DOI:
10.1039/D4MD00652F
(Review Article)
RSC Med. Chem., 2025,
16, 525-544
Hepatocyte targeting via the asialoglycoprotein receptor
Received
22nd August 2024
, Accepted 19th November 2024
First published on 2nd December 2024
Abstract
This review highlights the potential of asialoglycoprotein receptor (ASGPR)-mediated targeting in advancing liver-specific treatments and underscores the ongoing progress in the field. First, we provide a comprehensive examination of the nature of ASGPR ligands, both natural and synthetic. Next, we explore various drug delivery strategies leveraging ASGPR, with a particular emphasis on the delivery of therapeutic nucleic acids such as small interfering RNAs (siRNAs) and antisense oligonucleotides (ASOs). An in-depth analysis of the current status of RNA interference (RNAi) and ASO-based therapeutics is included, detailing approved therapies and those in various stages of clinical development (phases 1 to 3). Afterwards, we give an overview of other ASGPR-targeted conjugates, such as those with peptide nucleic acids or aptamers. Finally, targeted protein degradation of extracellular proteins through ASGPR is briefly discussed.
Introduction
The asialoglycoprotein receptor (ASGPR), also known as the Ashwell–Morell receptor, was the first lectin to be discovered in mammals.1 Its carbohydrate recognition depends on calcium, classifying it as a C-type lectin.2 ASGPR specifically binds desialylated glycoproteins exhibiting non-reducing terminal galactose (Gal) or N-acetylgalactosamine (GalNAc). Upon binding, these desialylated glycoproteins undergo endocytosis through clathrin-coated pits. Subsequently, when reaching the acidic endosomal compartment, they detach from the receptor and are transported to the lysosomes, thereby maintaining homeostasis.3–6
ASGPR is a highly conserved transmembrane protein consisting of two subunits with a 58% sequence homology, each spanning from 40 to 60 kDa. These subunits, denominated H1 and H2 for the human hepatic lectin, are typically present in an average ratio of 2–3
:
1, respectively. Both subunits are essential for the correct functioning of the receptor.7–11
The receptor is expressed predominantly on hepatocytes and minimally on extra-hepatic cells, with as many as 500
000 surface binding sites per cell.11–15 It is endocytosed and recycled approximately every 15 minutes, regardless of the presence or absence of ligands.14,16,17 These characteristics render it a very attractive target for receptor-mediated drug delivery to the liver.12
Numerous serious diseases are associated with hepatocytes, including hepatitis B18 and C,19 hepatocellular carcinoma,20 and malaria.21 Infection with HBV or HCV may lead to chronic hepatitis, which can progress to cirrhosis and liver cancer. Consequently, targeting hepatocytes via interaction with ASGPR presents a promising strategy for treating these diseases.
ASGPR ligands
ASGPR carbohydrate recognition domain (CRD) is a shallow pocket that binds terminal sugars in a complex with calcium ion.22,23 It recognizes and binds terminal Gal (1) or GalNAc (2), with the latter exhibiting 10–60-fold higher affinity in competition assays (Fig. 1A).24 In certain cases, glucose (Glc) is also bound, albeit with a lower affinity than Gal and GalNAc.12 Binding primarily occurs through the hydroxy groups in positions 3 and 4, with the substituents in position 2 also contributing to the binding. An equatorial hydroxyl in position 3 is indispensable. An axial OH in position 4 increases the binding affinity, but an equatorial OH is also tolerated.25 Substituents in position 2 show a certain degree of tolerance; N-acyl groups enhance binding provided they are not too large. The region that hosts these substituents has been described as a dumbbell cavity.26,27 Substituents in the anomeric position and in position 6 have a minimal impact on CRD binding, which can be exploited in the design of synthetic ligands.25,28,29
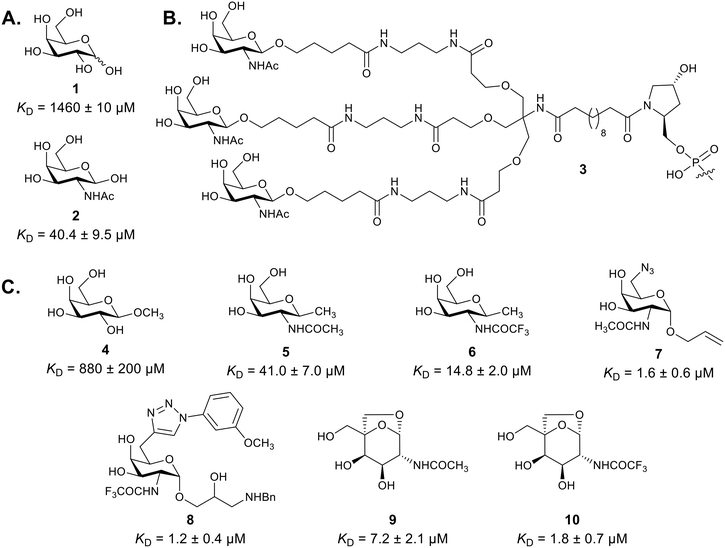 |
| Fig. 1 A. Structures and binding affinities of natural ASGPR ligands Gal (1)26 and GalNAc (2).22 B. Triantennary GalNAc ligand 3 built on Tris scaffold. C. Monovalent synthetic ASGPR ligands and their binding affinities.22 | |
The binding affinity increases from monoantennary to tetraantennary ligands. Monoantennary oligosaccharides bind in the millimolar (mM) range, whereas triantennary oligosaccharides bind in the nanomolar (nM) range, representing a 106-fold increase in the affinity despite only a three-fold increase in the absolute concentration of Gal.14,28 This phenomenon is referred to as the “cluster effect”.29 The increase in affinity from triantennary to tetraantennary ligands is only modest.12,30
Trivalent ligands are commonly constructed using a tris(hydroxymethyl)aminomethane (Tris) molecule as a scaffold (Fig. 1B). To this molecule, carbohydrate or glycomimetic ligands are attached via ether, ester or amide-based linkers (e.g. compound 3). The balance between the hydrophilicity, the hydrophobicity and the geometry greatly influence the ligand binding. It has been shown that an optimal separation between the terminal sugars is typically around 20 Å.31–33
Naturally occurring ASGPR binders include primarily glycoclusters. Endogenous ligands encompass mainly asialoorosomucoid (ASOR), asialofetuin (AF), asialoceruplasmin, asialotransferrin, immunoglobulin A (IgA), and von Willebrand factor (vWF).4,32–35 Notably, ASOR and AF served as benchmarks in the early studies of the receptor. Radiolabelled 125I-ASOR was used to elucidate the endocytosis kinetics and structural features of the receptor.15,16
Arabinogalactan and pullulan are natural polysaccharides based on galactose and glucose, respectively. They have been extensively studied for ASGPR-mediated targeting.36,37 Pullulan exploits the inability of ASGPR to discriminate between D-galactose and D-glucose.12 Nevertheless, the uptake of pullulan is lower than that of arabinogalactan.38
Synthetic ASGPR ligands (Fig. 1C) draw inspiration from natural binders. Although most of them still use GalNAc (2, Fig. 1A) as a warhead, numerous attempts to improve its affinity have been made.22 As mentioned above, positions 2 and 6 offer opportunities for modifications in order to improve the affinity. For example, replacing the acetamido group in position 2 with a trifluoroacetamido group enhanced the affinity (compare compounds 5 and 6), as did the replacement of 6-OH group with an azido (7) or a triazol (8) moiety.22 In 2017, M. G. Finn, V. Mascitti, and co-authors published a bridged ketal 9 (substituted 6,8-dioxabicyclo3.2.1]octane-2,3-diol), which was proposed based on the X-ray crystal structure of the ASGPR binding domain (PDB 1dv8).39,40 This bridged ketal enhanced the interaction between the hydrophobic α-face of the pyranose and the tryptophan residue Trp243. Compound 9 has a six-fold higher affinity compared to GalNAc and also a good ligand efficiency (LE = 0.45).39 Although an analogue of this compound, 10, with a trifluoroacetamido group in position 2 demonstrated a four-fold higher affinity, this compound was dismissed due to concerns regarding the long-term metabolic stability. Nevertheless, this warhead (10) is featured in a University of Yale patent for bi-functional molecules aimed at degrading circulating proteins.41 Avilar Therapeutics Inc., a company devoted to ASGPR therapeutics, drew inspiration from these two compounds and did extensive modifications and iterations, both on the bicyclic compound and on Gal. These modifications mainly involved eliminating substituents in position 1 in some monocyclic warheads, replacing the ketal bridge for an aliphatic bridge in the bicyclic warheads and introducing a wide variety of aryl rings in position 2.42–44
The majority of these synthetic warheads designed to target ASGPR are typically linked in multimers, commonly in trimers. The design and architecture of these ligands have been extensively reviewed by Huang et al.45 and therefore will not be discussed in this review.
Drug delivery strategies for targeting hepatocytes via ASGPR
Drug delivery strategies for liver targeted drugs via the interaction with ASGPR include direct GalNAc conjugation to therapeutic payloads (such as siRNAs, ASOs, and small-molecule drugs), nanoparticle-based delivery systems, and polymer-based drug delivery.
A comprehensive review of Gal-modified polymers and lipids was published in 2015.12 Recent research has focused on polymer–drug conjugates for delivering anticancer drugs to hepatocytes, particularly for treating hepatocellular carcinoma. Doxorubicin (DOX), one of the most widely used chemotherapeutics, often serves as a drug of choice. A review on DOX-base nanotherapeutics was published in 2019.46 Cationic polymers, such as poly-L-lysine (PLL) and poly-L-glutamic acid (PLGA), are effective vectors for gene delivery. These positively charged polymers interact with the anionic group of the ASGPR binding site and direct the formulations to the liver. Formulations of PLL modified with ASGPR ligands have demonstrated great efficacy in the transport to hepatic parenchymal cells.47–49 PK2 (FCE28069) is a copolymer derived from N-(2-hydroxypropyl)methacrylamide (HPMA) coupled with N-linked galactosamine and DOX, connected to the polymer backbone through a Gly-Phe-Leu-Gly peptidyl linker.49 In preclinical studies, the formulation displayed a five-fold decrease in cardiotoxicity compared to free DOX, supporting the progression of PK2 into early clinical investigation.50 Phase I trial in patients with primary or metastatic liver cancer showed that 17% of the administered dose was targeted to the liver, with 3% targeting the tumour.51 A recommended dosage for a phase II trial was established; however, no further progress has been reported.52
Targeted nanoparticles exploit the receptor-mediated endocytosis to deliver a drug payload to the liver while protecting it from degradation and facilitating the transport. These nanocarriers must degrade in a non-toxic manner to ensure safety and efficacy of the treatment. Clearance of hydrophobic nanoparticles by the reticuloendothelial system (RES) is a concern when employing this approach. To achieve a long circulation time, nanoparticles are typically coated with non-fouling polymers like PEG. However, this stealthy surface also impedes the uptake by the target cells. The incorporation of targeting ligands such as Gal or GalNAc for hepatocyte-specific delivery enhances uptake when employing this strategy.53 While liposomes are the most widely studied type of nanoparticles, other nanostructures such as micelles or dendrimers are also used.53–57 The Devarajan group reported DOX-containing nanoparticles decorated with pullulan and arabinogalactan for ASGPR targeting. They observed high selectivity in liver uptake (hepatocyte
:
nonparenchymal cell ratio 85
:
15). Pullulan-containing NPs showed high efficacy and greater safety compared to parent DOX.58
Yet another strategy are covalent drug–ligand conjugates.12 Petrov et al. prepared several glycoconjugates of small molecule drugs with GalNAc for targeted delivery to hepatocellular carcinoma. They conjugated paclitaxel,59 DOX60 and docetaxel.61 The latter displayed a nanomolar affinity for ASGPR, improved water solubility in comparison with parent docetaxel, and selective toxicity against hepatoma cells vs. control cell lines. Sanhueza et al. synthesized a conjugate of mifepristone (steroidal glucocorticoid antagonist, known also as RU-486) with a multivalent ASGPR ligand and observed an efficient accumulation of the conjugate in rat liver in vivo.39 Rico et al. published a conjugate of 5-fluorouracil with a triantennary GalNAc-based ligand intended to treat hepatocellular carcinoma.62 Apart from delivering anticancer agents, GalNAc conjugates are also used for targeted delivery of radiopharmaceuticals to the liver.63 The constructs typically contain a Gal or GalNAc as liver-targeting moiety, a linker, a chelating moiety and a radioisotope. Gamma-imaging radiotracers used in planar gamma scintigraphy and single-photon emission computed tomography (SPECT) most often employ 99mTc, while positron electron tomography (PET) employs 68Ga and 18F.64
Covalent linkage between GalNAc-based ASGPR targeting ligands and drug is also found in conjugates with therapeutic nucleic acids. These are discussed further in the following chapters.
Delivery of therapeutic nucleic acids to the liver
The clinical application of ASGPR-mediated targeting for gene silencing has emerged as a prominent area of interest. The objective is to impede mRNA translation using short complementary RNA fragments. GalNAc–RNA-based therapeutics have the potential to address unmet medical needs in numerous liver-related diseases. Two primary strategies employing this concept are the use of small interfering RNAs (siRNAs) and antisense oligonucleotides (ASOs).65 ASOs are single-stranded synthetic oligonucleotides (ONs) typically consisting of 16–20 nucleotides that bind to the target RNA by Watson–Crick base pairing.66 ASOs promote degradation by RNases, cause translational arrest by steric hindrance or interfere with the mRNA maturation, ultimately leading to downregulation of the target protein.67 siRNAs are short double-stranded ONs loaded into the RNA-induced silencing complex which then targets the mRNA for degradation.68
GalNAc–siRNA conjugates for RNA interference
RNA interference (RNAi) is an endogenous pathway for post-transcriptional silencing of gene expression that is triggered by double-stranded RNA (dsRNA). Through this pathway, siRNAs can silence the expression of virtually any gene with high efficiency and specificity, including “undruggable” targets.69
In 2014, Nair et al.70 proved that multivalent GalNAc-conjugated siRNA could elicit robust RNAi-mediated gene silencing in hepatocytes. Since then, several siRNA therapeutics targeted to hepatocytes have been developed and many others are in development.
