available, has hindered the development of reproducible and reliable EVs preparations.
To address the challenges associated with EVs isolation, various methods have been developed, each with its own strengths and limitations. These methods can be categorized based on their recovery and specificity: (1) high recovery, low specificity: ultracentrifugation, filtration, and precipitation techniques are commonly used due to their simplicity and relatively high yield, but they often result in low purity EVs preparations.266,267 (2) Intermediate specificity and recovery: size-exclusion chromatography (SEC) offers improved purity compared to ultracentrifugation, but with lower recovery rates. (3) High specificity, low recovery: immunoaffinity capture and microfluidic-based techniques provide high purity but often suffer from low yield and limited throughput.266,267 The ideal EVs isolation method would combine high recovery, specificity, efficiency, and reproducibility. However, no single method currently meets all these criteria.
To overcome these challenges, the development of standardized protocols, advanced characterization techniques, and innovative isolation methods is essential.
Conclusion
In conclusion, EVs have emerged as versatile platforms with substantial potential to revolutionize cancer therapy. This review provides a thorough overview of EVs, emphasizing their promise as innovative drug delivery vehicles for cancer therapeutics. The authors highlight different types of EVs, isolation techniques, and different characterization methods. Moreover, the authors spot the light onto the journey and the transformation process of EVs from intracellular trafficking molecules to fully fortified drug delivery vehicles and especially focused on cancer therapeutics. The promising ability of EVs to carry diverse cargos has also been highlighted, including chemotherapeutic agents, nucleic acids, immunomodulatory molecules, and photosensitizers thus offering unique advantages over conventional therapeutic modalities.
EVs exhibit heterogeneity in terms of size, composition, and biological function, necessitating the development of standardized isolation and characterization methods. While challenges remain in optimizing EVs production, cargo loading, and delivery, the potential benefits of EV-based therapies warrant continued research and development.
By addressing the limitations associated with EVs heterogeneity and developing innovative strategies for EVs engineering and combination therapies, the field can progress towards the clinical translation of this promising technology. Ultimately, the successful development of EV-based therapeutics holds the potential to improve patient outcomes and transform cancer treatment.
Data availability
This manuscript does not involve any experimental work.
Conflicts of interest
There are no conflicts to declare.
Acknowledgements
The authors acknowledge the financial support and sponsorship received from the Alexander von Humboldt Foundation, Germany.
References
- L. M. Doyle and M. Z. Wang, Overview of extracellular vesicles, their origin, composition, purpose, and methods for exosome isolation and analysis, Cells, 2019, 8(7), 727 CrossRef CAS PubMed.
- R. Jaiswal and L. M. Sedger, Intercellular vesicular transfer by exosomes, microparticles and oncosomes-implications for cancer biology and treatments, Front. Oncol., 2019, 9, 125 CrossRef PubMed.
- B. L. Deatherage and B. T. Cookson, Membrane vesicle release in bacteria, eukaryotes, and archaea: a conserved yet underappreciated aspect of microbial life, Infect. Immun., 2012, 80(6), 1948–1957 CrossRef CAS PubMed.
- G. Van Niel, G. d'Angelo and G. Raposo, Shedding light on the cell biology of extracellular vesicles, Nat. Rev. Mol. Cell Biol., 2018, 19(4), 213–228 CrossRef CAS PubMed.
- Z. Wang,
et al., Extracellular vesicles as an emerging drug delivery system for cancer treatment: Current strategies and recent advances, Biomed. Pharmacother., 2022, 153, 113480 CrossRef CAS PubMed.
- M. P. Zaborowski,
et al., Extracellular vesicles: composition, biological relevance, and methods of study, Bioscience, 2015, 65(8), 783–797 CrossRef PubMed.
- R. A. Youness,
et al., Oral Delivery of Psoralidin by Mucoadhesive Surface-Modified Bilosomes Showed Boosted Apoptotic and Necrotic Effects against Breast and Lung Cancer Cells, Polymers, 2023, 15(6), 1464 CrossRef CAS PubMed.
- A. Ramzy,
et al., Drugless nanoparticles tune-up an array of intertwined pathways contributing to immune checkpoint signaling and metabolic reprogramming in triple-negative breast cancer, Biomed. Mater., 2022, 18(1) DOI:10.1088/1748-605X/aca85d.
- R. A. Youness,
et al., A Snapshot of Photoresponsive Liposomes in Cancer Chemotherapy and Immunotherapy: Opportunities and Challenges, ACS Omega, 2023, 8(47), 44424–44436 CrossRef CAS PubMed.
- N. K. Sedky,
et al., Box-Behnken design of thermo-responsive nano-liposomes loaded with a platinum(iv) anticancer complex: evaluation of cytotoxicity and apoptotic pathways in triple negative breast cancer cells, Nanoscale Adv., 2023, 5(19), 5399–5413 RSC.
- D. K. Jeppesen,
et al., Reassessment of exosome composition, Cell, 2019, 177(2), 428–445 CrossRef CAS PubMed.
-
V. R. Minciacchi, M. R. Freeman, and D. Di Vizio, Extracellular vesicles in cancer: exosomes, microvesicles and the emerging role of large oncosomes, in Seminars in Cell & Developmental Biology, Elsevier, 2015 Search PubMed.
- F. T. Borges, L. Reis and N. Schor, Extracellular vesicles: structure, function, and potential clinical uses in renal diseases, Braz. J. Med. Biol. Res., 2013, 46, 824–830 CrossRef CAS PubMed.
- T. Huyan,
et al., Extracellular vesicles–advanced nanocarriers in cancer therapy: Progress and achievements, Int. J. Nanomed., 2020, 6485–6502 CrossRef CAS PubMed.
- Y. A. ZeinElAbdeen, A. AbdAlSeed and R. A. Youness, Decoding Insulin-Like Growth Factor Signaling Pathway From a Non-coding RNAs Perspective: A Step Towards Precision Oncology in Breast Cancer, J. Mammary Gland Biol. Neoplasia, 2022, 27(1), 79–99 CrossRef PubMed.
- R. A. Youness,
et al., Contradicting interplay between insulin-like growth factor-1 and miR-486-5p in primary NK cells and hepatoma cell lines with a contemporary inhibitory impact on HCC tumor progression, Growth Factors, 2016, 34(3–4), 128–140 CrossRef CAS PubMed.
- Y. M. Shaalan,
et al., Destabilizing the interplay between miR-1275 and IGF2BPs by Tamarix articulata and quercetin in hepatocellular carcinoma, Nat. Prod. Res., 2018, 32(18), 2217–2220 CrossRef CAS PubMed.
- N. A. Selem,
et al., Let-7a/cMyc/CCAT1/miR-17–5p Circuit Re-sensitizes Atezolizumab Resistance in Triple Negative Breast Cancer through Modulating PD-L1, Pathol., Res. Pract., 2023, 248, 154579 CrossRef CAS PubMed.
- R. M. Abdallah,
et al., Hindering the synchronization between miR-486-5p and H19 lncRNA by hesperetin halts breast cancer aggressiveness through tuning ICAM-1, Anti Cancer Agents Med. Chem., 2022, 22(3), 586–595 CrossRef CAS PubMed.
- R. A. Youness,
et al., A novel role of sONE/NOS3/NO signaling cascade in mediating hydrogen sulphide bilateral effects on triple negative breast cancer progression, Nitric Oxide, 2018, 80, 12–23 CrossRef CAS PubMed.
- N. A. Selem, R. A. Youness and M. Z. Gad, What is beyond LncRNAs in breast cancer: A special focus on colon cancer-associated Transcript-1 (CCAT-1), Non-coding RNA Res., 2021, 6(4), 174–186 CrossRef CAS PubMed.
- R. Y. Mekky,
et al., MALAT-1: Immunomodulatory lncRNA hampering the innate and the adaptive immune arms in triple negative breast cancer, Transl. Oncol., 2023, 31, 101653 CrossRef CAS PubMed.