However, delivery of siRNA is challenging as unmodified siRNA is susceptible to degradation by both extra- and intracellular nucleases, unstable in the bloodstream, immunogenic, and unable to penetrate cells. To circumvent these hurdles, the ONs are modified. Alnylam Pharmaceuticals (Alnylam) pioneered a modification pattern named standard template chemistry (STC), which includes changes to the 2′ position of the nucleotides, such as 2′-deoxy-2′-fluoro- or 2′-O-methyl and replacing phosphodiester bonds with phosphorothioate (PS). In the next generation, STC was succeeded by an enhanced stabilization chemistry (ESC) approach, leading to increased potency and prolonged duration of action.71,72 While working on GalNAc–siRNA conjugates, issues with off-target gene silencing were encountered, prompting the development of a new modification pattern called ESC+ (advanced ESC). To mitigate off-target seed interactions, glycol nucleic acid (GNA) was incorporated (Fig. 2A).73–76 Other companies like Arrowhead Pharmaceuticals (Arrowhead), Dicerna Pharmaceuticals (Dicerna), Silence Therapeutics (Silence), Arbutus Biopharma (Arbutus), OliX Pharmaceuticals (OliX), and Suzhou Ribo Life Science have developed their own sets of modifications for siRNA therapies.77
 |
| Fig. 2 A. Design of GalNAc–siRNA conjugates: STC – standard template chemistry, ESC – extended stabilization chemistry, ESC+ – extended stabilization chemistry plus, Silence platform – single GalNAc positioned at opposite sides of the sense strand. The top strand is the sense strand and the bottom is the antisense strand. B. Sirnaomics platform based on mxRNA and Dicerna's GalXC™ platform (passenger strand with tetraloop hairpin, ca. 36 bases, guide strand complementary to target mRNA, ca. 22 bases). C. Structure of monovalent GalNAc ligand built on serinol scaffold. Reproduced from ref. 78 with permission from the Royal Society of Chemistry, copyright 2023. | |
Arrowhead developed a versatile delivery platform TRiM™ (Targeted RNAi Molecule), which can be used for the delivery to the liver (using GalNAc as targeting molecule, directly conjugated to siRNA),79 lungs (using integrin alpha-v-beta-6 (αvβ6) ligand) or tumour (using Arg-Gly-Asp (RGD) peptide).80 In order to reduce off-target side effects, OliX has introduced asymmetric siRNA (asiRNA) technology. They have patented a GalNAc–asiRNA platform81 and several such conjugates are in preclinical and early stages of clinical trials. Dicerna/Novo Nordisk82 uses GalXC™ (pronounced like “galaxy”) and GalXC-Plus™ (targeting extrahepatic cell and tissue types) platforms. GalAhead™ (pronounced like “Galahad”) developed by Sirnaomics is based on miniaturised hairpin RNAi triggers (mxRNA™) and multi-unit RNAi triggers (muRNA™) rather than conventional siRNA (Fig. 2B).83,84 In 2020, Weingärtner et al. from Silence Therapeutics published a novel platform based on serinol-attached GalNAc unit(s) (11, Fig. 2C) either in a series (2–4 units) or two single GalNAc units positioned at opposite ends of the sense strand (Fig. 2A). This platform exhibited enhanced stability against endosomal and lysosomal degradation, as well as enhanced in vivo activity and duration of action when compared to a classical triantennary architecture.85 Other delivery platforms include mRNAi GOLD™ by Silence,86 RIBO-GalSTAR™ (GalNAc-based System for liver TARgeting) by Suzhou Ribo Life Science,87 and LEAD™ (Ligand and Enhancer Assisted Delivery) by SanegeneBio.
RNAi therapeutics utilizing GalNAc conjugate technology are administered subcutaneously. Intravenous injections lead to a rapid distribution of siRNAs to the liver, reaching a saturation state and wasting considerable doses. In contrast, subcutaneous injections give way to a slow release and absorption.80 Lower dose levels administered subcutaneously can maximize the proportion of drug reaching the liver.88
As with any therapeutic, understanding and monitoring pharmacodynamics (PD) and pharmacokinetics (PK) are essential for advancing through the development pipeline. In the case of GalNAc-conjugated siRNAs, there is a disconnect between plasma PK and PD effects: while plasma half-life is relatively short – 1–3 h in rats, 2–6 h in monkeys, and under 10 h in humans – the therapeutic effects persist for weeks in rodents and several months in monkeys and humans.89,90 This results in a delayed onset and extended duration of PD action. Although each therapeutic has its own dosing requirements determined in early-phase studies, treatments like givosiran and lumasiran are administered monthly, vutrisiran every three months, and inclisiran three months after the first dose, then every six months.91,92 GalNAc–siRNA conjugates bind to the ASGPR and undergo uptake into endosomes, where the conjugate dissociates from the receptor. The sugar moieties and branches are rapidly cleaved from the oligonucleotide, allowing the oligonucleotide to escape from the endosome and enter the cytoplasm (Fig. 3).75 The prolonged pharmacological activity is attributed to the gradual release of endocytosed conjugates.91,93
 |
| Fig. 3 Mechanism of action of siRNA–GalNAc conjugates. Reproduced from ref. 78 with permission from the Royal Society of Chemistry, copyright 2023. | |
An overview of the clinical advancement of siRNA–GalNAc conjugates is detailed in Table 1. The information was taken from the websites https://clinicaltrials.gov and https://anzctr.org.au, the pipeline data on the websites of the companies (Alnylam, Novo Nordisk, Arrowhead Pharmaceuticals, Silence Therapeutics, Arbutus Biopharma, Suzhou Ribo Life Science, OliX Pharma, Sirnaomics, SangeneBio) (accessed July 2024) and from literature reviews.80,94–96 As of July 2024, five GalNAc–siRNA drugs are on the market, six are in the late stage of development (phase III) and at least 27 are in the early stages of development (phase I and II). Compounds in preclinical development have not been included in this review.
Table 1 GalNAc–siRNA in the clinics or clinical development. Note: the lists of clinical trials and published results are not exhaustive
No. |
Name |
Company |
Indication |
Target |
Clinical phase |
Clinical trials |
Ref. |
† initially developed by |
* completed |
‡ currently being developed by |
** ongoing |
*** not yet started |
**** terminated |
Based on mxRNA rather than siRNA.
Announcement of the initiation of the phase I trial came on August 1, 2024.144
|
1 |
Givosiran (GIVLAARI), ALN-AS1 |
Alnylam |
Acute hepatic porphyria (AHP) |
5-Aminolevulinic acid synthase 1 (ALAS1) |
Registered |
NCT02949830* |
Lit.97–100 |
NCT03338816* |
NCT03505853* |
NCT02452372* |
NCT04883905** |
2 |
Lumasiran (OXLUMO), ALN-GO1 |
Alnylam |
Primary hyperoxaluria type 1 (PH1) |
Glycolate oxidase 1 (GO1) |
Registered |
NCT04152200** |
Lit.101–106 |
NCT03905694** |
NCT06225882** |
NCT04982393** |
NCT03350451* |
NCT03681184* |
NCT02706886* |
NCT06225544*** |
NCT05161936**** |
3 |
Inclisiran (LEQVIO), ALN-PCSsc |
Alnylam†/Novartis‡ |
Hypercholesterolemia (heterozygous familial and non-familial) or mixed dyslipidaemia |
Proprotein convertase subtilisin–kexin type 9 (PCSK9) |
Registered |
49 studies reported |
Lit.107–109 |
NCT03851705* |
NCT03060577* |
NCT03814187* |
NCT04807400* |
NCT02597127* |
NCT03397121* |
NCT02963311* |
NCT03400800* |
NCT03399370* |
NCT05362903** |
NCT03159416* |
4 |
Vutrisiran (AMVUTTRA), ALN-TTRsc02 |
Alnylam |
Transthyretin-mediated amyloidosis (ATTR) |
Transthyretin (TTR) |
Registered |
NCT03759379** |
Lit.110 |
Hereditory ATTR (hATTR) with polyneuropathy |
NCT05873868** |
ATTR with cardiomyopathy (ongoing) |
NCT04153149** |
NCT04561518** |
5 |
Nedosiran (RIVFLOZA), NN7022, DCR-PHXC |
Novo Nordisk |
Primary hyperoxaluria (PH) |
Lactate dehydrogenase (LDH) |
Registered |
NCT05001269** |
Lit.111,112 |
NCT04580420** |
NCT04042402** |
NCT03847909* |
NCT04555486* |
NCT03392896* |
6 |
Fitusiran, ALN-AT3sc |
Alnylam†/Sanofi‡ |
Hemophilia A and B, rare bleeding disorders (RBDs) |
Antithrombin (AT) |
Phase III |
NCT03974113** |
Lit.113,114 |
NCT06145373** |
NCT03754790** |
NCT05662319** |
NCT03754790** |
NCT03549871* |
NCT03417245* |
NCT03417102* |
NCT02035605* |
7 |
Cemdisiran, ALN-CC5 |
Alnylam |
Paroxysmal nocturnal hemoglobinuria, complement-mediated diseases, myasthenia gravis |
Complement C5 (CC5) |
Phase III |
NCT02035605* |
Lit.115 |
NCT05070858** |
NCT05744921** |
NCT05133531** |
NCT02352493* |
NCT04888507* |
NCT04811716* |
NCT04601844* |
NCT04940364* |
NCT03841448**** |
8 |
Plozasiran, ARO-APOC3 |
Arrowhead |
Familial chylomicronemia syndrome, severe hypertriglyceridemia |
Apolipoprotein C-III (apoC-III) |
Phase III |
NCT06347133** |
Lit.116–119 |
NCT06347016** |
NCT06347003** |
NCT05902598*** |
NCT05089084** |
NCT03783377* |
NCT04720534* |
|
|
Dyslipidemia |
|
Phase II |
NCT05413135** |
|
NCT04998201* |
9 |
Olpasiran, AMG 890, ARO-LPA |
Arrowhead†/Amgen‡ |
Cardiovascular disease |
Lipoprotein(a) (LPA) |
Phase III |
NCT05581303** |
Lit.120–122 |
NCT04270760* |
NCT05489614* |
NCT04987320* |
NCT05481411* |
NCT03626662* |
NCT06411860*** |
10 |
Fazirsiran, ARO-AAT, TAK-999 |
Arrowhead†/Takeda‡ |
α1 antitrypsin deficiency associated liver disease (AATD) |
Alpha-1 antitrypsin (AAT) |
Phase III |
28 studies reported |
Lit.123–125 |
NCT05891158** |
NCT05899673** |
NCT05677971** |
NCT06165341** |
NCT03946449** |
NCT03362242* |
NCT03945292* |
11 |
Lepodisiran, LY3819469 |
Dicerna†/Eli Lilly‡ |
Cardiovascular disease |
Lipoprotein(a) (LPA) |
Phase III |
NCT06292013** |
Lit.126 |
NCT05565742** |
NCT04914546* |
NCT05841277* |
NCT05932446* |
12 |
ARO-HBV, JNJ-73763989, JNJ-3989 |
Arrowhead†/GSK‡ |
Hepatitis B infection |
Hepatitis B viral proteins |
Phase II |
NCT04535544** |
Lit.88,127–129 |
NCT04439539* |
NCT04585789* |
NCT04002752* |
NCT04208386* |
NCT03365947* |
NCT03982186* |
NCT04129554* |
NCT04963738* |
NCT04586439* |
NCT04667104* |
NCT05005507**** |
13 |
Xalnesiran, RO7445482, DCR-HBVS, RG6346 |
Dicerna†/Roche‡ |
Hepatitis B infection |
Hepatitis B viral proteins |
Phase II |
NCT04225715** |
|
NCT03772249* |
14 |
Imdusiran, AB-729 |
Arbutus |
Hepatitis B infection |
Hepatitis B viral proteins |
Phase II |
NCT04980482** |
|
NCT06245291** |
NCT06154278** |
NCT04820686**** |
NCT04847440**** |
15 |
Elebsiran, ALN-HBV02, VIR-2218 |
Alnylam/Vir biotechnology |
Hepatitis B infection |
Hepatitis B viral proteins |
Phase II |
NCT05844228** |
Lit.130 |
NCT05484206** |
NCT04856085** |
NCT04412863** |
NCT05970289** |
NCT05612581** |
NCT04891770* |
NCT03672188* |
NCT04507269* |
NCT04749368* |
NCT06092333*** |
|
|
Hepatitis D infection |
Hepatitis B viral proteins |
Phase II |
NCT05461170** |
|
16 |
RBD1016 |
Suzhou Ribo Life Science |
Chronic hepatitis B |
Hepatitis B viral proteins |
Phase II |
NCT05961098** |
|
NCT04685564* |
NCT05017116* |
17 |
Zerlasiran, SLN360 |
Silence |
Cardiovascular disease |
Lipoprotein(a) (LPA) |
Phase II |
NCT05537571* |
Lit.131,132 |
NCT04606602* |
18 |
Zodasiran, ARO-ANG3 |
Arrowhead |
Mixed dyslipidemia, homozygous familial hypercholesterolemia |
Angiopoietin-like protein 3 (ANGPTL3) |
Phase II |
NCT04832971** |
Lit.133–137 |
NCT05217667** |
NCT03747224* |
19 |
Solbinsiran, LY3561774 |
Dicerna†/Eli Lilly‡ |
Dyslipidemia, cardiovascular disease |
ANGPTL3 |
Phase II |
NCT05256654* |
|
NCT04644809* |
20 |
Zilebesiran, ALN-AGT01, RG6615 |
Alnylam†/Roche‡ |
Hypertension |
Angiotensinogen (AGT) |
Phase II |
NCT04936035** |
Lit.138,139 |
NCT05103332** |
NCT06272487** |
NCT03934307* |
NCT06423352** |
21 |
GSK4532990, ARO-HSD |
Arrowhead†/GlaxoSmithKline‡ |
Metabolic dysfunction-associated steatohepatitis (MASH) |
17-Beta hydroxysteroid dehydrogenase 13 (HSD17B13) |
Phase II |
NCT06104319** |
Lit.140 |
NCT05583344** |
NCT04202354* |
22 |
ALN-HSD |
Alnylam in collaboration with Regeneron |
MASH |