- A. Dawoud,
et al., Circular RNAs: New layer of complexity evading breast cancer heterogeneity, Non-coding RNA Res., 2023, 8(1), 60–74 CrossRef CAS PubMed.
- S. A. Fahmy,
et al., Molecular Engines, Therapeutic Targets, and Challenges in Pediatric Brain Tumors: A Special Emphasis on Hydrogen Sulfide and RNA-Based Nano-Delivery, Cancers, 2022, 14(21), 5244 CrossRef CAS PubMed.
- T. Abaza,
et al., Emerging Role of Circular RNAs in Hepatocellular Carcinoma Immunotherapy, Int. J. Mol. Sci., 2023, 24(22), 16484 CrossRef CAS PubMed.
-
S. E. Wang, Extracellular vesicles in cancer therapy, in Seminars in Cancer Biology, Elsevier, 2022 Search PubMed.
- N. M. Elemam,
et al., Expression of GPR68, an Acid-Sensing Orphan G Protein-Coupled Receptor, Breast Cancer Front. Oncol., 2022, 12, 847543 CrossRef CAS PubMed.
- N. M. Elemam,
et al., Editorial: Understanding the crosstalk between immune cells and the tumor microenvironment in cancer and its implications for immunotherapy, Front. Med., 2023, 10, 1202581 CrossRef PubMed.
- A. Becker,
et al., Extracellular vesicles in cancer: cell-to-cell mediators of metastasis, Cancer cell, 2016, 30(6), 836–848 CrossRef CAS PubMed.
- A. R. Chin and S. E. Wang, Cancer tills the premetastatic field: mechanistic basis and clinical implications, Clin. Cancer Res., 2016, 22(15), 3725–3733 CrossRef CAS PubMed.
- S. E. Wang, Extracellular vesicles and metastasis, Cold Spring Harb. Perspect. Med., 2020, 10(7), a037275 CrossRef CAS PubMed.
- Y. Zhang and X.-F. Wang, A niche role for cancer exosomes in metastasis, Nat. Cell Biol., 2015, 17(6), 709–711 CrossRef CAS PubMed.
- N. I. Rizk,
et al., Revealing the role of serum exosomal novel long non-coding RNA NAMPT-AS as a promising diagnostic/prognostic biomarker in colorectal cancer patients, Life Sci., 2024, 352, 122850 CrossRef CAS PubMed.
- Y. K. Abd El Fattah,
et al., CCDC144NL-AS1/hsa-miR-143-3p/HMGA2 interaction: In-silico and clinically implicated in CRC progression, correlated to tumor stage and size in case-controlled study; step toward ncRNA precision, Int. J. Biol. Macromol., 2023, 253, 126739 CrossRef CAS PubMed.
- T. A. Shtam,
et al., Exosomes are natural carriers of exogenous siRNA to human cells in vitro, Cell Commun. Signal., 2013, 11(1), 1–10 CrossRef PubMed.
- M. Bakhti, C. Winter and M. Simons, Inhibition of myelin membrane sheath formation by oligodendrocyte-derived exosome-like vesicles, J. Biol. Chem., 2011, 286(1), 787–796 CrossRef CAS PubMed.
- S. Wang,
et al., Synapsin I is an oligomannose-carrying glycoprotein, acts as an oligomannose-binding lectin, and promotes neurite outgrowth and neuronal survival when released via glia-derived exosomes, J. Neurosci., 2011, 31(20), 7275–7290 CrossRef CAS PubMed.
- E. R. Abels and X. O. Breakefield, Introduction to extracellular vesicles: biogenesis, RNA cargo selection, content, release, and uptake, Cell. Mol. Neurobiol., 2016, 36, 301–312 CrossRef CAS PubMed.
- L. A. Mulcahy, R. C. Pink and D. R. F. Carter, Routes and mechanisms of extracellular vesicle uptake, J. Extracell. Vesicles, 2014, 3(1), 24641 CrossRef PubMed.
- R. A. Haraszti,
et al., High-resolution proteomic and lipidomic analysis of exosomes and microvesicles from different cell sources, J. Extracell. Vesicles, 2016, 5(1), 32570 CrossRef PubMed.
- C. N. Suire and M. D. Hade, Extracellular vesicles in type 1 diabetes: A versatile tool, Bioengineering, 2022, 9(3), 105 CrossRef CAS PubMed.
- G. Raposo and W. Stoorvogel, Extracellular vesicles: exosomes, microvesicles, and friends, J. Cell Biol., 2013, 200(4), 373–383 CrossRef CAS PubMed.
- M. P. Bebelman,
et al., Biogenesis and function of extracellular vesicles in cancer, Pharmacol. Therapeut., 2018, 188, 1–11 CrossRef CAS PubMed.
- W. Möbius,
et al., Immunoelectron microscopic localization of cholesterol using biotinylated and non-cytolytic perfringolysin O, J. Histochem. Cytochem., 2002, 50(1), 43–55 CrossRef PubMed.
- B. J. Tauro,
et al., Comparison of ultracentrifugation, density gradient separation, and immunoaffinity capture methods for isolating human colon cancer cell line LIM1863-derived exosomes, Methods, 2012, 56(2), 293–304 CrossRef CAS PubMed.
- K. W. Witwer,
et al., Standardization of sample collection, isolation and analysis methods in extracellular vesicle research, J. Extracell. Vesicles, 2013, 2(1), 20360 CrossRef PubMed.
- J. C. Akers,
et al., Biogenesis of extracellular vesicles (EV): exosomes, microvesicles, retrovirus-like vesicles, and apoptotic bodies, J. Neuro Oncol., 2013, 113, 1–11 CrossRef PubMed.
- C. Théry,
et al., Minimal information for studies of extracellular vesicles 2018 (MISEV2018): a position statement of the International Society for Extracellular Vesicles and update of the
MISEV2014 guidelines, J. Extracell. Vesicles, 2018, 7(1), 1535750 CrossRef PubMed.
- H. Cai, K. Reinisch and S. Ferro-Novick, Coats, tethers, Rabs, and SNAREs work together to mediate the intracellular destination of a transport vesicle, Dev. Cell, 2007, 12(5), 671–682 CrossRef CAS PubMed.
- C. Tricarico, J. Clancy and C. D'Souza-Schorey, Biology and biogenesis of shed microvesicles, Small GTPases, 2017, 8(4), 220–232 CrossRef CAS PubMed.
- X. Xu, Y. Lai and Z.-C. Hua, Apoptosis and apoptotic body: disease message and therapeutic target potentials, Biosci. Rep., 2019, 39(1), BSR20180992 CrossRef CAS PubMed.
-
M. Battistelli and E. Falcieri, Apoptotic bodies: particular extracellular vesicles involved in intercellular communication, Advances in Medical Biochemistry, Genomics, Physiology, and Pathology, 2021, pp. 473–486 Search PubMed.
- S. Elmore, Apoptosis: a review of programmed cell death, Toxicol. Pathol., 2007, 35(4), 495–516 CrossRef CAS PubMed.
- M. C. Cufaro,
et al., Extracellular vesicles and their potential use in monitoring cancer progression and therapy: the contribution of proteomics, J. Oncol., 2019, 2019 Search PubMed.
- V. R. Minciacchi,
et al., Large oncosomes contain distinct protein cargo and represent a separate functional class of tumor-derived extracellular vesicles, Oncotarget, 2015, 6(13), 11327 CrossRef PubMed.
- S. R. Li,
et al., Tissue-derived extracellular vesicles in cancers and non-cancer diseases: Present and future, J. Extracell. Vesicles, 2021, 10(14), e12175 CrossRef CAS PubMed.
- T. Vagner,
et al., Large extracellular vesicles carry most of the tumour DNA circulating in prostate cancer patient plasma, J. Extracell. Vesicles, 2018, 7(1), 1505403 CrossRef CAS PubMed.