HSD17B13 |
Phase II |
NCT05519475** |
|
NCT04565717**** |
23 |
Belcesiran, NN6021, DCR-A1AT-201 |
Dicerna†/Novo Nordisk‡ |
α1 antitrypsin deficiency associated liver disease (AATD) |
Serpin family A member (SERPINA-1) |
Phase II |
NCT04764448**** |
|
NCT04174118* |
NCT05146882**** |
24 |
ALN-KHK |
Alnylam |
Type 2 diabetes mellitus |
Ketohexokinase (KHK) |
Phase I/II |
NCT05761301** |
|
25 |
Divesiran, SLN124 |
Silence |
β-Thalassaemia and myelodysplastic syndrome (MDS) |
Transmembrane serine protease 6 (TMPRSS6) |
Phase I |
NCT04718844* |
Lit.141–143 |
NCT04559971* |
NCT04176653*** |
|
|
Polycythemia vera |
|
Phase I/II |
NCT05499013** |
|
26 |
LY3875383 |
Eli Lilly |
Cardiovascular disease |
Apolipoprotein C-III (APOC3) |
Phase I |
NCT05609825* |
|
27 |
RBD5044 |
Suzhou Ribo Life Science |
Triglycerides (TGs)-type hypertriglyceridemia |
APOC3 |
Phase I |
NCT05539651** |
|
28 |
LY3849891 |
Eli Lilly |
MASH |
Patatin-like phospholipase domain containing 3 (PNPLA3) |
Phase I |
NCT05395481** |
|
29 |
LY3885125 |
Eli Lilly |
MASH |
SCAP |
Phase I |
NCT06007651** |
|
30 |
ALN-PNP |
Alnylam |
MASH |
PNPLA3 |
Phase I |
NCT05648214** |
|
NCT06024408** |
31 |
NN6581, NNC0581–0001 |
Novo Nordisk |
MASH |
MARC1 |
Phase I |
NCT05599945** |
|
32 |
NN6582, NNC0582–0001 |
Novo Nordisk |
MASH |
LXRα |
Phase I |
NCT05624580** |
|
33 |
ARO-PNPLA3, JNJ-75220795 |
Janssen/Arrowhead |
MASH |
PNPLA3 |
Phase I |
NCT04844450* |
|
NCT05039710**** |
34 |
OLX702A, OLX75016 |
OliX |
MASH |
Undisclosed |
Phase I |
ACTRN12624000023550 |
|
35 |
ALN-TTRsc04 |
Alnylam |
ATTR |
TTR |
Phase I |
NCT05661916** |
|
36 |
ALN-BCAT |
Alnylam |
Hepatocellular carcinoma (HCC) |
β-Catenin |
Phase I |
Plan to initiate phase I in early 2024 |
|
37 |
ARO-C3 |
Arrowhead |
Complement-mediated diseases (paroxysmal nocturnal hemoglobinuria, complement-mediated renal disease) |
Complement component 3 |
Phase I |
NCT05083364** |
|
38 |
DCR-AUD |
Dicerna, a Novo Nordisk company |
Alcohol use disorder (AUD) |
Aldehyde dehydrogenase (ALDH2) |
Phase I |
NCT05021640* |
|
NCT05845398* |
39 |
ARO-CFB |
Arrowhead |
Complement-mediated disease |
Complement factor B (CFB) |
Phase I |
NCT06209177** |
|
40 |
RBD4059 |
Suzhou Ribo Life Science |
Thrombosis |
Coagulation factor XI (FXI) |
Phase I |
NCT05653037** |
|
41 |
RBD7022 |
Suzhou Ribo Life Science |
Hyperlipidemia |
PCSK9 |
Phase I |
NCT05912296** |
|
42 |
STP122Ga |
Sirnaomics |
Thrombosis |
Coagulation factor XI (FXI) |
Phase I |
NCT05844293** |
|
43 |
IBI3016, SGB-3908b |
Innovent/SanegeneBio |
Hypertension |
Angiotensinogen |
Phase I |
NCT06501586** |
|
The first attempt to clinically silence genes was made with revusiran (ALN-TTRsc, Alnylam Pharmaceuticals). This GalNAc–siRNA conjugate targeted hepatic transthyretin (TTR) production and was designed to treat cardiomyopathy caused by hereditary transthyretin-mediated amyloidosis (hATTR). The drug used the standard template chemistry (STC). Its development was discontinued in 2016 in a phase III trial for increased rate of mortality in patients treated with revusiran compared to placebo (ENDEAVOUR: NCT02319005).145 Although the compound did not make it to the market, it served as a valuable proof of concept.146 Interestingly, the second-generation siRNA–GalNAc conjugates use ESC rather than STC which enables the dose and exposure levels to be 12–30 times lower than those for revusiran. For example, patients treated with revusiran received 28 g per year compared to vutrisiran (new TTR GalNAc–siRNA conjugate, see below), which achieves similar pharmacodynamic effects with only 100 mg per year. This 280-fold lower drug exposure owed to the second-generation chemistry significantly improves safety.
Givosiran
(GIVLAARI, ALN-AS1, Alnylam Pharmaceuticals) was the first GalNAc–siRNA conjugate to reach the market. It is directed against 5-aminolevulinate synthase 1 and is used to treat acute hepatic porphyria, a genetic disorder resulting in the build-up of toxic porphyrin precursors formed during the production of heme. Givosiran was approved in 2019 after a successful phase III study (ENVISION: NCT03338816).97,147 FDA granted the application for givosiran breakthrough therapy and first-in-class medication designation, priority review designation, and an orphan drug status. Its pharmacological properties and role in the treatment of acute hepatic porphyria have been recently reviewed.98,148
Lumasiran
(OXLUMO, ALN-GO1, Alnylam Pharmaceuticals) was approved in 2020 after a successful phase III study (ILLUMINATE-A: NCT03681184)101,106 for the treatment of severe primary hyperoxaluria type 1 (PH1). The compound targets mRNA encoding for glycolate oxidase, thus reducing hepatic oxalate production.102,149 Lumasiran received orphan drug and breakthrough therapy designation. It also underwent a phase II clinical trial in patients with recurrent calcium oxalate kidney stone disease (NCT05161936). A phase II study in patients that have a kidney disease and need dialysis treatment is supposed to start in 2024 (NCT06225544).
Inclisiran
(LEQVIO, ALN-PCSsc, developed by The Medicines Company, a subsidiary of Novartis, which licensed the rights to inclisiran from Alnylam Pharmaceuticals), used for the treatment of hypercholesterolemia, targets proprotein convertase subtilisin/kexin type 9 (PCSK9). It was approved in the EU in 2020 and in the UK and USA in 2021 after successful phase III studies (ORION-9: NCT03397121, ORION-10: NCT03399370, ORION-11: NCT03400800).107–109 Its administration is necessary only every 3–6 months, which is a major advantage over statins and monoclonal antibody therapy.150 A number of further clinical studies, aimed among others at treating mixed dyslipidemia and atherosclerotic cardiovascular disease, are on-going (https://www.clinicaltrials.gov).
Vutrisiran
(Amvuttra, ALN-TTRsc02, Alnylam Pharmaceuticals), designed for the treatment of transthyretin-mediated amyloidosis, inhibits the production transthyretin protein by the liver.110 Vutrisiran was approved in 2022 both in the USA and EU for the treatment of the polyneuropathy of hATTR in adults.151 It has been granted orphan drug status in the US, EU and Japan for the treatment of ATTR. In the US, it has also been granted a fast track designation for the treatment of the polyneuropathy of hATTR in adults. Two phase III clinical studies are ongoing. HELIOS-A study (NCT03759379) in patients with hATTR with neuropathy was initiated in 2019 and is supposed to last until 2026. Vutrisiran is to be compared with patisiran (Onpattro), the first siRNA-based drug approved in the US and the first drug approved by the FDA to treat hATTR. A 2022 report confirmed that vutrisiran met all secondary endpoints measured at 18 months.152 When compared to patisiran, vutrisiran was found to be non-inferior in terms of serum transthyretin reduction. Vutrisiran's primary advantage over patisiran is its dosing regimen. While patients taking patisiran must undergo an 80-minute infusion every three weeks, vutrisiran is administered by a subcutaneous injection that is administered every three months. This highlights the advantage of directly conjugated siRNA therapeutics over lipid nanoparticle-based delivery to the targeted cells. HELIOS-B study (NCT04153149), also initiated in 2019, is running to evaluate the efficacy and safety of vutrisiran compared to placebo in patients with ATTR with cardiomyopathy.153
Nedosiran
(Rivfloza, DCR-PHXC, Novo Nordisk, which acquired Dicerna Pharmaceuticals in 2021) targets hepatic lactate dehydrogenase A and was approved in 2023 for the treatment of primary hyperoxaluria (PH).112,154 It is indicated to lower urinary oxalate levels in people with PH type 1. PH is a family of three autosomal recessive inherited disorders of hepatic glyoxylate metabolism characterised by oxalate overproduction. Subsequent calcium oxalate precipitation leads to nephrocalcinosis, recurrent urolithiasis, kidney damage, and ultimately to kidney or even multiple organ failure.155 FDA granted it a first-in-class status as well as breakthrough therapy and orphan drug designation. The drug is currently evaluated in a phase II study in paediatric patients (11 years of age or younger) who have PH1, PH2 or PH3 and relatively intact renal function (NCT05001269).
Six GalNAc–siRNA conjugates are currently in the late stage of development (phase III).
Fitusiran
(ALN-AT3sc, Sanofi Genzyme, licensed from Alnylam Pharmaceuticals) targets antithrombin (AT) and is in development for the treatment of hemophilia and rare bleeding disorders (RBDs).113,114 Phase III studies ATLAS-A/B (NCT03417245) and ATLAS-INH (NCT03417102) were completed in 2021, and long-term safety and efficacy study ATLAS-OLE (NCT03754790) and a study in paediatric patients ATLAS-PEDS (NCT03974113) are ongoing with expected completion date in 2026.
Cemdisiran
(ALN-CC5, Alnylam Pharmaceuticals) targets the C5 component of the complement pathway and is in development for the treatment of complement-mediated diseases.115 Cemdisiran is being evaluated mostly in combination with pozelimab in several phase III studies: NIMBLE study (NCT05070858) in patients with symptomatic generalised myasthenia gravis and ACCESS-1 (NCT05133531) and ACCESS-EXT (NCT05744921) in patients with paroxysmal nocturnal hemoglobinuria. In 2021, EU granted cemdisiran orphan designation for the treatment of primary IgA nephropathy (phase II completed in 2023, NCT03841448).
Plozasiran
(ARO-APOC3, Arrowhead Pharmaceuticals) targets apolipoprotein C-III and is in a phase III study in patients with familial chylomicronemia syndrome (NCT05089084) and with hypertriglyceridemia (NCT06347133). Two additional phase III studies in patients with hypertriglyceridemia are to start in 2024 (NCT06347016, NCT06347003).
Olpasiran
(AMG890, ARO-LPA, Amgen) reduces lipoprotein(a) synthesis in the liver. It is evaluated in a phase III study (NCT05581303) on the risk for coronary heart disease death, myocardial infarction, or urgent coronary revascularization in participants with atherosclerotic cardiovascular disease and elevated lipoprotein(a).
Fazirsiran
(ARO-AAT, Arrowhead Pharmaceuticals, in collaboration with Takeda) targets alpha-1 antitrypsin (AAT) and is studied for the treatment of liver disease associated with AAT deficiency (AATD) (phase III NCT05899673, NCT05677971, NCT06165341).123
Lepodisiran
(LY3819469, Eli Lilly) is directed at hepatic synthesis of apolipoprotein(a), an essential component necessary for assembly of lipoprotein(a) particles, which contribute to atherosclerotic disease and aortic stenosis. It is evaluated in a phase III study (NCT06292013) for the reduction of major adverse cardiovascular events in adults with elevated lipoprotein(a) who have established atherosclerotic cardiovascular disease or are at risk for a first cardiovascular event.
Five GalNAc–siRNA conjugates are in phase II trials against chronic HBV infection: xalnesiran (RO7445482, RG6346, DCR-HBVS, developed by Dicerna Pharmaceuticals/Novo Nordisk in collaboration with Hoffmann-La Roche), imdusiran (AB-729, Arbutus), elebsiran (ALN-HBV02, VIR-2218, Vir Biotechnology in collaboration with Alnylam), JNJ-3989 (ARO-HBV, Arrowhead Pharmaceuticals, initially licensed to Janssen Pharmaceuticals, subsequently to GlaxoSmithKline), and RB1016 (Suzhou Ribo Life Science). These conjugates are designed to inhibit expression of HBV proteins, including hepatitis B surface antigen (HBsAg). They are investigated as stand-alone therapy as well as for use in combination with other direct antiviral agents, such as nucleoside and nucleotide analogues. Additionally, elebsiran is also evaluated in combination with tobevibart, an IgG1-lambda humanised monoclonal antibody, in a phase II study against chronic hepatitis D.
Targeting ASGPR as hepatic receptor is also thoroughly studied for the treatment of various liver-related diseases. GSK4532990 (ARO-HSD, GlaxoSmithKline, phase II), ALN-HSD (Alnylam, in collaboration with Regeneron, phase II), ARO-PNPLA3 (JNJ-75220795, Arrowhead), LY3849891 (Eli Lilly, phase I), LY3885125 (Eli Lilly, phase I), ALN-PNP (Alnylam, phase I), NN6581 (Novo Nordisk, phase I), NN6582 (Novo Nordisk, phase I), and OLX702A (OLX75016, OliX, phase I) are being developed for the treatment of metabolic dysfunction-associated steatohepatitis (MASH), previously referred to as nonalcoholic steatohepatitis (NASH). MASH one of the most common chronic liver diseases worldwide, with a global prevalence of approximately 24% in the general population.156Belcesiran (NN6021, DCR-A1AT-201, Novo Nordisk, phase II) and ALN-TTRsc04 (Alnylam, phase I) target α-1 antitrypsin for the treatment of AAT deficiency-associated liver disease (α-1 liver disease). ALN-BCAT (Alnylam, phase I) targets β-catenin for the treatment of hepatocellular carcinoma.
A number of GalNAc–siRNA conjugates are in early development for the treatment of cardiovascular diseases, hypercholesterolemia and dyslipidemia. Zerlasiran (SLN360, Silence Therapeuticals, phase II) is designed to reduce the production of apolipoprotein A, a key component of lipoprotein(a), which has been genetically linked with increased risk of cardiovascular diseases, independent of cholesterol and LDL levels. Two conjugates target apolipoprotein C-III: LY3819469 (Eli Lilly, phase I), and RBD5044 (Suzhou Ribo Life Science, phase I). Two conjugates reduce the production of angiopoietin-like protein 3 (ANGPTL3), a liver-synthesised inhibitor of lipoprotein lipase and endothelial lipase: zodasiran (ARO-ANG3, Arrowhead, phase II), and solbinsiran (LY3561774, Eli Lilly, phase II). Zilebesiran (ALN-AGT01, RG6615, Roche, phase II) and IBI3016 (SGB-3908, phase I) target angiotensinogen (AGT) and are evaluated for the treatment of hypertension. RBD7022 (Suzhou Ribo Life Science, phase I) targets PCSK9 and is developed for the treatment of hyperlipidemia.
Complement-mediated diseases are targeted by ARO-C3 (Arrowhead, phase I) designed to reduce the production of complement component 3 and ARO-CFB (Arrowhead, phase I) reducing complement factor B.
Other GalNAc–siRNA conjugates in the early stage of development include divesiran (SLN124, Silence Therapeuticals, phase I/II) downregulating TMPRSS6 and developed to treat β-thalassaemia, myelodysplastic syndrome and polycythemia vera, ALN-KHK (Alnylam, phase I/II) targeting ketohexokinase to treat diabetes mellitus 2, DCR-AUD (DCR-A1203, Dicerna Pharmaceuticals/Novo Nordisk, phase I) designed to silence aldehyde dehydrogenase (ALDH2) for the treatment of alcohol use disorder, ALN-APP (Alnylam Pharmaceuticals) targeting amyloid precursor protein (APP) to treat Alzheimer's disease and cerebral amyloid angiopathy, and RBD4059 (Suzhou Ribo Life Science, phase I) and STP122G (Sirnaomics, phase I, based on mxRNA rather than siRNA) targeting coagulation factor XI as potential treatment of thrombosis.
GalNAc–ASO conjugates
Single strand modified ASOs conjugated to a triantennary GalNAc3 moiety were initially developed by Ionis Pharmaceuticals.157 These GalNAc–ASO conjugates are also known as ligand-conjugated antisense medicines (LICAs). Akcea Therapeutics was initially formed in 2014 as an Ionis subsidiary to further develop the compounds, but was acquired back by its parent company in 2020.
Similar to GalNAc-conjugated siRNAs, a thorough understanding of PK and PD is critical for advancing GalNAc-conjugated ASOs. After subcutaneous injection, the time to reach maximum plasma concentration ranges from 0.25 to 1 hour in mice and 1 to 4 hours in monkeys and humans. In mice, the major circulating species in the blood post-absorption is the conjugated ASO, but after 24 hours, the unconjugated ASO becomes the predominant species.157 Elimination half-lives across all tissues, including the liver, are 3–4 weeks in both monkeys and humans.158
An overview of the compounds on the market and in clinical development is summarised in Table 2.
Table 2 GalNAc–ASO conjugates on the market and in clinical development
No. |
Name |
Company |
Indication |
Target |
Clinical phase |
Clinical trials |
Ref. |
† initially developed by |
* completed |
‡ currently being developed by |
** ongoing |
*** not yet started |
**** terminated |
1 |
Wainua, eplontersen, ION-682884, IONIS-TTR-LRx, AKCEA-TTR-LRx, ISIS-TTRRx |
Akcea/Ionis†/Astra Zeneca‡ |
Transthyretin-mediated amyloidosis (ATTR) |
Transthyretin (TTR) |
Registered |
NCT06194825** |
Lit.159–162 |
NCT05667493** |
NCT05071300** |
NCT04136171** |
NCT06073587** |
NCT05259072** |
NCT06073574** |
NCT04302064* |
NCT04136184* |
NCT03728634* |
2 |
Pelacarsen, AKCEA-APO(a)-LRx, TQJ230, ISIS 681257 |
Akcea/Ionis†/Novartis‡ |
Cardiovascular disease |
Apolipoprotein(a) |
Phase III |
NCT05900141** |
Lit.163,164 |
NCT05646381** |
NCT05305664** |
NCT04023552** |
NCT03070782* |
NCT05337878* |
NCT05026996* |
NCT06267560*** |
NCT04993664**** |
3 |
Olezarsen, IONIS-APOCIII-LRx, AKCEA-APOCIII-LRx |
Akcea/Ionis |
Severe hypertriglyceridemia (sHTG) |
Apolipoprotein C-III (ApoC-III) |
Phase III |
NCT05681351** |
|
NCT05610280** |
NCT05552326** |
NCT05079919** |
NCT05579860* |
NCT05355402* |
|
|
Familial chylomicronemia syndrome (FCS) |
Apolipoprotein C-III (ApoC-III) |
Phase III |
NCT05185843** |
|
NCT06360237** |
NCT05130450** |
NCT04568434* |
4 |
Donidalorsen, IONIS-PKK-LRx, ISIS 721744 |
Ionis |
Hereditary angioedema |
Prekallikrein (PKK) |
Phase III |
NCT06415448** |
Lit.165,166 |
NCT05392114** |
NCT04307381** |
NCT03263507* |
NCT04030598* |
NCT05139810* |
5 |
Bepirovirsen, GSK3389404, IONIS-HBV-LRx |
Ionis†/GSK‡ |
Hepatitis B infection |
Hepatitis B viral protein |
Phase III |
NCT06058390** |
Lit.167 |
NCT05630807** |
NCT05630820** |
NCT05276297** |
NCT05330455** |
NCT06422767*** |
NCT03020745* |
NCT02647281* |
6 |
Fesomersen, BAY2976217, ISIS-FXIRx, ISIS 416858, IONIS-FXI-LRx |
Ionis†/Bayer‡ |
Thrombosis, clotting disorders |
Factor XI |
Phase II |
NCT03582462* |
Lit.168 |
NCT04534114* |
NCT02553889* |
NCT03358030* |
NCT01713361* |
7 |
Sapablursen, IONIS-TMPRSS6-LRx |
Ionis |
β-Thalassaemia, polycythemia vera |
Transmembrane serine protease 6 (TMPRSS6) |
Phase II |
NCT05143957** |
Lit.169 |
NCT03165864* |
NCT04059406**** |
8 |
IONIS-FB-LRx, RG6299 |
Ionis†/Roche‡ |
IgA nephropathy (IgAN) |
Complement factor B (FB) |
Phase II |
NCT04014335* |
Lit.170 |
NCT03815825* |
NCT03446144*** |
|
|
Age-related macular degeneration, geographic atrophy |
Complement factor B (FB) |
Phase II |
NCT03815825** |
|
NCT03446144**** |
9 |
ION904 |
Ionis |
Treatment-resistant hypertension (TRH) |
Angiotensinogen |
Phase II |
NCT04731623* |
|
NCT05314439* |
10 |
ION224, IONIS DGAT2Rx |
Ionis |
MASH |
Diacylglycerol acyltransferase 2 (DGAT2) |
Phase II |
NCT04932512* |
Lit.171 |
NCT03334214* |
11 |
AZD2693, ION839, IONIS-AZ6-2.5-LRx |
Ionis†/AstraZeneca‡ |
MASH |
Patatin-like phospholipase domain-containing protein 3 (PNPLA3) |
Phase II |
NCT05809934** |
|
NCT05919069** |
NCT04483947* |
NCT04142424* |
NCT05107336* |
12 |
ION532, IONIS-AZ5-2.5Rx, AZD2373 |
Ionis†/AstraZeneca‡ |
Apolipoprotein L1-associated chronic kidney disease |
Apolipoprotein L1 (APOL1) |
Phase I |
NCT05351047* |
|
NCT04269031* |
Eplontersen
(ION-682884, IONIS-TTR-LRx, AKCEA-TTR-LRx, Akcea/Ionis/AstraZeneca) was the first GalNAc–ASO conjugate to be approved in the USA in December 2023. It is designed to reduce the production of transthyretin to treat all types of TTR.159,161 The dosing schedule involves monthly subcutaneous injections, with no significant accumulation observed following repeated dosing every four weeks.172
Four GalNAc–ASO conjugates are currently evaluated in phase III.
Pelacarsen
(AKCEA-APO)(a)-LRx, AKCEA-APO(a)-LRx, TQJ230, Novartis) is designed to reduce the production of apolipoprotein(a) and is being developed for patients who are at a significant risk of cardiovascular disease because of their elevated Lp(a).163,173–177 Phase III studies Lp(a) HORIZON: NCT04023552, NCT05305664 as well as an open-label extension study (NCT05900141) are ongoing.
Olezarsen
(IONIS-APOCIII-LRx, AKCEA-APOCIII-LRx, Ionis) is designed to inhibit the production of apoC-III to treat hypertriglyceridemia.178 Additionally, it has an orphan drug status for the treatment of familial chylomicronemia syndrome. A number of phase III trials for both conditions are ongoing.
Donidalorsen
(IONIS-PKK-LRx, ISIS 721744, Ionis) is designed to reduce the production of prekallikrein (PKK) to treat patients with hereditary angioedema (phase III trial NCT05392114, phase II trial NCT04307381).165,166
Bepirovirsen
(GSK3389404, IONIS-HBV-LRx, GSK) targets HBV viral proteins to treat chronic HBV and is evaluated in several phase III studies.
Six GalNAc–ASO conjugates are currently in phase II studies and one more in a phase I study.
Conjugates in development aimed at treating diseases affecting the cardiovascular system include fesomersen (BAY2976217, ISIS-FXIRx, ISIS 416858, IONIS-FXI-LRx, Bayer) targeting factor XI to treat thrombosis, ION-904 (Ionis) targeting angiotensinogen for treatment-resistant hypertension and sapablursen (IONIS-TMPRSS6-LRx, Ionis) targeting transmembrane serine protease 6 (TMPRSS6) to treat β-thalassaemia and polycythemia vera. ION224 (IONIS DGAT2Rx) and AZD2693 (ION839, IONIS-AZ6-2.5-LRx, Ionis/AstraZeneca) are in development to treat MASH. While ION224 targets diacylglycerol acyltransferase 2, AZD2693 targets patatin-like phospholipase domain-containing protein 3. IONIS-FB-LRx (Ionis/Roche) targets complement factor to treat IgA nephropathy (IgAN) and age-related macular degeneration. To complete the list of conjugates in development, ION532 (IONIS-AZ5-2.5Rx, AZD2373, Astra Zeneca) is in a phase I study to treat apolipoprotein L1-associated chronic kidney disease.
In addition to the ASO conjugates developed by Ionis, Roche is developing GalNAc3 conjugates with locked nucleic acid (LNA)-containing single stranded oligonucleotides (SSO) for the treatment of chronic HBV.179RG6084 (PD-L1 LNA, RO7191863) that targets PD-L1/PD-1 immune checkpoint inhibitory pathway, which is overexpressed in the liver of patients with chronic hepatitis B, is now in a phase II (NCT04225715) study for a combination HBV therapy.