- S. A. Fahmy,
et al., Emerging tendencies for the nano-delivery of gambogic acid: a promising approach in oncotherapy, RSC Adv., 2024, 14(7), 4666–4691 RSC.
- M. Dimik,
et al., The exosome: a review of current therapeutic roles and capabilities in human reproduction, Drug Delivery Transl. Res., 2023, 13(2), 473–502 CrossRef PubMed.
- T. Wollert and J. H. Hurley, Molecular mechanism of multivesicular body biogenesis by ESCRT complexes, Nature, 2010, 464(7290), 864–869 CrossRef CAS PubMed.
- N. M. Elemam,
et al., Pharmacogenomic and epigenomic approaches to untangle the enigma of IL-10 blockade in oncology, Expet Rev. Mol. Med., 2024, 26, e1 CrossRef PubMed.
- A. H. Soliman,
et al., Phytochemical-derived tumor-associated macrophage remodeling strategy using Phoenix dactylifera L. boosted photodynamic therapy in melanoma via H19/iNOS/PD-L1 axis, Photodiagnosis Photodyn. Ther., 2023, 44, 103792 CrossRef CAS PubMed.
- H. Nafea,
et al., Dual targeting of H(2)S synthesizing enzymes; cystathionine β-synthase and cystathionine γ-lyase by miR-939-5p effectively curbs triple negative breast cancer, Heliyon, 2023, 9(10), e21063 CrossRef CAS PubMed.
- A. Dawoud,
et al., Pan-inhibition of the three H(2)S synthesizing enzymes restrains tumor progression and immunosuppression in breast cancer, Cancer Cell Int., 2024, 24(1), 136 CrossRef CAS PubMed.
- R. A. Youness,
et al., Targeting hydrogen sulphide signaling in breast cancer, J. Adv. Res., 2021, 27, 177–190 CrossRef CAS PubMed.
- M. K. A. El-Aziz,
et al., Decoding hepatocarcinogenesis from a noncoding RNAs perspective, J. Cell. Physiol., 2023, 238(9), 1982–2009 CrossRef CAS PubMed.
- F. H. El Kilany,
et al., miR-744/eNOS/NO axis: A novel target to halt triple negative breast cancer progression, Breast Dis., 2021, 40(3), 161–169 CAS.
- S. A. Fahmy,
et al., Hijacking 5-Fluorouracil Chemoresistance in Triple Negative Breast Cancer via microRNAs-Loaded Chitosan Nanoparticles, Int. J. Mol. Sci., 2024, 25(4), 2070 Search PubMed.
- R. Kalluri and V. S. LeBleu, The biology, function, and biomedical applications of exosomes, Science, 2020, 367(6478), eaau6977 CrossRef CAS PubMed.
- M. A. Kumar,
et al., Extracellular vesicles as tools and targets in therapy for diseases, Signal Transduct. Targeted Ther., 2024, 9(1), 27 CrossRef PubMed.
- R. A. Youness,
et al., Heat Shock Proteins: Central Players in Oncological and Immuno-Oncological Tracks, Adv. Exp. Med. Biol., 2023, 1409, 193–203 CrossRef CAS PubMed.
- Z. Hassan,
et al., How Viruses Hijack and Modify the Secretory Transport Pathway, Cells, 2021, 10(10), 2535 CrossRef CAS PubMed.
- E. Calzada, O. Onguka and S. M. Claypool, Phosphatidylethanolamine
Metabolism in Health and Disease, Int. Rev. Cell Mol. Biol., 2016, 321, 29–88 CAS.
- K. S. Negara,
et al., The role of caspase-dependent and caspase-independent pathways of apoptosis in the premature rupture of the membranes: A case-control study, Int. J. Reprod. Biomed., 2020, 18(6), 439 Search PubMed.
- E. Petrovčíková, K. Vičíková and V. Leksa, Extracellular vesicles–biogenesis, composition, function, uptake and therapeutic applications, Biologia, 2018, 73(4), 437–448 CrossRef.
- C. Ciardiello,
et al., Focus on extracellular vesicles: new frontiers of cell-to-cell communication in cancer, Int. J. Mol. Sci., 2016, 17(2), 175 CrossRef PubMed.
- M. Sheta,
et al., Extracellular vesicles: New classification and tumor immunosuppression, Biology, 2023, 12(1), 110 CrossRef CAS PubMed.
- T. Eguchi,
et al., Cell stress induced stressome release including damaged membrane vesicles and extracellular HSP90 by prostate cancer cells, Cells, 2020, 9(3), 755 CrossRef CAS PubMed.
- R. A. Assal,
et al., A Novel Epigenetic Strategy to Concurrently Block Immune Checkpoints PD-1/PD-L1 and CD155/TIGIT in Hepatocellular Carcinoma, Transl. Oncol., 2024, 45, 101961 CrossRef CAS PubMed.
- S. Walker,
et al., Extracellular vesicle-based drug delivery systems for cancer treatment, Theranostics, 2019, 9(26), 8001 CrossRef CAS PubMed.
- H. H. Rashwan,
et al., Harnessing the supremacy of MEG3 LncRNA to defeat gastrointestinal malignancies, Pathol., Res. Pract., 2024, 256, 155223 CrossRef CAS PubMed.
- S. Tiwari,
et al., Preparation and characterization of extracellular vesicles, Am. J. Reprod. Immunol., 2021, 85(2), e13367 CrossRef CAS PubMed.
- K. Brennan,
et al., A comparison of methods for the isolation and separation of extracellular vesicles from protein and lipid particles in human serum, Sci. Rep., 2020, 10(1), 1039 CrossRef CAS PubMed.
- A. Akbar,
et al., Methodologies to Isolate and Purify Clinical Grade Extracellular Vesicles for Medical Applications, Cells, 2022, 11(2), 186 CrossRef CAS PubMed.
- M. Y. Konoshenko,
et al., Isolation of Extracellular Vesicles: General Methodologies and Latest Trends, Biomed. Res. Int., 2018, 2018, 8545347 Search PubMed.
- C. Théry,
et al., Isolation and characterization of exosomes from cell culture supernatants and biological fluids, Curr. Protoc. Cell Biol., 2006, 30(1), 1–29 Search PubMed.
- P. M. Vanderboom,
et al., A size-exclusion-based approach for purifying extracellular vesicles from human plasma, Cell Rep. Methods, 2021, 1(3), 100055 CrossRef CAS PubMed.
- L. Mincheva-Nilsson,
et al., Isolation and characterization of exosomes from cultures of tissue explants and cell lines, Curr. Protoc. Im., 2016, 115(1), 1–21 Search PubMed.
- F. Momen-Heravi,
et al., Current methods for the isolation of extracellular vesicles, Biol. Chem., 2013, 394(10), 1253–1262 CrossRef CAS PubMed.
- M. A. Rider, S. N. Hurwitz and D. G. Meckes Jr, ExtraPEG: a polyethylene glycol-based method for enrichment of extracellular vesicles, Sci. Rep., 2016, 6(1), 23978 CrossRef CAS PubMed.
- H. Shin,
et al., High-yield isolation of extracellular vesicles using aqueous two-phase system, Sci. Rep., 2015, 5(1), 13103 CrossRef CAS PubMed.
- M. L. Heinemann,
et al., Benchtop isolation and characterization of functional exosomes by sequential filtration, J. Chromatogr. A, 2014, 1371, 125–135 CrossRef CAS PubMed.
- F. Liu,
et al., The exosome total isolation chip, ACS Nano, 2017, 11(11), 10712–10723 CrossRef CAS PubMed.
- M. Logozzi,
et al., Immunocapture-based ELISA to characterize and quantify exosomes in both cell culture supernatants and body fluids, Methods Enzymol., 2020, 645, 155–180 CAS.
- D. Sun,
et al., Recent progress in aptamer-based microfluidics for the detection of circulating tumor cells and extracellular vesicles, J. Pharm. Anal., 2023, 13(4), 340–354 CrossRef PubMed.
- R. Vaidyanathan,
et al., Detecting exosomes specifically: a multiplexed device based on alternating current electrohydrodynamic induced nanoshearing, Anal. Chem., 2014, 86(22), 11125–11132 CrossRef CAS PubMed.
- J. Zhang,
et al., Exosome and exosomal microRNA: trafficking, sorting, and function, Genomics, Proteomics Bioinf., 2015, 13(1), 17–24 CrossRef CAS PubMed.
- G. Corso,
et al., Reproducible and scalable purification of extracellular vesicles using combined bind-elute and size exclusion chromatography, Sci. Rep., 2017, 7(1), 11561 CrossRef PubMed.
- V. S. Chernyshev,
et al., Asymmetric depth-filtration: A versatile and scalable method for high-yield isolation of extracellular vesicles with low contamination, J. Extracell. Vesicles, 2022, 11(8), e12256 CrossRef CAS PubMed.
- S. Cheng,
et al., Advances in microfluidic extracellular vesicle analysis for cancer diagnostics, Lab Chip, 2021, 21(17), 3219–3243 RSC.
- J. Suthar,
et al., Recent developments in biosensing methods for extracellular vesicle protein characterization, Wiley Interdiscip. Rev.:Nanomed. Nanobiotechnol., 2023, 15(1), e1839 CAS.
- L. M. Doyle and M. Z. Wang, Overview of Extracellular Vesicles, Their Origin, Composition, Purpose, and Methods for Exosome Isolation and Analysis, Cells, 2019, 8(7), 727 CrossRef CAS PubMed.
- V. C. Kok and C. C. Yu, Cancer-Derived Exosomes: Their Role in Cancer Biology and Biomarker Development, Int. J. Nanomed., 2020, 15, 8019–8036 CrossRef CAS PubMed.
- M. Domsicova,
et al., New Insights into Aptamers: An Alternative to Antibodies in the Detection of Molecular Biomarkers, Int. J. Mol. Sci., 2024, 25(13), 6833 CrossRef CAS PubMed.
- A. D. Flynn and H. Yin, Lipid-Targeting Peptide Probes for Extracellular Vesicles, J. Cell. Physiol., 2016, 231(11), 2327–2332 CrossRef CAS PubMed.
- D. Buschmann, V. Mussack and J. B. Byrd, Separation, characterization, and standardization of extracellular vesicles for drug delivery applications, Adv. Drug Deliv. Rev., 2021, 174, 348–368 CrossRef CAS PubMed.
- S. Cho,
et al., Isolation of extracellular vesicle from blood plasma using electrophoretic migration through porous membrane, Sens. Actuators, B, 2016, 233, 289–297 CrossRef CAS.
- F. G. Ortega-Sanchez,
et al., Microfluidic systems in extracellular vesicles single analysis. A systematic review, Trac. Trends Anal. Chem., 2023, 159, 116920 CrossRef CAS.
- T. A. Duncombe, A. M. Tentori and A. E. Herr, Microfluidics: reframing biological enquiry, Nat. Rev. Mol. Cell Biol., 2015, 16(9), 554–567 CrossRef CAS PubMed.
- Y. Zeinelabdeen,
et al., MIAT LncRNA: A multifunctional key player in non-oncological pathological conditions, Non-coding RNA Res., 2024, 9(2), 447–462 CrossRef CAS PubMed.
- B. S. Joshi, D. Ortiz and I. S. Zuhorn, Converting extracellular vesicles into nanomedicine: loading and unloading of cargo, Mat. Today Nano, 2021, 16, 100148 CrossRef CAS.
- A. Al-Jipouri,
et al., Liposomes or extracellular vesicles: a comprehensive comparison of both lipid bilayer vesicles for pulmonary drug delivery, Polymers, 2023, 15(2), 318 CrossRef CAS PubMed.
- A. Jeyaram,
et al., Enhanced Loading of Functional miRNA Cargo via pH Gradient Modification of Extracellular Vesicles, Mol. Ther., 2020, 28(3), 975–985 CrossRef CAS PubMed.
- Y. Han,
et al., Overview and Update on Methods for Cargo Loading into Extracellular Vesicles, Processes, 2021, 9(2), 356 CrossRef CAS PubMed.
- G. Fuhrmann,
et al., Active loading into extracellular vesicles significantly improves the cellular uptake and photodynamic effect of porphyrins, J. Contr. Release, 2015, 205, 35–44 CrossRef CAS PubMed.
- Q. Liu,
et al., Targeted therapy using engineered extracellular vesicles: principles and strategies for membrane modification, J. Nanobiotechnol., 2023, 21(1), 334 CrossRef PubMed.
- I. Podolak, A. Galanty and D. Sobolewska, Saponins as cytotoxic agents: a review, Phytochemistry Rev., 2010, 9, 425–474 CrossRef CAS PubMed.
- F. Villa, R. Quarto and R. Tasso, Extracellular Vesicles as Natural, Safe and Efficient Drug Delivery Systems, Pharmaceutics, 2019, 11(11), 557 CrossRef CAS PubMed.
- C. Chen,
et al., Active cargo loading into extracellular vesicles: Highlights the heterogeneous encapsulation behaviour, J. Extracell. Vesicles, 2021, 10(13), e12163 CrossRef CAS PubMed.
- X. Zhang,
et al., Engineered extracellular vesicles for cancer therapy, Adv. Mater., 2021, 33(14), 2005709 CrossRef CAS PubMed.
- J. Chen,
et al., Engineered extracellular vesicles: potentials in cancer combination therapy, J. Nanobiotechnol., 2022, 20(1), 132 CrossRef CAS PubMed.
- L. Ramasubramanian, P. Kumar and A. Wang, Engineering extracellular vesicles as nanotherapeutics for regenerative medicine, Biomolecules, 2019, 10(1), 48 CrossRef PubMed.
- G. Rosso and V. Cauda, Biomimicking Extracellular Vesicles with Fully Artificial Ones: A Rational Design of EV-BIOMIMETICS toward Effective Theranostic Tools in Nanomedicine, ACS Biomater. Sci.
Eng., 2022, 5924–5932 Search PubMed.
- K. P. De Sousa,
et al., Isolation and characterization of extracellular vesicles and future directions in diagnosis and therapy, Wiley Interdiscip. Rev.:Nanomed. Nanobiotechnol., 2023, 15(1), e1835 CAS.
- M. I. Ramirez,
et al., Technical challenges of working with extracellular vesicles, Nanoscale, 2018, 10(3), 881–906 RSC.
- C. Gardiner,
et al., Techniques used for the isolation and characterization of extracellular vesicles: results of a worldwide survey, J. Extracell. Vesicles, 2016, 5(1), 32945 CrossRef PubMed.
- E. Van der Pol,
et al., Particle size distribution of exosomes and microvesicles determined by transmission electron microscopy, flow cytometry, nanoparticle tracking analysis, and resistive pulse sensing, J. Thromb. Haemostasis, 2014, 12(7), 1182–1192 CrossRef CAS PubMed.
- D. Fortunato,
et al., Opportunities and pitfalls of fluorescent labeling methodologies for extracellular vesicle profiling on high-resolution single-particle platforms, Int. J. Mol. Sci., 2021, 22(19), 10510 CrossRef CAS PubMed.
- C. Bağcı,
et al., Overview of extracellular vesicle characterization techniques and introduction to combined reflectance and fluorescence confocal microscopy to distinguish extracellular vesicle subpopulations, Neurophotonics, 2022, 9(2), 021903 Search PubMed.
- A. Shehzad,
et al., Extracellular vesicles in cancer diagnostics and therapeutics, Pharmacol. Ther., 2021, 223, 107806 CrossRef CAS PubMed.