Other examples of GalNAc conjugates targeted to the liver
Peptide nucleic acids (PNAs) are synthetic DNA analogues in which the deoxyribose phosphate backbone is replaced by a pseudo-peptide structure. This substitution imparts electrical neutrality, enhances chemo-enzymatic stability, and improves target–strand hybridization.180,181 As a result, PNAs hold potential as antisense or antigene drugs. However, PNAs also face significant challenges: they have low cell permeability compared to phosphodiester or phosphorothioate oligonucleotides,181 some sequences exhibit poor water solubility,180 they lack cell-type specificity, and are rapidly cleared from the body.182
To address issues with cell permeability and specificity, PNAs were conjugated to ligands to enhance targeted uptake.181 For example, lactose–PNA conjugates were able to enter cells expressing ASGPR in vitro, though only at high concentrations, while cells lacking this receptor showed no uptake.183 Studies by Van Berkel and Biessen demonstrated that synthetic ASGPR ligands conjugated to PNAs could downregulate human and murine microsomal triglyceride transfer protein (MTP). Targeted PNA was observed to reduce MTP expression in parenchymal liver cells by up to 70%.181,184
Ishihara et al. reported a method for delivering PNAs using AF–DNA conjugates. In this approach, AF is conjugated to a 10-mer DNA strand complementary to the target PNA sequence, allowing the PNA to hybridize with the AF–DNA conjugate. Upon internalization, the PNA is spontaneously released through a strand exchange mechanism. This system enabled successful delivery of PNAs to the liver following intravenous injection, resulting in the inhibition of telomerase.182
A structure–activity relationship (SAR) study by Bhingardeve et al. emphasized the importance of ASGPR ligand architecture in designing ASGPR–PNA conjugates. The study found that a conjugate with GalNAc arranged sequentially achieved 13-fold higher internalization efficiency than the triantennary GalNAc ligand. This sequential design offers a more cost-effective and simpler approach for producing conjugates.185
Aptamers are single-stranded DNA (ssDNA) or RNA (ssRNA) oligonucleotides that fold into defined architectures tertiary structures. They bind with high affinity and specificity to their targets and are non-immunogenic.186 However, the uptake of DNA ONs in cells is problematic. Zamay et al. reported the delivery of aptamers inside cells using arabinogalactan as a carrier. The ASGPR-internalised aptamers inhibited adenocarcinoma growth.187 In another example of ASGPR-targeted aptamers, Zhu et al. reported the construction of lysosome targeting chimeras (LYTACs, vide infra) using an aptamer equipped with a three GalNAc units at the end to target ASGPR. These aptamer-based LYTACs quickly degraded the extracellular proteins through a lysosomal degradation pathway.188
Facile genome engineering using clustered regularly interspaced palindromic repeat (CRISPR) and their associated (Cas) nucleases revolutionized molecular biology. This technique is the state of the art for gene editing.189 Nevertheless, methods for cell- and tissue-selective delivery currently limits its uses. Doudna et al. reported the design of a Cas9 protein bearing a triantennary ASGPR ligand for receptor-mediated genome editing. In vitro tests showed selective gene editing in ASGPR expressing cells. This approach to gene editing needed the assistance of an endosomolytic agent to achieve endosomal escape.190 A patent application for this technology was filed in 2016 by Pfizer Inc. and The Reagents of The University of California.191
Targeted protein degradation of extracellular proteins through ASGPR
Targeted protein degradation (TPD) is an innovative therapeutic strategy that harnesses the cell's natural degradation pathways to selectively eliminate specific proteins. Unlike traditional inhibitors that block protein function, TPD employs heterobifunctional molecules (chimeras) whose one end binds to the protein of interest and the other end directs the complex towards a chosen degradation pathway, either to proteasome after having been labelled with ubiquitin or to the lysosome. For recent reviews on TPD, check references.192,193 The first-generation LYTACs (LYsosome TArgeting Chimeras), developed by Bertozzi targeted mannose-6-phosphate receptor (CI-M6PR) to exploit receptor-mediated endocytosis of extracellular proteins into the lysosome.194
In 2021, the research groups of Bertozzi, Spiegel and Tang independently reported chimeric molecules featuring triantennary GalNAc for targeted protein degradation in the liver.195–198 Bertozzi's second-generation LYTAC, comprising a 3.4 kDa peptide binder linked to a triantennary GalNAc ligand, successfully degraded integrins and reduced cancer cell proliferation.199 Spiegel developed small molecule-based lysosome targeting degraders, termed MoDE-As, that depleted an antibody and a proinflammatory cytokine.196 Tang demonstrated that both small molecule- and antibody-based degraders could induce lysosomal degradation through ASGPR. In addition, he showed that molecular size plays a significant role, with internalization through ASGPR being more efficient for smaller degrader–target protein complexes.197
Wang applied a chemoenzymatic glycan remodelling method to prepare a series of site-specific antibody–ligand conjugates bearing natural bi- and tri-antennary N-glycans as well as synthetic tri-GalNAc ligands. The conjugates were aimed at degradation of EGFR and PCSK9 as representatives of membrane and secreted proteins, respectively.200 Wang et al. also developed amphiphilic peptide-modified GalNAc that can self-assemble into nanospheres and showed that these can degrade various extracellular and membrane proteins by linking them with the relevant antibodies.201
Avilar Therapeutics, a biopharmaceutical company specializing in extracellular protein degradation, has introduced an ATAC platform designed to target pathological proteins using the natural ASGPR protein degradation pathway. ATACs (ASGPR TArgeting Chimeras) are bifunctional molecules composed of an ASGPR-binding ligand linked to a ligand targeting a disease-causing extracellular protein. The chemical nature of the ASGPR ligand used in ATACs has not been disclosed. The company synthesised hundreds of monosaccharide-based ASGPR ligands, with over 100 having KD ≤ 1000 nM and about 40 having KD ≤ 100 nM. Additionally, they have over 20 X-ray structures of ASGPR–ligand complexes.202 The selected ASGPR ligand boasts approximately 2000-fold higher affinity than GalNAc and significantly higher affinity (>60-fold) than the bicyclic bridged ketal 9. In contrast to earlier compounds that featured trivalent GalNAc ligands linked to an antibody,203 ATACs utilize bi- and mono-dentate ligands connected to a peptide or small molecule.204 Initial studies targeting IgG and TNFα demonstrated successful in vitro binding, ternary complex formation, cellular uptake, and target protein degradation.
Conclusion and future perspectives
In conclusion, targeting hepatocytes via the asialoglycoprotein receptor represents a promising strategy for liver-specific drug delivery, especially in the field of RNA interference and gene editing. Delivery of therapeutic nucleic acids such as siRNAs and ASOs demonstrate significant advancements with six candidates already on the market and a number of compounds in clinical development. We can expect expanding the therapeutic applications to treat more genetic liver diseases. Additionally, targeting viral genetic material in hepatocytes is a promising strategy for treating chronic hepatitis B and potentially also hepatitis D. We may also anticipate expansion beyond RNAi and ASOs to include conjugates with PNAs or aptamers, as well as ASGPR-targeted nanocarriers for CRISPR-Cas. Furthermore, targeted protein degradation technologies, such as LYTACs and ATACs, offer innovative approaches for therapeutic intervention. Finally, although there are still significant challenges, research into oral formulations for GalNAc conjugates is ongoing.205
Data availability
No primary research results, software or code have been included and no new data were generated or analysed as part of this review.
Conflicts of interest
There are no conflicts of interest to declare.
Acknowledgements
This work has been supported by the Max Planck Society through their Partner Group programme.
References
-
P. K. Grewal, in Methods in Enzymology, ed. M. Fukuda, Academic Press, 2010, vol. 479, pp. 223–241 Search PubMed.
- K. Drickamer, Curr. Opin. Struct. Biol., 1999, 9, 585–590 CrossRef CAS.
- G. Ashwell and J. Harford, Annu. Rev. Biochem., 1982, 51, 531–554 CrossRef CAS PubMed.
- M. R. Hardy, R. R. Townsend, S. M. Parkhurst and Y. C. Lee, Biochemistry, 1985, 24, 22–28 CrossRef CAS.
- P. K. Grewal, S. Uchiyama, D. Ditto, N. Varki, D. T. Le, V. Nizet and J. D. Marth, Nat. Med., 2008, 14, 648–655 CrossRef CAS PubMed.
- E. I. Rigopoulou, D. Roggenbuck, D. S. Smyk, C. Liaskos, M. G. Mytilinaiou, E. Feist, K. Conrad and D. P. Bogdanos, Autoimmun. Rev., 2012, 12, 260–269 CrossRef CAS.
- M. Spiess and H. F. Lodish, Proc. Natl. Acad. Sci. U. S. A., 1985, 82, 6465–6469 CrossRef CAS PubMed.
- M. Spiess, A. L. Schwartz and H. F. Lodish, J. Biol. Chem., 1985, 260, 1979–1982 CrossRef CAS.
- Y. I. Henis, Z. Katzir, M. A. Shia and H. F. Lodish, J. Cell Biol., 1990, 111, 1409–1418 CrossRef CAS.
- J. Bischoff and H. F. Lodish, J. Biol. Chem., 1987, 262, 11825–11832 CrossRef CAS.
- R. J. Stockert, Physiol. Rev., 1995, 75, 591–609 CrossRef CAS.
- A. A. D'Souza and P. V. Devarajan, J. Controlled Release, 2015, 203, 126–139 CrossRef.
- J.-H. Park, E.-W. Cho, S. Y. Shin, Y.-J. Lee and K. L. Kim, Biochem. Biophys. Res. Commun., 1998, 244, 304–311 CrossRef CAS.
- M. Spiess, Biochemistry, 1990, 29, 10009–10018 CrossRef CAS PubMed.
- P. H. Weigel and J. A. Oka, J. Biol. Chem., 1983, 258, 5095–5102 CrossRef CAS.
- A. L. Schwartz, S. E. Fridovich and H. F. Lodish, J. Biol. Chem., 1982, 257, 4230–4237 CrossRef CAS.
-
I. Geffen and M. Spiess, in International Review of Cytology, ed. M. Friedlander and M. Mueckler, Academic Press, 1993, vol. 137, pp. 181–219 Search PubMed.
- L. Singh, S. Indermun, M. Govender, P. Kumar, L. C. Du Toit, Y. E. Choonara and V. Pillay, Viruses, 2018, 10, 267 CrossRef PubMed.
- M. H. Elberry, N. H. E. Darwish and S. A. Mousa, Virol. J., 2017, 14, 88 CrossRef.
- R. Dutta and R. I. Mahato, Pharmacol. Ther., 2017, 173, 106–117 CrossRef CAS PubMed.
- S. Borrmann and K. Matuschewski, Trends Mol. Med., 2011, 17, 527–536 CrossRef CAS PubMed.
- S. K. Mamidyala, S. Dutta, B. A. Chrunyk, C. Préville, H. Wang, J. M. Withka, A. McColl, T. A. Subashi, S. J. Hawrylik, M. C. Griffor, S. Kim, J. A. Pfefferkorn, D. A. Price, E. Menhaji-Klotz, V. Mascitti and M. G. Finn, J. Am. Chem. Soc., 2012, 134, 1978–1981 CAS.
- A. R. Kolatkar and W. I. Weis, J. Biol. Chem., 1996, 271, 6679–6685 CAS.
- N. I. Ruiz and K. Drickamer, Glycobiology, 1996, 6, 551–559 CAS.
- Y. C. Lee, FASEB J., 1992, 6, 3193–3200 CrossRef CAS PubMed.
- D. Stokmaier, O. Khorev, B. Cutting, R. Born, D. Ricklin, T. O. G. Ernst, F. Böni, K. Schwingruber, M. Gentner, M. Wittwer, M. Spreafico, A. Vedani, S. Rabbani, O. Schwardt and B. Ernst, Bioorg. Med. Chem., 2009, 17, 7254–7264 CAS.
- A. R. Kolatkar, A. K. Leung, R. Isecke, R. Brossmer, K. Drickamer and W. I. Weis, J. Biol. Chem., 1998, 273, 19502–19508 CAS.
- Y. C. Lee, R. R. Townsend, M. R. Hardy, J. Lönngren, J. Arnarp, M. Haraldsson and H. Lönn, J. Biol. Chem., 1983, 258, 199–202 CAS.
- D. T. Connolly, R. R. Townsend, K. Kawaguchi, W. R. Bell and Y. C. Lee, J. Biol. Chem., 1982, 257, 939–945 CrossRef CAS.
- R. T. Lee and Y. C. Lee, Glycoconjugate J., 2000, 17, 543–551 CrossRef CAS PubMed.
- E. A. L. Biessen, D. M. Beuting, H. C. P. F. Roelen, G. A. van de Marel, J. H. Van Boom and T. J. C. Van Berkel, J. Med. Chem., 1995, 38, 1538–1546 CrossRef CAS PubMed.
- J. U. Baenziger and D. Fiete, Cell, 1980, 22, 611–620 CrossRef CAS.
- M. Monestier, P. Charbonnier, C. Gateau, M. Cuillel, F. Robert, C. Lebrun, E. Mintz, O. Renaudet and P. Delangle, ChemBioChem, 2016, 17, 590–594 CrossRef CAS.
-
G. Ashwell and A. G. Morell, in Advances in Enzymology and Related Areas of Molecular Biology, 1974, pp. 99–128, DOI:10.1002/9780470122860.ch3.
- Y. C. Lee, R. R. Townsend, M. R. Hardy, J. Lönngren, J. Arnarp, M. Haraldsson and H. Lönn, J. Biol. Chem., 1983, 258, 199–202 CrossRef CAS.
- D. U. Warrier, A. K. Dhanabalan, G. Krishnasamy, H. Kolge, V. Ghormade, C. R. Gupta, P. K. Ambre and U. A. Shinde, Int. J. Biol. Macromol., 2022, 207, 683–699 CrossRef CAS.
- T. Tanaka, Y. Fujishima, S. Hamano and Y. Kaneo, Eur. J. Pharm. Sci., 2004, 22, 435–444 CrossRef CAS PubMed.
- Y. Kaneo, T. Tanaka, T. Nakano and Y. Yamaguchi, J. Controlled Release, 2001, 70, 365–373 CrossRef CAS PubMed.