- K. E. Thane, A. M. Davis and A. M. Hoffman, Improved methods for fluorescent labeling and detection of single extracellular vesicles using nanoparticle tracking analysis, Sci. Rep., 2019, 9(1), 12295 CrossRef PubMed.
- R. Szatanek,
et al., The methods of choice for extracellular vesicles (EVs) characterization, Int. J. Mol. Sci., 2017, 18(6), 1153 CrossRef PubMed.
- C. M. Hoo,
et al., A comparison of atomic force microscopy (AFM) and dynamic light scattering (DLS) methods to characterize nanoparticle size distributions, J. Nanoparticle Res., 2008, 10, 89–96 CrossRef CAS.
- W. C. S. Cho, Extracellular Vesicles: Biology and Potentials in Cancer Therapeutics, Int. J. Mol. Sci., 2021, 22(17), 9586 CrossRef CAS PubMed.
- K. P. De Sousa,
et al., Isolation and characterization of extracellular vesicles and future directions in diagnosis and therapy, WIREs Nanomed. Nanobiotechnol., 2023, 15(1), e1835 CrossRef CAS PubMed.
- A. Kotrbová,
et al., TEM ExosomeAnalyzer: a computer-assisted software tool for quantitative evaluation of extracellular vesicles in transmission electron microscopy images, J. Extracell. Vesicles, 2019, 8(1), 1560808 CrossRef PubMed.
- Z. Zhao,
et al., Isolation and analysis methods of extracellular vesicles (EVs), Extracell. Vesicles Circ. Nucleic Acids, 2021, 2, 80–103 CAS.
- D. Bachurski,
et al., Extracellular vesicle measurements with nanoparticle tracking analysis–An accuracy and repeatability comparison between NanoSight NS300 and ZetaView, J. Extracell. Vesicles, 2019, 8(1), 1596016 CrossRef CAS PubMed.
- M. Malenica,
et al., Perspectives of Microscopy Methods for Morphology Characterisation of Extracellular Vesicles from Human Biofluids, Biomedicines, 2021, 9(6), 603 CrossRef CAS PubMed.
- Y. Yuana,
et al., Atomic force microscopy: a novel approach to the detection of nanosized blood microparticles, J. Thromb. Haemostasis, 2010, 8(2), 315–323 CrossRef CAS PubMed.
- P. Parisse,
et al., Atomic force microscopy analysis of extracellular vesicles, Eur. Biophys. J., 2017, 46, 813–820 CrossRef CAS PubMed.
- M. i. Li,
et al., Multiparametric atomic force microscopy imaging of single native exosomes, Acta Biochim. Biophys. Sin., 2021, 53(3), 385–388 CrossRef CAS PubMed.
- K. Singh,
et al., Separation of distinct exosome subpopulations: isolation and characterization approaches and their associated challenges, Analyst, 2021, 146(12), 3731–3749 RSC.
- T. Ito,
et al., Comparison of nanoparticle size and electrophoretic mobility measurements using a carbon-nanotube-based coulter counter, dynamic light scattering, transmission electron microscopy, and phase analysis light scattering, Langmuir, 2004, 20(16), 6940–6945 CrossRef CAS PubMed.
- S. L. Maas, M. L. Broekman and J. de Vrij, Tunable resistive pulse sensing for the characterization of extracellular vesicles. Exosomes and microvesicles, Methods Protoc., 2017, 21–33 CAS.
- A. Morales-Kastresana and J. C. Jones, Flow Cytometric Analysis of Extracellular Vesicles, Methods Mol. Biol., 2017, 1545, 215–225 CrossRef CAS PubMed.
- E. Van Der Pol,
et al., Optical and non-optical methods for detection and characterization of microparticles and exosomes, J. Thromb. Haemostasis, 2010, 8(12), 2596–2607 CrossRef CAS PubMed.
- K. P. De Sousa,
et al., Isolation and characterization of extracellular vesicles and future directions in diagnosis and therapy, Wiley Interdiscip. Rev.:Nanomed. Nanobiotechnol., 2023, 15(1), e1835 CAS.
- J. M. Carnino, H. Lee and Y. Jin, Isolation and characterization of extracellular vesicles from Broncho-alveolar lavage fluid: a review and comparison of different methods, Respir, 2019, 20, 1–11 CAS.
- N. Salmond,
et al., Nanoscale flow cytometry for immunophenotyping and quantitating extracellular vesicles in blood plasma, Nanoscale, 2021, 13(3), 2012–2025 RSC.
- E. Serrano-Pertierra,
et al., Extracellular vesicles: current analytical techniques for detection and quantification, Biomolecules, 2020, 10(6), 824 CrossRef CAS PubMed.
- D. L. Rupert,
et al., Methods for the physical characterization and quantification of extracellular vesicles in biological samples, Biochim. Biophys. Acta Gen. Subj., 2017, 1861(1), 3164–3179 CrossRef CAS PubMed.
- G. Midekessa,
et al., Zeta potential of extracellular vesicles: toward understanding the attributes that determine colloidal stability, ACS Omega, 2020, 5(27), 16701–16710 CrossRef CAS PubMed.
- S. Y. Liu,
et al., Diagnostic Role of Extracellular Vesicles in Cancer: A Comprehensive Systematic Review and Meta-Analysis, Front. Cell Dev. Biol., 2021, 9, 705791 CrossRef PubMed.
- E. M. Veziroglu and G. I. Mias, Characterizing extracellular vesicles and their diverse RNA contents, Front. Genet., 2020, 11, 700 CrossRef CAS PubMed.
- E. Weatherall and G. R. Willmott, Applications of tunable resistive pulse sensing, Analyst, 2015, 140(10), 3318–3334 RSC.
- T. Akagi,
et al., On-chip immunoelectrophoresis of extracellular vesicles released from human breast cancer cells, One, 2015, 10(4), e0123603 CrossRef PubMed.
- K. P. De Sousa,
et al., Proteomic identification of the contents of small extracellular vesicles from in vivo Plasmodium yoelii infection, Int. J. Parasitol., 2022, 52(1), 35–45 CrossRef CAS PubMed.
- Y. Yuana, A. Sturk and R. Nieuwland, Extracellular vesicles in physiological and pathological conditions, Blood Rev., 2013, 27(1), 31–39 CrossRef CAS PubMed.
- J. Conde-Vancells,
et al., Characterization and comprehensive proteome profiling of exosomes secreted by hepatocytes, J. Proteome Res., 2008, 7(12), 5157–5166 CrossRef CAS PubMed.
- H. Shao,
et al., New technologies for analysis of extracellular vesicles, Chem. Rev., 2018, 118(4), 1917–1950 CrossRef CAS PubMed.
- R. R. Mizenko,
et al., Tetraspanins are unevenly distributed across single extracellular vesicles and bias sensitivity to multiplexed cancer biomarkers, J. Nanobiotechnol., 2021, 19(1), 250 CrossRef CAS PubMed.
- J. Lötvall, A. F. Hill, F. Hochberg, E. I. Buzás, D. Di Vizio, C. Gardiner, Y. S. Gho, I. V. Kurochkin, S. Mathivanan, P. Quesenberry, S. Sahoo, H. Tahara, M. H. Wauben, K. W. Witwer and C. Théry, Minimal experimental requirements for definition of extracellular vesicles and their functions: a position statement from the International Society for Extracellular Vesicles, J. Extracell. Vesicles, 2014, 3, 26913 CrossRef PubMed.
- Y. Yoshioka,
et al., Comparative marker analysis of extracellular vesicles in different human cancer types, J. Extracell. Vesicles, 2013, 2(1), 20424 CrossRef PubMed.
- J. Suthar,
et al., Recent developments in biosensing methods for extracellular vesicle protein characterization, Wiley Interdiscip. Rev.:Nanomed. Nanobiotechnol., 2023, 15(1), e1839 CAS.
- T. A. Hartjes,
et al., Extracellular vesicle quantification and characterization: common methods and emerging approaches, Bioengineering, 2019, 6(1), 7 CrossRef CAS PubMed.
- Y. Jiang,
et al., Aptamer/AuNP biosensor for colorimetric profiling of exosomal proteins, Angew. Chem. Int. Ed., 2017, 56(39), 11916–11920 CrossRef CAS PubMed.
- B. Domon and R. Aebersold, Options and considerations when selecting a quantitative proteomics strategy, Nat. Biotechnol., 2010, 28(7), 710–721 CrossRef CAS PubMed.
- A. Y. T. Wu, K. Ueda and C. P. K. Lai, Proteomic analysis of extracellular vesicles for cancer diagnostics, Proteomics, 2019, 19(1–2), 1800162 CrossRef PubMed.
- S. Rontogianni,
et al., Proteomic
profiling of extracellular vesicles allows for human breast cancer subtyping, Commun. Biol., 2019, 2(1), 325 CrossRef PubMed.
- J. Zhou,
et al., A sensitive detection assay based on signal amplification technology for Alzheimer's disease's early biomarker in exosome, Anal. Chim. Acta, 2018, 1022, 124–130 CrossRef CAS PubMed.
- K. Lee,
et al., Multiplexed profiling of single extracellular vesicles, ACS Nano, 2018, 12(1), 494–503 CrossRef CAS PubMed.
- W. Zhao,
et al., Microsphere mediated exosome isolation and ultra-sensitive detection on a dielectrophoresis integrated microfluidic device, Analyst, 2021, 146(19), 5962–5972 RSC.
- M. Huang,
et al., Homogeneous, low-volume, efficient, and sensitive quantitation of circulating exosomal PD-L1 for cancer diagnosis and immunotherapy response prediction, Angew. Chem., 2020, 132(12), 4830–4835 CrossRef.
- Y. Li,
et al., Molecular identification of tumor-derived extracellular vesicles using thermophoresis-mediated DNA computation, J. Am. Chem. Soc., 2021, 143(3), 1290–1295 CrossRef CAS PubMed.
- S. A. Hinger,
et al., Diverse long RNAs are differentially sorted into extracellular vesicles secreted by colorectal cancer cells, Cell Rep., 2018, 25(3), 715–725 CrossRef CAS PubMed.
- K. O'Brien,
et al., RNA delivery by extracellular vesicles in mammalian cells and its applications, Nat. Rev. Mol. Cell Biol., 2020, 21(10), 585–606 CrossRef PubMed.
- V. N. Aushev,
et al., Comparisons of microRNA patterns in plasma before and after tumor removal reveal new biomarkers of lung squamous cell carcinoma, PloS One, 2013, 8(10), e78649 CrossRef CAS PubMed.
- L. Manterola,
et al., A small noncoding RNA signature found in exosomes of GBM patient serum as a diagnostic tool, Neuro Oncol., 2014, 16(4), 520–527 CrossRef CAS PubMed.
- D. Qin,
et al., Detection of pancreatic ductal adenocarcinoma by a qPCR-based normalizer-free circulating extracellular vesicles RNA signature, J. Cancer, 2021, 12(5), 1445 CrossRef CAS PubMed.
- P. M. Ozawa,
et al., Identification of miRNAs enriched in extracellular vesicles derived from serum samples of breast cancer patients, Biomolecules, 2020, 10(1), 150 CrossRef CAS PubMed.
- H. Xu,
et al., Circular RNA expression in extracellular vesicles isolated from serum of patients with endometrial cancer, Epigenomics, 2018, 10(2), 187–197 CrossRef CAS PubMed.
- L. Dong,
et al., Circulating long RNAs in serum extracellular vesicles: their characterization and potential application as biomarkers for diagnosis of colorectal cancer, Cancer Epidemiol. Biomarkers Prev., 2016, 25(7), 1158–1166 CrossRef CAS PubMed.
- L. Moldovan,
et al., Analyzing the circulating microRNAs in exosomes/extracellular vesicles from serum or plasma by qRT-PCR. Circulating MicroRNAs, Methods Protoc., 2013, 129–145 CAS.
- M. Del Re,
et al., Reply to Ugo De Giorgi, Vincenza Conteduca, and Emanuela Scarpi's Letter to the Editor re: Marzia Del Re, Elisa Biasco, Stefania Crucitta, et al. The Detection of Androgen Receptor Splice Variant 7 in Plasma-derived Exosomal RNA Strongly Predicts Resistance to Hormonal Therapy in Metastatic Prostate Cancer Patients. Eur Urol 2017; 71: 680-7, Eur. Urol., 2017, 73(1), e11–e12 Search PubMed.
- W. W. Chen,
et al., BEAMing and droplet digital PCR analysis of mutant IDH1 mRNA in glioma patient serum and cerebrospinal fluid extracellular vesicles, Mol. Ther. Nucleic Acids, 2013, 2, e109 CrossRef PubMed.
- M. G. Amorim,
et al., A total transcriptome profiling method for plasma-derived extracellular vesicles: applications for liquid biopsies, Rep, 2017, 7(1), 1–11 CAS.
- L. Cheng,
et al., Characterization and deep sequencing analysis of exosomal and non-exosomal miRNA in human urine, Kidney Int., 2014, 86(2), 433–444 CrossRef CAS PubMed.
- A. A. Sabo,
et al., Small non-coding RNA profiling in plasma extracellular vesicles of bladder cancer patients by next-generation sequencing: expression levels of miR-126-3p and piR-5936 increase with higher histologic grades, Cancers, 2020, 12(6), 1507 CrossRef CAS PubMed.
- J. D. Kuhlmann,
et al., Extracellular vesicle-associated miRNAs in ovarian cancer–design of an integrated NGS-based workflow for the identification of blood-based biomarkers for platinum-resistance, Clin. Chem. Lab. Med., 2019, 57(7), 1053–1062 CrossRef CAS PubMed.
- T. Bjørnetrø,
et al., Next-generation sequencing reveals mitogenome diversity in plasma extracellular vesicles from colorectal cancer patients, BMC Cancer, 2023, 23(1), 650 CrossRef PubMed.
- J. W. Roy,
et al., Small RNA sequencing analysis of peptide-affinity isolated plasma extracellular vesicles distinguishes pancreatic cancer patients from non-affected individuals, Sci. Rep., 2023, 13(1), 9251 CrossRef CAS PubMed.
- S. Kreimer,
et al., Mass-spectrometry-based molecular characterization of extracellular vesicles: lipidomics and proteomics, J. Proteome Res., 2015, 14(6), 2367–2384 CrossRef CAS PubMed.
- A. Zebrowska,
et al., Metabolome of exosomes: Focus on vesicles released by cancer cells and present in human body fluids, Int. J. Mol. Sci., 2019, 20(14), 3461 CrossRef CAS PubMed.
- D. Schwudke,
et al., Shotgun lipidomics on high resolution mass spectrometers, Cold Spring Harbor Perspect. Biol., 2011, 3(9), a004614 Search PubMed.
- N. Nishida-Aoki,
et al., Lipidomic analysis of cells and extracellular vesicles from high-and low-metastatic triple-negative breast cancer, Metabolites, 2020, 10(2), 67 CrossRef CAS PubMed.
- J. S. Brzozowski,
et al., Lipidomic profiling of extracellular vesicles derived from prostate and prostate cancer cell lines, Lipids Health Dis., 2018, 17, 1–12 CrossRef PubMed.
- T. Altadill,
et al., Enabling metabolomics based biomarker discovery studies using molecular phenotyping of exosome-like vesicles, PLoS One, 2016, 11(3), e0151339 CrossRef PubMed.
- M. Clos-Garcia,
et al., Metabolic alterations in urine extracellular vesicles are associated to prostate cancer pathogenesis and progression, J. Extracell. Vesicles, 2018, 7(1), 1470442 CrossRef PubMed.
- R. Hayasaka,
et al., Metabolomic analysis of small extracellular vesicles derived from pancreatic cancer cells cultured under normoxia and hypoxia, Metabolites, 2021, 11(4), 215 CrossRef CAS PubMed.