- C. A. Sanhueza, M. M. Baksh, B. Thuma, M. D. Roy, S. Dutta, C. Préville, B. A. Chrunyk, K. Beaumont, R. Dullea, M. Ammirati, S. Liu, D. Gebhard, J. E. Finley, C. T. Salatto, A. King-Ahmad, I. Stock, K. Atkinson, B. Reidich, W. Lin, R. Kumar, M. Tu, E. Menhaji-Klotz, D. A. Price, S. Liras, M. G. Finn and V. Mascitti, J. Am. Chem. Soc., 2017, 139, 3528–3536 CrossRef CAS PubMed.
-
S. Liras, V. Mascitti and B. Thuma, US Pat., US9340553B2, 2016 Search PubMed.
-
D. Spiegel and D. Caianiello, US Pat., US11767301B2, 2019 Search PubMed.
-
M. G. Saulnier, J. J. Chen, S. Karra, K. T. Sprott, J. A. Wiles and S. Ray, US Pat., US11819551B2, 2021 Search PubMed.
-
M. Deshpande, M. G. Saulnier, K. T. Sprott, J. J. Chen, S. Ray and J. A. Wiles, WO Pat., WO2022035997A1, 2021 Search PubMed.
-
J. A. Wiles, S. Karra, M. G. Saulnier, J. J. Chen, K. T. Sprott and S. Ray, CA Pat., CA3174145A1, 2022 Search PubMed.
- X. Huang, J.-C. Leroux and B. Castagner, Bioconjugate Chem., 2017, 28, 283–295 CrossRef CAS.
- M. Gonçalves, S. Mignani, J. Rodrigues and H. Tomás, J. Controlled Release, 2020, 317, 347–374 CrossRef.
- M. Hashida, M. Nishikawa, F. Yamashita and Y. Takakura, Adv. Drug Delivery Rev., 2001, 52, 187–196 CrossRef CAS PubMed.
- K. Akamatsu, M. Imai, Y. Yamasaki, M. Nishikawa, Y. Takakura and M. Hashida, J. Drug Targeting, 1998, 6, 229–239 CrossRef CAS.
- S. Wang, L. Cheng, F. Yu, W. Pan and J. Zhang, Int. J. Pharm., 2006, 311, 82–88 CrossRef CAS PubMed.
- P. J. Julyan, L. W. Seymour, D. R. Ferry, S. Daryani, C. M. Boivin, J. Doran, M. David, D. Anderson, C. Christodoulou, A. M. Young, S. Hesslewood and D. J. Kerr, J. Controlled Release, 1999, 57, 281–290 CrossRef CAS PubMed.
- L. W. Seymour, D. R. Ferry, D. Anderson, S. Hesslewood, P. J. Julyan, R. Poyner, J. Doran, A. M. Young, S. Burtles and D. J. Kerr, J. Clin. Oncol., 2002, 20, 1668–1676 CrossRef CAS.
- R. Duncan, Adv. Drug Delivery Rev., 2009, 61, 1131–1148 CrossRef CAS.
- Y. Zou, Y. Song, W. Yang, F. Meng, H. Liu and Z. Zhong, J. Controlled Release, 2014, 193, 154–161 CrossRef CAS PubMed.
- E. F. Craparo, D. Triolo, G. Pitarresi, G. Giammona and G. Cavallaro, Biomacromolecules, 2013, 14, 1838–1849 CrossRef CAS.
- S. H. Medina, V. Tekumalla, M. V. Chevliakov, D. S. Shewach, W. D. Ensminger and M. E. H. El-Sayed, Biomaterials, 2011, 32, 4118–4129 CrossRef CAS PubMed.
- Y. Hayashi, T. Higashi, K. Motoyama, Y. Mori, H. Jono, Y. Ando and H. Arima, J. Drug Targeting, 2013, 21, 487–496 CrossRef CAS PubMed.
- G. Zheng, R. Zhao, A. Xu, Z. Shen, X. Chen and J. Shao, Eur. J. Pharm. Sci., 2018, 111, 492–502 Search PubMed.
- S. Pranatharthiharan, M. D. Patel, V. C. Malshe, V. Pujari, A. Gorakshakar, M. Madkaikar, K. Ghosh and P. V. Devarajan, Drug Delivery, 2017, 24, 20–29 CrossRef CAS.
- R. A. Petrov, S. Y. Maklakova, Y. A. Ivanenkov, S. A. Petrov, O. V. Sergeeva, E. Y. Yamansarov, I. V. Saltykova, I. I. Kireev, I. B. Alieva, E. V. Deyneka, A. A. Sofronova, A. V. Aladinskaia, A. V. Trofimenko, R. S. Yamidanov, S. V. Kovalev, V. E. Kotelianski, T. S. Zatsepin, E. K. Beloglazkina and A. G. Majouga, Bioorg. Med. Chem. Lett., 2018, 28, 382–387 CrossRef CAS PubMed.
- Y. A. Ivanenkov, A. G. Majouga, R. A. Petrov, S. A. Petrov, S. V. Kovalev, S. Y. Maklakova, E. Y. Yamansarov, I. V. Saltykova, E. V. Deyneka, G. I. Filkov, V. E. Kotelianski, T. S. Zatsepin and E. K. Beloglazkina, Bioorg. Med. Chem. Lett., 2018, 28, 503–508 CrossRef CAS.
- R. A. Petrov, S. R. Mefedova, E. Y. Yamansarov, S. Y. Maklakova, D. A. Grishin, E. V. Lopatukhina, O. Y. Burenina, A. V. Lopukhov, S. V. Kovalev, Y. V. Timchenko, E. E. Ondar, Y. A. Ivanenkov, S. A. Evteev, A. N. Vaneev, R. V. Timoshenko, N. L. Klyachko, A. S. Erofeev, P. V. Gorelkin, E. K. Beloglazkina and A. G. Majouga, Mol. Pharmaceutics, 2021, 18, 461–468 CrossRef CAS PubMed.
- L. Rico, M. E. Østergaard, M. Bell, P. P. Seth and S. Hanessian, Bioorg. Med. Chem. Lett., 2018, 28, 2652–2654 CrossRef CAS.
- Y. Hua and C. Yu, Eur. J. Med. Chem., 2024, 269, 116278 CrossRef CAS.
- A. Werner, M. Freesmeyer, C. Kühnel, R. Drescher and J. Greiser, Diagnostics, 2023, 13, 1144 CrossRef CAS PubMed.
- H. Cui, X. Zhu, S. Li, P. Wang and J. Fang, ACS Omega, 2021, 6, 16259–16265 CrossRef CAS PubMed.
- C. F. Bennett, Annu. Rev. Med., 2019, 70, 307–321 CrossRef CAS PubMed.
- J. H. Chan, S. Lim and W. F. Wong, Clin. Exp. Pharmacol. Physiol., 2006, 33, 533–540 Search PubMed.
- P. D. Zamore, T. Tuschl, P. A. Sharp and D. P. Bartel, Cell, 2000, 101, 25–33 Search PubMed.
- R. Kanasty, J. R. Dorkin, A. Vegas and D. Anderson, Nat. Mater., 2013, 12, 967–977 CrossRef CAS PubMed.
- J. K. Nair, J. L. S. Willoughby, A. Chan, K. Charisse, M. R. Alam, Q. Wang, M. Hoekstra, P. Kandasamy, A. V. Kel'in, S. Milstein, N. Taneja, J. O'Shea, S. Shaikh, L. Zhang, R. J. van der Sluis, M. E. Jung, A. Akinc, R. Hutabarat, S. Kuchimanchi, K. Fitzgerald, T. Zimmermann, T. J. C. van Berkel, M. A. Maier, K. G. Rajeev and M. Manoharan, J. Am. Chem. Soc., 2014, 136, 16958–16961 Search PubMed.
- J. K. Nair, H. Attarwala, A. Sehgal, Q. Wang, K. Aluri, X. Zhang, M. Gao, J. Liu, R. Indrakanti, S. Schofield, P. Kretschmer, C. R. Brown, S. Gupta, J. L. S. Willoughby, J. A. Boshar, V. Jadhav, K. Charisse, T. Zimmermann, K. Fitzgerald, M. Manoharan, K. G. Rajeev, A. Akinc, R. Hutabarat and M. A. Maier, Nucleic Acids Res., 2017, 45, 10969–10977 Search PubMed.
- M. R. Hassler, A. A. Turanov, J. F. Alterman, R. A. Haraszti, A. H. Coles, M. F. Osborn, D. Echeverria, M. Nikan, W. E. Salomon, L. Roux, B. Godinho, S. M. Davis, D. V. Morrissey, P. D. Zamore, S. A. Karumanchi, M. J. Moore, N. Aronin and A. Khvorova, Nucleic Acids Res., 2018, 46, 2185–2196 CAS.
- M. K. Schlegel, D. J. Foster, A. V. Kel'in, I. Zlatev, A. Bisbe, M. Jayaraman, J. G. Lackey, K. G. Rajeev, K. Charissé, J. Harp, P. S. Pallan, M. A. Maier, M. Egli and M. Manoharan, J. Am. Chem. Soc., 2017, 139, 8537–8546 Search PubMed.
- M. M. Janas, M. K. Schlegel, C. E. Harbison, V. O. Yilmaz, Y. Jiang, R. Parmar, I. Zlatev, A. Castoreno, H. Xu, S. Shulga-Morskaya, K. G. Rajeev, M. Manoharan, N. D. Keirstead, M. A. Maier and V. Jadhav, Nat. Commun., 2018, 9, 723 Search PubMed.
- A. J. Debacker, J. Voutila, M. Catley, D. Blakey and N. Habib, Mol. Ther., 2020, 28, 1759–1771 CAS.
- M. Egli, M. K. Schlegel and M. Manoharan, RNA, 2023, 29, 402–414 CrossRef CAS PubMed.
- B. Hu, L. Zhong, Y. Weng, L. Peng, Y. Huang, Y. Zhao and X.-J. Liang, Signal Transduction Targeted Ther., 2020, 5, 101 CrossRef CAS.
- S. Leusmann, P. Ménová, E. Shanin, A. Titz and C. Rademacher, Chem. Soc. Rev., 2023, 52, 3663–3740 RSC.
-
Z. Li, R. Zhu, C. I. Wooddell, B. D. Given, T. Pei, D. L. Lewis, L. J. Almeida, D. B. Rozema and D. H. Wakefield, WO Pat., WO2018027106A2, 2016 Search PubMed.
- F. Guo, Y. Li, W. Yu, Y. Fu, J. Zhang and H. Cao, Mol. Pharmaceutics, 2024, 21, 2081–2096 CrossRef CAS PubMed.
-
D. K. Lee, US Pat., US20130273657A1, 2013 Search PubMed.
- Taking RNAi under the skin, https://www.nature.com/articles/d43747-020-00189-y.pdf, accessed July 2024).
-
D. Samarsky, EP Pat., EP3844279A2, 2019 Search PubMed.
- GalAhead™ Platform & Programs, https://sirnaomics.com/media/v5an4hex/galahead-a-novel-therapeutic-galnac-rnai-platform-to-downregulate-single-and-multiple-genes.pdf, accessed July 2024).
- A. Weingärtner, L. Bethge, L. Weiss, M. Sternberger and M. W. Lindholm, Mol. Ther.--Nucleic Acids, 2020, 21, 242–250 CrossRef PubMed.
-
C. Frauendorf and M. Cameron, WO Pat., WO2017174657A1, 2016 Search PubMed.
-
H. Zhang, Z. Yang, L. Cao and L. Wan, US Pat., US20200338201A1, 2020 Search PubMed.
- L. Sandra, H. T'Jollyn, N. Goeyvaerts, A. Vermeulen, A. G. Dosne and J. J. Perez-Ruixo, J. Pharmacol. Exp. Ther., 2022, 383, 70–79 Search PubMed.
- X. Jing, V. Arya, K. S. Reynolds and H. Rogers, Drug Metab. Dispos., 2023, 51, 193–198, DOI:10.1124/dmd.122.001107.
- R. McDougall, D. Ramsden, S. Agarwal, S. Agarwal, K. Aluri, M. Arciprete, C. Brown, E. Castellanos-Rizaldos, K. Charisse, S. Chong, J. Cichocki, K. Fitzgerald, V. Goel, Y. Gu, D. Guenther, B. Habtemariam, V. Jadhav, M. Janas, M. Jayaraman, J. Kurz, J. Li, J. Liu, X. Liu, S. Liou, C. Maclauchlin, M. Maier, M. Manoharan, J. K. Nair, G. Robbie, K. Schmidt, P. Smith, C. Theile, A. Vaishnaw, S. Waldron, Y. Xu, X. Zhang, I. Zlatev and J. T. Wu, Drug Metab. Dispos., 2022, 50, 781–797 CrossRef CAS.
- G. An, J. Clin. Pharmacol., 2024, 64, 45–57 CrossRef CAS PubMed.
- V. S. Ayyar and D. Song, J. Pharm. Sci., 2024, 113, 176–190 Search PubMed.
- C. R. Brown, S. Gupta, J. Qin, T. Racie, G. He, S. Lentini, R. Malone, M. Yu, S. Matsuda, S. Shulga-Morskaya, A. V. Nair, C. S. Theile, K. Schmidt, A. Shahraz, V. Goel, R. G. Parmar, I. Zlatev, M. K. Schlegel, J. K. Nair, M. Jayaraman, M. Manoharan, D. Brown, M. A. Maier and V. Jadhav, Nucleic Acids Res., 2020, 48, 11827–11844 CAS.
- J. Belgrad, H. H. Fakih and A. Khvorova, Nucleic Acid Ther., 2024, 34, 52–72 Search PubMed.
- E. A. Narasipura, R. VanKeulen-Miller, Y. Ma and O. S. Fenton, Bioconjugate Chem., 2023, 34, 1177–1197 CAS.
- Y. Chen, Y. Li, C. Li, D. Zhang, Y. Liu, J. Zhang, S. Guan, X. Ding and Q. Xiao, Drug Dev. Res., 2024, 85, e22164 CAS.
- M. Balwani, E. Sardh, P. Ventura, P. A. Peiró, D. C. Rees, U. Stölzel, D. M. Bissell, H. L. Bonkovsky, J. Windyga, K. E. Anderson, C. Parker, S. M. Silver, S. B. Keel, J.-D. Wang, P. E. Stein, P. Harper, D. Vassiliou, B. Wang, J. Phillips, A. Ivanova, J. G. Langendonk, R. Kauppinen, E. Minder, Y. Horie, C. Penz, J. Chen, S. Liu, J. J. Ko, M. T. Sweetser, P. Garg, A. Vaishnaw, J. B. Kim, A. R. Simon and L. Gouya, N. Engl. J. Med., 2020, 382, 2289–2301 CrossRef CAS PubMed.
- Y. Y. Syed, Drugs, 2021, 81, 841–848 Search PubMed.
- B. Wang, P. Ventura, K. I. Takase, M. Thapar, D. Cassiman, I. Kubisch, S. Liu, M. T. Sweetser and M. Balwani, Orphanet J. Rare Dis., 2022, 17, 327 Search PubMed.
- R. Marchi, L. Duarte, S. P, R. D, A. Ke, B. Hl, S. E, H. P, B. M and E. Al, Hematol. Transfus. Cell Ther., 2020, 42, 17–18 CrossRef.
- S. F. Garrelfs, Y. Frishberg, S. A. Hulton, M. J. Koren, W. D. O'Riordan, P. Cochat, G. Deschênes, H. Shasha-Lavsky, J. M. Saland, W. G. van't Hoff, D. G. Fuster, D. Magen, S. H. Moochhala, G. Schalk, E. Simkova, J. W. Groothoff, D. J. Sas, K. A. Meliambro, J. Lu, M. T. Sweetser, P. P. Garg, A. K. Vaishnaw, J. M. Gansner, T. L. McGregor and J. C. Lieske, N. Engl. J. Med., 2021, 384, 1216–1226 CrossRef CAS PubMed.
- F. Erger and B. B. Beck, Nat. Rev. Nephrol., 2021, 17, 573–574 CrossRef CAS PubMed.
- W. Hayes, D. J. Sas, D. Magen, H. Shasha-Lavsky, M. Michael, A. L. Sellier-Leclerc, J. Hogan, T. Ngo, M. T. Sweetser, J. M. Gansner, T. L. McGregor and Y. Frishberg, Pediatr. Nephrol., 2023, 38, 1075–1086 CrossRef PubMed.
- M. Michael, J. W. Groothoff, H. Shasha-Lavsky, J. C. Lieske, Y. Frishberg, E. Simkova, A. L. Sellier-Leclerc, A. Devresse, F. Guebre-Egziabher, S. A. Bakkaloglu, C. Mourani, R. Saqan, R. Singer, R. Willey, B. Habtemariam, J. M. Gansner, I. Bhan, T. McGregor and D. Magen, Am. J. Kidney Dis., 2023, 81, 145–155 CrossRef CAS PubMed , e141.
- D. J. Sas, D. Magen, W. Hayes, H. Shasha-Lavsky, M. Michael, I. Schulte, A. L. Sellier-Leclerc, J. Lu, A. Seddighzadeh, B. Habtemariam, T. L. McGregor, K. P. Fujita and Y. Frishberg, Genet. Med., 2022, 24, 654–662 CrossRef CAS PubMed.
- Y. Frishberg, G. Deschênes, J. W. Groothoff, S.-A. Hulton, D. Magen, J. Harambat, W. G. van't Hoff, U. Lorch, D. S. Milliner, J. C. Lieske, P. Haslett, P. P. Garg, A. K. Vaishnaw, S. Talamudupula, J. Lu, B. A. Habtemariam, D. V. Erbe, T. L. McGregor and P. Cochat, Clin. J. Am. Soc. Nephrol., 2021, 16, 1025–1036 CrossRef CAS PubMed.
- F. J. Raal, D. Kallend, K. K. Ray, T. Turner, W. Koenig, R. S. Wright, P. L. J. Wijngaard, D. Curcio, M. J. Jaros, L. A. Leiter and J. J. P. Kastelein, N. Engl. J. Med., 2020, 382, 1520–1530 CrossRef CAS PubMed.
- K. K. Ray, R. S. Wright, D. Kallend, W. Koenig, L. A. Leiter, F. J. Raal, J. A. Bisch, T. Richardson, M. Jaros, P. L. J. Wijngaard and J. J. P. Kastelein, N. Engl. J. Med., 2020, 382, 1507–1519 CrossRef CAS.
- Y. N. Lamb, Drugs, 2021, 81, 389–395 CrossRef CAS PubMed.
- B. A. Habtemariam, V. Karsten, H. Attarwala, V. Goel, M. Melch, V. A. Clausen, P. Garg, A. K. Vaishnaw, M. T. Sweetser, G. J. Robbie and J. Vest, Clin. Pharmacol. Ther., 2021, 109, 372–382 CAS.
- B. Hoppe, A. Koch, P. Cochat, S. F. Garrelfs, M. A. Baum, J. W. Groothoff, G. Lipkin, M. Coenen, G. Schalk, A. Amrite, D. McDougall, K. Barrios and C. B. Langman, Kidney Int., 2022, 101, 626–634 CAS.
- Y. Y. Syed, Drugs, 2023, 83, 1729–1733 CrossRef CAS PubMed.
- K. J. Pasi, T. Lissitchkov, V. Mamonov, T. Mant, M. Timofeeva, C. Bagot, P. Chowdary, P. Georgiev, L. Gercheva-Kyuchukova, K. Madigan, H. Van Nguyen, Q. Yu, B. Mei, C. C. Benson and M. V. Ragni, J. Thromb. Haemostasis, 2021, 19, 1436–1446 CAS.
- M. V. Ragni, P. Georgiev, T. Mant, M. D. Creagh, T. Lissitchkov, D. Bevan, S. Austin, C. R. Hay, I. Hegemann, R. Kazmi, P. Chowdary, S. Rangarajan, C.-H. Soh, A. Akinc, A. M. Partisano, B. Sorenson and K. J. Pasi, Blood, 2016, 128, 2572 CrossRef.
- P. Badri, X. Jiang, A. Borodovsky, N. Najafian, J. Kim, V. A. Clausen, V. Goel, B. Habtemariam and G. J. Robbie, Clin. Pharmacokinet., 2021, 60, 365–378 CrossRef CAS PubMed.
- C. Schwabe, Eur. Heart J., 2020, 41, 3330 CrossRef.
- P. Clifton, D. Sullivan, J. Baker, C. Schwabe, S. Thackwray, R. Scott, J. Hamilton, A. J. L. Pineda, B. Given, S. Melquist, I. Chen, J. S. Martin, G. F. Watts, I. J. Goldberg, J. Knowles, D. Gaudet, R. A. Hegele and C. M. Ballantyne, Circulation, 2021, 144, A10357 Search PubMed.
- C. M. Ballantyne, S. Vasas, M. Azizad, P. Clifton, R. S. Rosenson, T. Chang, S. Melquist, R. Zhou, M. A. Mushin, N. J. Leeper, J. Hellawell and D. Gaudet, N. Engl. J. Med., 2024, 391(10), 899–912 CAS.
- D. Gaudet, D. Pall, G. F. Watts, S. J. Nicholls, R. S. Rosenson, K. Modesto, J. San Martin, J. Hellawell and C. M. Ballantyne, JAMA Cardiol., 2024, 9, 620–630 Search PubMed.
- M. J. Koren, P. M. Moriarty, S. J. Baum, J. Neutel, M. Hernandez-Illas, H. S. Weintraub, M. Florio, H. Kassahun, S. Melquist, T. Varrieur, S. M. Haldar, W. Sohn, H. Wang, M. Elliott-Davey, B. M. Rock, T. Pei, O. Homann, J. Hellawell and G. F. Watts, Nat. Med., 2022, 28, 96–103 CrossRef CAS PubMed.
- M. L. O'Donoghue, R. S. Rosenson, B. Gencer, J. A. G. López, N. E. Lepor, S. J. Baum, E. Stout, D. Gaudet, B. Knusel, J. F. Kuder, X. Ran, S. A. Murphy, H. Wang, Y. Wu, H. Kassahun and M. S. Sabatine, N. Engl. J. Med., 2022, 387, 1855–1864 CrossRef PubMed.
- M. L. O'Donoghue, J. A. G. López, B. Knusel, B. Gencer, H. Wang, Y. Wu, H. Kassahun and M. S. Sabatine, Am. Heart J., 2022, 251, 61–69 CrossRef.
- C. I. Wooddell, K. Blomenkamp, R. M. Peterson, V. M. Subbotin, C. Schwabe, J. Hamilton, Q. Chu, D. R. Christianson, J. O. Hegge, J. Kolbe, H. L. Hamilton, M. F. Branca-Afrazi, B. D. Given, D. L. Lewis, E. Gane, S. B. Kanner and J. H. Teckman, JCI Insight, 2020, 5, e135348 CrossRef PubMed.
- A. M. Turner, J. Stolk, R. Bals, J. D. Lickliter, J. Hamilton, D. R. Christianson, B. D. Given, J. G. Burdon, R. Loomba, J. K. Stoller and J. H. Teckman, J. Hepatol., 2018, 69, 378–384 CrossRef CAS PubMed.
- P. Strnad, M. Mandorfer, G. Choudhury, W. Griffiths, C. Trautwein, R. Loomba, T. Schluep, T. Chang, M. Yi, B. D. Given, J. C. Hamilton, J. S. Martin and J. H. Teckman, N. Engl. J. Med., 2022, 387, 514–524 CrossRef CAS.
- S. E. Nissen, H. Linnebjerg, X. Shen, K. Wolski, X. Ma, S. Lim, L. F. Michael, G. Ruotolo, G. Gribble, A. M. Navar and S. J. Nicholls, JAMA, J. Am. Med. Assoc., 2023, 330, 2075–2083 CrossRef.
- T. N. Kakuda, A. Halabi, G. Klein, M. Sanga, C. Guinard-Azadian, M. Kowalik, K. Nedoschinsky, J. Nangosyah, E. N. Ediage, V. Hillewaert, P. Verboven, I. Goris, J. Snoeys, M. Palmer and M. Biermer, J. Clin. Pharmacol., 2023, 63, 732–741 Search PubMed.
- H. Li, X. Niu, Y. Zhang, D. Zhang, Y. Zhang, L. Wang, Y. Miao, Y. Jiang, J. Ji, Q. Chen, X. Wu, E. N. Ediage, T. N. Kakuda and M. Biermer, Clin. Pharmacol. Drug Dev., 2023, 12, 175–180 Search PubMed.
- E. Gane, M. F. Yuen, T. N. Kakuda, T. Ogawa, Y. Takahashi, N. Goeyvaerts, I. Lonjon-Domanec, T. Vaughan, T. Schluep, J. Hamilton, E. Njumbe Ediage, V. Hillewaert, J. Snoeys, O. Lenz, W. Talloen and M. Biermer, Antiviral Ther., 2022, 27, 13596535221093856 CAS.
- S. V. Gupta, M. C. Fanget, C. MacLauchlin, V. A. Clausen, J. Li, D. Cloutier, L. Shen, G. J. Robbie and E. Mogalian, Drugs R&D, 2021, 21, 455–465 CAS.
- S. E. Nissen, K. Wolski, C. Balog, D. I. Swerdlow, A. C. Scrimgeour, C. Rambaran, R. J. Wilson, M. Boyce, K. K. Ray, L. Cho, G. F. Watts, M. Koren, T. Turner, E. S. Stroes, C. Melgaard and G. V. Campion, JAMA, J. Am. Med. Assoc., 2022, 327, 1679–1687 CAS.
- D. A. Rider, M. Eisermann, K. Löffler, M. Aleku, D. I. Swerdlow, S. Dames, J. Hauptmann, E. Morrison, M. W. Lindholm, S. Schubert and G. Campion, Atherosclerosis, 2022, 349, 240–247 Search PubMed.
- G. F. Watts, C. Schwabe, R. Scott, P. Gladding, D. Sullivan, J. Baker, P. Clifton, J. Hamilton, B. Given, J. San Martin, S. Melquist, J. W. Knowles, I. Goldberg, R. Hegele and C. Ballantyne, Eur. Heart J., 2020, 41, ehaa946.3331 Search PubMed.
- G. F. Watts, C. Schwabe, R. Scott, P. Gladding, D. Sullivan, J. Baker, P. Clifton, J. Hamilton, B. Given, J. S. Martin, S. Melquist, T. Chang, N. Rajicic, I. J. Goldberg, D. Gaudet, J. W. Knowles, R. A. Hegele and C. M. Ballantyne, Circulation, 2020, 142, A15751 Search PubMed.
- G. Watts, P. Gladding, C. Schwabe, R. Scott, P. Clifton, D. Sullivan, J. Baker, J. Hamilton, B. Given, S. Melquist, J. S. Martin, J. Knowles, I. Goldberg, D. Gaudet, R. Hegele and C. Ballantyne, J. Clin. Lipidol., 2020, 14, 599 Search PubMed.