- M. Harmati,
et al., The role of the metabolite cargo of extracellular vesicles in tumor progression, Cancer Metastasis Rev., 2021, 1–19 Search PubMed.
- M. Palviainen,
et al., Cancer alters the metabolic fingerprint of extracellular vesicles, Cancers, 2020, 12(11), 3292 CrossRef CAS PubMed.
- M. Čuperlović-Culf,
et al., Analysis and simulation of glioblastoma cell lines-derived extracellular vesicles metabolome, Metabolites, 2020, 10(3), 88 CrossRef PubMed.
- R. A. Youness,
et al., A comprehensive insight and in Silico analysis of CircRNAs in hepatocellular carcinoma: a step toward ncRNA-based precision medicine, Cells, 2024, 13(15), 1245 Search PubMed.
- Y. Ma,
et al., Extracellular vesicles: an emerging nanoplatform for cancer therapy, Front. Oncol., 2021, 10, 606906 CrossRef PubMed.
- D. Sousa, R. T. Lima and M. H. Vasconcelos, Intercellular transfer of cancer drug resistance traits by extracellular vesicles, Trends Mol. Med., 2015, 21(10), 595–608 CrossRef CAS PubMed.
- J. Liu,
et al., Functional extracellular vesicles engineered with lipid-grafted hyaluronic acid effectively reverse cancer drug resistance, Biomaterials, 2019, 223, 119475 CrossRef CAS PubMed.
- Q. Lin,
et al., Exosome-like nanoplatform modified with targeting ligand improves anti-cancer and anti-inflammation effects of imperialine, J. Contr. Release, 2019, 311–312, 104–116 CrossRef CAS PubMed.
- L. Qiao,
et al., Tumor cell-derived exosomes home to their cells of origin and can be used as Trojan horses to deliver cancer drugs, Theranostics, 2020, 10(8), 3474 CrossRef CAS PubMed.
- K.-L. Zhang,
et al., Artificial chimeric exosomes for anti-phagocytosis and targeted cancer therapy, Chem. Sci., 2019, 10(5), 1555–1561 RSC.
- Y. Tian,
et al., A doxorubicin delivery platform using engineered natural membrane vesicle exosomes for targeted tumor therapy, Biomaterials, 2014, 35(7), 2383–2390 CrossRef CAS PubMed.
- M. J. Haney,
et al., Macrophage-derived extracellular vesicles as drug delivery systems for triple negative breast cancer (TNBC) therapy, J. Neuroimmune Pharmacol., 2020, 15, 487–500 CrossRef PubMed.
- R. Kanchanapally,
et al., Drug-loaded exosomal preparations from different cell types exhibit distinctive loading capability, yield, and antitumor efficacies: a comparative analysis, Int. J. Nanomed., 2019, 531–541 CrossRef CAS PubMed.
- G. Rashid,
et al., miRNA expression in PCOS: Unveiling a paradigm shift toward biomarker discovery, Arch. Gynecol. Obstet., 2024, 309(5), 1707–1723 CrossRef CAS PubMed.
- S. M. El-Daly, R. M. Talaat, M. Braoudaki, R. A. Youness and W. C. Cho, Editorial: Recent breakthroughs in the decoding of circulating nucleic acids and their applications to human diseases, Front. Mol. Biosci., 2023, 10, 1203495 CrossRef PubMed.
- M. Muskan,
et al., Therapeutic potential of RNA-enriched extracellular vesicles: the next generation in RNA delivery via biogenic nanoparticles, Mol. Ther., 2024, 2939–2949 CrossRef CAS PubMed.
- H. I. Kim,
et al., Recent advances in extracellular vesicles for therapeutic cargo delivery, Exp. Mol. Med., 2024, 56(4), 836–849 CrossRef CAS PubMed.
- X. Wang,
et al., Exosomes serve as nanoparticles to deliver anti-miR-214 to reverse chemoresistance to cisplatin in gastric cancer, Mol. Ther., 2018, 26(3), 774–783 CrossRef CAS PubMed.
- H. Huang,
et al., MicroRNA profiling of exosomes derived from red blood cell units: implications in transfusion-related immunomodulation, BioMed Res. Int., 2019, 2019(1), 2045915 Search PubMed.
- G. Liang,
et al., Engineered exosome-mediated delivery of functionally active miR-26a and its enhanced suppression effect in HepG2 cells, Int. J. Nanomed., 2018, 585–599 CrossRef CAS PubMed.
-
M. Pirisinu, et al., Extracellular vesicles as natural therapeutic agents and innate drug delivery systems for cancer treatment: Recent advances, current obstacles, and challenges for clinical translation, in Seminars in Cancer Biology, Elsevier, 2022 Search PubMed.
- Z. Belhadj,
et al., A combined "eat me/don't eat me" strategy based on extracellular vesicles for anticancer nanomedicine, J. Extracell. Vesicles, 2020, 9(1), 1806444 CrossRef CAS PubMed.
- T. L. Whiteside, Therapeutic targeting of oncogenic KRAS in pancreatic cancer by engineered exosomes, Transl. Cancer Res., 2017, 6(Suppl 9), S1406–s1408 CrossRef CAS PubMed.
- S. Nag,
et al., Nanomaterials-assisted photothermal therapy for breast cancer: State-of-the-art advances and future perspectives, Photodiagnosis Photodyn. Ther., 2024, 45, 103959 CrossRef CAS PubMed.
- M. Chehelgerdi,
et al., Progressing nanotechnology to improve targeted cancer treatment: overcoming hurdles in its clinical implementation, Mol. Cancer, 2023, 22(1), 169 CrossRef PubMed.
- Y. Cao,
et al., Engineered exosome-mediated near-infrared-II region V2C quantum dot delivery for nucleus-target low-temperature photothermal therapy, ACS Nano, 2019, 13(2), 1499–1510 CAS.
- S. Pan,
et al., Passion fruit-like exosome-PMA/Au-BSA@ Ce6 nanovehicles for real-time fluorescence imaging and enhanced targeted photodynamic therapy with deep penetration and superior retention behavior in tumor, Biomaterials, 2020, 230, 119606 CrossRef CAS PubMed.
- M. Abdel-Latif and R. A. Youness, Why natural killer cells in triple negative breast cancer?, World J. Clin. Oncol., 2020, 11(7), 464 CrossRef PubMed.
- M. Abdel-Latif,
et al., MALAT-1/p53/miR-155/miR-146a ceRNA circuit tuned by methoxylated quercitin glycoside alters immunogenic and oncogenic profiles of breast cancer, Mol. Cell. Biochem., 2022, 477(4), 1281–1293 CrossRef CAS PubMed.
- N. N. Parayath, S. Padmakumar and M. M. Amiji, Extracellular vesicle-mediated nucleic acid transfer and reprogramming in the tumor microenvironment, Cancer Lett., 2020, 482, 33–43 CrossRef CAS PubMed.
- W. Fu,
et al., CAR exosomes derived from effector CAR-T cells have potent antitumour effects and low toxicity, Nat. Commun., 2019, 10(1), 4355 CrossRef PubMed.
- X. Shi,
et al., Genetically engineered cell-derived nanoparticles for targeted breast cancer immunotherapy, Mol. Ther., 2020, 28(2), 536–547 CrossRef CAS PubMed.
- Q. Cheng,
et al., Reprogramming exosomes as nanoscale controllers of cellular immunity, J. Am. Chem.Society, 2018, 140(48), 16413–16417 CrossRef CAS PubMed.
- M. Wu,
et al., Extracellular vesicles: Emerging anti-cancer drugs and advanced functionalization platforms for cancer therapy, Drug Deliv., 2022, 29(1), 2513–2538 CrossRef CAS PubMed.
- L. Zhu,
et al., Folate-engineered microvesicles for enhanced target and synergistic therapy toward breast cancer, ACS Appl. Mater. Interfaces, 2017, 9(6), 5100–5108 CrossRef CAS PubMed.
- G. Liang,
et al., Engineered exosomes for targeted co-delivery of miR-21 inhibitor and chemotherapeutics to reverse drug resistance in colon cancer, J. Nanobiotechnol., 2020, 18(1), 1–15 CrossRef PubMed.
- J. Wang,
et al., Designer exosomes for active targeted chemo-photothermal synergistic tumor therapy, Adv. Funct. Mater., 2018, 28(18), 1707360 CrossRef.
- W. Zhou,
et al., Pancreatic cancer-targeting exosomes for enhancing immunotherapy and reprogramming tumor microenvironment, Biomaterials, 2021, 268, 120546 CrossRef CAS PubMed.
- L. Cheng,
et al., Gene-engineered exosomes-thermosensitive liposomes hybrid nanovesicles by the blockade of CD47 signal for combined photothermal therapy and cancer immunotherapy, Biomaterials, 2021, 275, 120964 CrossRef CAS PubMed.
- G. Wang,
et al., Cocktail strategy based on NK cell-derived exosomes and their biomimetic nanoparticles for dual tumor therapy, Cancers, 2019, 11(10), 1560 CrossRef CAS PubMed.
- Y. Wang,
et al., Delivering antisense oligonucleotides across the blood-brain barrier by tumor cell-derived small apoptotic bodies, Adv. Sci., 2021, 8(13), 2004929 CrossRef CAS PubMed.
- H. Cheng,
et al., Chimeric peptide engineered exosomes for dual-stage light guided plasma membrane and nucleus targeted photodynamic therapy, Biomaterials, 2019, 211, 14–24 CrossRef CAS PubMed.
- S. Li,
et al., Engineering extracellular vesicles for cancer therapy: recent advances and challenges in clinical translation, Biomater. Sci., 2020, 8(24), 6978–6991 RSC.
- R. Kalluri, The biology and function of exosomes in cancer, J. Clin. Investig., 2016, 126(4), 1208–1215 CrossRef PubMed.
- J. Palma,
et al., MicroRNAs are exported from malignant cells in customized particles, Nucleic Acids Res., 2012, 40(18), 9125–9138 CrossRef CAS PubMed.
- S. Y. Ko,
et al., The glycoprotein CD147 defines miRNA-enriched extracellular vesicles that derive from cancer cells, J. Extracell. Vesicles, 2023, 12(4), 12318 CrossRef CAS PubMed.
- L. T. Vu,
et al., microRNA exchange via extracellular vesicles in cancer, Cell Prolif., 2020, 53(11), e12877 CrossRef CAS PubMed.
- H. Sork,
et al., Heterogeneity and interplay of the extracellular vesicle small RNA transcriptome and proteome, Sci. Rep., 2018, 8(1), 10813 CrossRef PubMed.
- B. N. Hannafon,
et al., Plasma exosome microRNAs are indicative of breast cancer, Breast Cancer Res., 2016, 18, 1–14 CrossRef PubMed.
- A. Turchinovich, L. Weiz and B. Burwinkel, Extracellular miRNAs: the mystery of their origin and function, Trends Biochem. Sci., 2012, 37(11), 460–465 CrossRef CAS PubMed.
- L. Pigati,
et al., Selective Release of MicroRNA Species from Normal and Malignant Mammary Epithelial Cells, PLoS One, 2010, 5(10), e13515 CrossRef PubMed.
- R. Crescitelli,
et al., Subpopulations of extracellular vesicles from human metastatic melanoma tissue identified by quantitative proteomics after optimized isolation, J. Extracell. Vesicles, 2020, 9(1), 1722433 CrossRef CAS PubMed.
- T. Vagner,
et al., Protein composition reflects extracellular vesicle heterogeneity, Proteomics, 2019, 19(8), 1800167 CrossRef PubMed.
- E. Willms,
et al., Cells release subpopulations of exosomes with distinct molecular and biological properties, Sci. Rep., 2016, 6(1), 22519 CrossRef CAS PubMed.
- W. Meng,
et al., Prospects and challenges of extracellular vesicle-based drug delivery system: considering cell source, Drug Deliv., 2020, 27(1), 585–598 CrossRef CAS PubMed.
- X. Luan,
et al., Engineering exosomes as refined biological nanoplatforms for drug delivery, Acta Pharmacol. Sin., 2017, 38(6), 754–763 CrossRef CAS PubMed.
- P. Vader,
et al., Extracellular vesicles for drug delivery, Adv. Drug Deliv. Rev., 2016, 106, 148–156 CrossRef CAS PubMed.
- R. van der Meel,
et al., Extracellular vesicles as drug delivery systems: lessons from the liposome field, J. Contr. Release, 2014, 195, 72–85 CrossRef CAS PubMed.
- M. J. Haney,
et al., Exosomes as drug delivery vehicles for Parkinson's disease therapy, J. Contr. Release, 2015, 207, 18–30 CrossRef CAS PubMed.
- M. S. Kim,
et al., Development of exosome-encapsulated paclitaxel to overcome MDR in cancer cells, Nanomed. Nanotechnol. Biol. Med., 2016, 12(3), 655–664 CrossRef CAS PubMed.
- A. Jeyaram and S. M. Jay, Preservation and storage stability of extracellular vesicles for therapeutic applications, AAPS J., 2018, 20, 1–7 CrossRef CAS PubMed.
- J. H. Crowe,
et al., Stabilization of dry phospholipid bilayers and proteins by sugars, Biochem. J., 1987, 242(1), 1 CrossRef CAS PubMed.
- K. A. Murray and M. I. Gibson, Chemical approaches to cryopreservation, Nat. Rev. Chem, 2022, 6(8), 579–593 CrossRef CAS PubMed.
- S. Bosch,
et al., Trehalose prevents aggregation of exosomes and cryodamage, Sci. Rep., 2016, 6(1), 36162 CrossRef CAS PubMed.
- C. Charoenviriyakul,
et al., Erratum to'Preservation of exosomes at room temperature using lyophilization, Int. J. Pharm., 2018, 553, 1–7 CrossRef CAS PubMed . International Journal of Pharmaceutics, 2019. 559: pp. 427–428..
- R. Stranska,
et al., Comparison of membrane affinity-based method with size-exclusion chromatography for isolation of exosome-like vesicles from human plasma, J. Transl. Med., 2018, 16, 1–9 CrossRef CAS PubMed.
- G. Vergauwen,
et al., Confounding factors of ultrafiltration and protein analysis in extracellular vesicle research, Sci. Rep., 2017, 7(1), 1–12 CrossRef CAS PubMed.
- Q. Lin,
et al., Exosome-like nanoplatform modified with targeting ligand improves anti-cancer and anti-inflammation effects of imperialine, J. Contr. Release, 2019, 311, 104–116 CrossRef PubMed.
- K. Tang,
et al., Delivery of chemotherapeutic drugs in tumour cell-derived microparticles, Nat. Commun., 2012, 3(1), 1282 CrossRef PubMed.
- C. Admyre,
et al., Direct exosome stimulation of peripheral humanT cells detected by
ELISPOT, Eur. J. Immunol., 2006, 36(7), 1772–1781 CrossRef CAS PubMed.
- E. Koh,
et al., Exosome-SIRPα, a CD47 blockade increases cancer cell phagocytosis, Biomaterials, 2017, 121, 121–129 CrossRef CAS PubMed.
- D. Wang,
et al., Engineered cell-derived microparticles Bi2Se3/DOX@ MPs for imaging guided synergistic photothermal/low-dose chemotherapy of cancer, Adv. Sci., 2020, 7(3), 1901293 CrossRef CAS PubMed.
Footnote |
† Equal contribution. |
|
This journal is © The Royal Society of Chemistry 2025 |
Click here to see how this site uses Cookies. View our privacy policy here.