- R. S. Rosenson, D. Gaudet, R. A. Hegele, C. M. Ballantyne, S. J. Nicholls, K. J. Lucas, J. S. Martin, R. Zhou, M. A. Muhsin, T. Chang, J. Hellawell and G. F. Watts, N. Engl. J. Med., 2024, 391(10), 913–925 CAS.
- G. F. Watts, C. Schwabe, R. Scott, P. A. Gladding, D. Sullivan, J. Baker, P. Clifton, J. Hamilton, B. Given, S. Melquist, R. Zhou, T. Chang, J. San Martin, D. Gaudet, I. J. Goldberg, J. W. Knowles, R. A. Hegele and C. M. Ballantyne, Nat. Med., 2023, 29, 2216–2223 CAS.
- S. Huang, J. Taubel, S. Casey, P. M. Leung, D. J. Webb, A. S. Desai, Y. Cheng, S. Rhyee, J. Harrop, B. Habtemariam and G. L. Bakris, Circulation, 2021, 144, A10974 Search PubMed.
- A. S. Desai, D. J. Webb, J. Taubel, S. Casey, Y. Cheng, G. J. Robbie, D. Foster, S. A. Huang, S. Rhyee, M. T. Sweetser and G. L. Bakris, N. Engl. J. Med., 2023, 389, 228–238 CrossRef CAS.
- L. Y. Mak, E. Gane, C. Schwabe, K. T. Yoon, J. Heo, R. Scott, J. H. Lee, J. I. Lee, Y. O. Kweon, M. Weltman, S. A. Harrison, B. A. Neuschwander-Tetri, K. Cusi, R. Loomba, B. D. Given, D. R. Christianson, E. Garcia-Medel, M. Yi, J. Hamilton and M. F. Yuen, J. Hepatol., 2023, 78, 684–692 CrossRef CAS.
- S. Altamura, M. U. Muckenthaler, S. Dames, C. Frauendorf, S. Schubert, M. Aleku, J. Vadolas, G. Grigoriadis and U. Zügel, Blood, 2018, 132, 2340 CrossRef.
- S. Altamura, U. Schaeper, S. Dames, K. Löffler, M. Eisermann, C. Frauendorf, K. Müdder, J. Neves and M. U. Muckenthaler, Hemasphere, 2019, 3, e301 CrossRef PubMed.
- J. B. Porter, A. Scrimgeour, A. Martinez, L. James, M. Aleku, R. Wilson, M. Muckenthaler, M. Boyce, D. Wilkes, U. Schaeper and G. V. Campion, Am. J. Hematol., 2023, 98, 1425–1435 CrossRef CAS.
- Innovent and SanegeneBio Announce First Participant Dosed in a Phase 1 Clinical Trial of IBI3016 (AGT siRNA), https://www.prnewswire.com/apac/news-releases/innovent-and-sanegenebio-announce-first-participant-dosed-in-a-phase-1-clinical-trial-of-ibi3016-agt-sirna-302213108.html, (accessed 1 August 2024, 2024).
- D. P. Judge, A. V. Kristen, M. Grogan, M. S. Maurer, R. H. Falk, M. Hanna, J. Gillmore, P. Garg, A. K. Vaishnaw, J. Harrop, C. Powell, V. Karsten, X. Zhang, M. T. Sweetser, J. Vest and P. N. Hawkins, Cardiovasc. Drugs Ther., 2020, 34, 357–370 CrossRef CAS.
- T. S. Zimmermann, V. Karsten, A. Chan, J. Chiesa, M. Boyce, B. R. Bettencourt, R. Hutabarat, S. Nochur, A. Vaishnaw and J. Gollob, Mol. Ther., 2017, 25, 71–78 CrossRef CAS PubMed.
- R. Marchi, L. Duarte, A. Ke, B. Hl and E. Al, Hematol. Transfus. Cell Ther., 2020, 42, 17–18 CrossRef.
- L. J. Scott, Drugs, 2020, 80, 335–339 CrossRef PubMed.
- Z. A. Massy and T. B. Drueke, Kidney Int., 2022, 101, 208–211 CrossRef CAS.
- K. Dyrbuś, M. Gąsior, P. Penson, K. K. Ray and M. Banach, J. Clin. Lipidol., 2020, 14, 16–27 CrossRef.
- S. J. Keam, Drugs, 2022, 82, 1419–1425 CrossRef CAS.
- D. Adams, I. Tournev, M. Taylor, T. Coelho, V. Planté-Bordeneuve, J. Berk, A. González-Duarte, J. Gillmore, S.-C. Low, Y. Sekijima, L. Obici, C. Chen, P. Badri, S. Arum, J. Vest and M. Polydefkis, Neurology, 2022, 98, 2974 Search PubMed.
- R. Shilling, V. Karsten, N. Silliman, J. Chen, W. Li and J. Vest, J. Am. Coll. Cardiol., 2020, 75, 3579–3579 CrossRef.
- A. Liu, J. Zhao, M. Shah, J. M. Migliorati, S. M. Tawfik, R. Bahal, T. P. Rasmussen, J. E. Manautou and X. B. Zhong, ACS Pharmacol. Transl. Sci., 2022, 5, 1007–1016 CrossRef CAS PubMed.
- B. Hoppe, Nat. Rev. Nephrol., 2012, 8, 467–475 CrossRef CAS.
-
A. Armandi and J. M. Schattenberg, in From Obesity to Diabetes, ed. J. Eckel and K. Clément, Springer International Publishing, Cham, 2022, pp. 253–267, DOI:10.1007/164_2021_561.
- Y. Wang, R. Z. Yu, S. Henry and R. S. Geary, Expert Opin. Drug Metab. Toxicol., 2019, 15, 475–485 CrossRef CAS.
- S. T. Crooke, B. F. Baker, S. Xia, R. Z. Yu, N. J. Viney, Y. Wang, S. Tsimikas and R. S. Geary, Nucleic Acid Ther., 2019, 29, 16–32 CrossRef CAS.
- M. Maurer, A. Kristen, M. Benson, R. Falk, G. Buchele, M. Brambatti, S. Tsimikas, N. Viney, L. Tai, C. Monteiro, Q. Yang, L. O'Dea, E. Schneider, R. Geary and B. Monia, Can. J. Cardiol., 2021, 37, S69 CrossRef.
- E. J. Ackermann, S. Guo, S. Booten, L. Alvarado, M. Benson, S. Hughes and B. P. Monia, Amyloid, 2012, 19, 43–44 CrossRef CAS.
- N. J. Viney, S. Guo, L.-J. Tai, B. F. Baker, M. Aghajan, S. W. Jung, R. Z. Yu, S. Booten, H. Murray, T. Machemer, S. Burel, S. Murray, G. Buchele, S. Tsimikas, E. Schneider, R. S. Geary, M. D. Benson and B. P. Monia, ESC Heart Fail., 2021, 8, 652–661 CrossRef PubMed.
- J. K. Diep, R. Z. Yu, N. J. Viney, E. Schneider, S. Guo, S. Henry, B. Monia, R. Geary and Y. Wang, Br. J. Clin. Pharmacol., 2022, 88, 5389–5398 CrossRef CAS PubMed.
- L. C. A. Stiekema, K. H. M. Prange, R. M. Hoogeveen, S. L. Verweij, J. Kroon, J. G. Schnitzler, K. E. Dzobo, A. J. Cupido, S. Tsimikas, E. S. G. Stroes, M. P. J. de Winther and M. Bahjat, Eur. Heart J., 2020, 41, 2262–2271 CrossRef CAS PubMed.
- S. Tsimikas, E. Karwatowska-Prokopczuk, I. Gouni-Berthold, J.-C. Tardif, S. J. Baum, E. Steinhagen-Thiessen, M. D. Shapiro, E. S. Stroes, P. M. Moriarty, B. G. Nordestgaard, S. Xia, J. Guerriero, N. J. Viney, L. O'Dea and J. L. Witztum, N. Engl. J. Med., 2020, 382, 244–255 CrossRef CAS.
- L. M. Fijen, M. A. Riedl, L. Bordone, J. A. Bernstein, J. Raasch, R. Tachdjian, T. Craig, W. R. Lumry, M. E. Manning, V. J. Alexander, K. B. Newman, A. Revenko, B. F. Baker, C. Nanavati, A. R. MacLeod, E. Schneider and D. M. Cohn, N. Engl. J. Med., 2022, 386, 1026–1033 CrossRef CAS.
- G. Bhattacharjee, A. S. Revenko, J. R. Crosby, C. May, D. Gao, C. Zhao, B. P. Monia and A. R. MacLeod, Nucleic Acid Ther., 2013, 23, 175–187 CrossRef CAS PubMed.
- M. F. Yuen, J. Heo, H. Kumada, F. Suzuki, Y. Suzuki, Q. Xie, J. Jia, Y. Karino, J. Hou, K. Chayama, M. Imamura, J. Y. Lao-Tan, S. G. Lim, Y. Tanaka, W. Xie, J. H. Yoon, Z. Duan, M. Kurosaki, S. J. Park, M. E. Labio, R. Kumar, Y. O. Kweon, H. J. Yim, Y. Tao, J. Cremer, R. Elston, M. Davies, S. Baptiste-Brown, K. Han, F. M. Campbell, M. Paff and D. Theodore, J. Hepatol., 2022, 77, 967–977 CrossRef CAS PubMed.
- H. R. Büller, C. Bethune, S. Bhanot, D. Gailani, B. P. Monia, G. E. Raskob, A. Segers, P. Verhamme and J. I. Weitz, N. Engl. J. Med., 2015, 372, 232–240 CrossRef.
- M. McCaleb, J. Lickliter, A. Dibble, E. Schneider, M. Aghajan, S. Guo, S. Hughes, R. S. Geary and B. P. Monia, Blood, 2018, 132, 3634 CrossRef.
- G. J. Jaffe, J. Sahni, S. Fauser, R. S. Geary, E. Schneider and M. McCaleb, Invest. Ophthalmol. Visual Sci., 2020, 61, 4305 Search PubMed.
- R. Loomba, E. Morgan, L. Watts, S. Xia, L. A. Hannan, R. S. Geary, B. F. Baker and S. Bhanot, Lancet Gastroenterol. Hepatol., 2020, 5, 829–838 CrossRef.
- T. Nie, Drugs, 2024, 84, 473–478 CrossRef CAS.
- C. Yeang, E. Karwatowska-Prokopczuk, F. Su, B. Dinh, S. Xia, J. L. Witztum and S. Tsimikas, J. Am. Coll. Cardiol., 2022, 79, 1035–1046 CrossRef CAS PubMed.
- J. Hardy, S. Niman, R. F. Goldfaden, M. Ashchi, M. Bisharat, J. Huston, H. Hartmann and R. Choksi, Am. J. Cardiovasc. Drugs, 2022, 22, 47–54 CrossRef CAS.
- B. A. Warden and P. B. Duell, Drugs Future, 2022, 47, 11–25 CrossRef.
- S. Tsimikas, E. Karwatowska-Prokopczuk, I. Gouni-Berthold, J. Tardif, S. Baum, E. Steinhagen-Thiessen, M. Shapiro, E. Stroes, P. Moriarty and B. Nordestgaard, N. Engl. J. Med., 2020, 382, 244–255 CrossRef CAS PubMed.
- R. Fernandez-Prado, M. V. Perez-Gomez and A. Ortiz, Clin. Kidney J., 2020, 13, 753–757 CrossRef CAS.
- J.-C. Tardif, E. Karwatowska-Prokopczuk, E. S. Amour, C. M. Ballantyne, M. D. Shapiro, P. M. Moriarty, S. J. Baum, E. Hurh, V. J. Bartlett, J. Kingsbury, A. L. Figueroa, V. J. Alexander, J. Tami, J. L. Witztum, R. S. Geary, L. S. L. O'Dea, S. Tsimikas and D. Gaudet, Eur. Heart J., 2022, 43, 1401–1412 CrossRef CAS PubMed.
- H. Javanbakht, H. Mueller, J. Walther, X. Zhou, A. Lopez, T. Pattupara, J. Blaising, L. Pedersen, N. Albæk, M. Jackerott, T. Shi, C. Ploix, W. Driessen, R. Persson, J. Ravn, J. A. T. Young and S. Ottosen, Mol. Ther.--Nucleic Acids, 2018, 11, 441–454 CrossRef CAS.
- N. Brodyagin, M. Katkevics, V. Kotikam, C. A. Ryan and E. Rozners, Beilstein J. Org. Chem., 2021, 17, 1641–1688 CrossRef CAS PubMed.
- S. M. van Rossenberg, K. M. Sliedregt-Bol, P. Prince, T. J. van Berkel, J. H. van Boom, G. A. van der Marel and E. A. Biessen, Bioconjugate Chem., 2003, 14, 1077–1082 CrossRef CAS PubMed.
- T. Ishihara, A. Kano, K. Obara, M. Saito, X. Chen, T. G. Park, T. Akaike and A. Maruyama, J. Controlled Release, 2011, 155, 34–39 CrossRef CAS.
- X. Zhang, C. G. Simmons and D. R. Corey, Bioorg. Med. Chem. Lett., 2001, 11, 1269–1272 CrossRef CAS.
- E. A. Biessen, K. Sliedregt-Bol, P. A. C. 't Hoen, P. Prince, E. Van der Bilt, A. R. Valentijn, N. J. Meeuwenoord, H. Princen, M. K. Bijsterbosch, G. A. Van der Marel, J. H. Van Boom and T. J. Van Berkel, Bioconjugate Chem., 2002, 13, 295–302 CrossRef CAS PubMed.
- P. Bhingardeve, B. R. Madhanagopal, H. Naick, P. Jain, M. Manoharan and K. Ganesh, J. Org. Chem., 2020, 85, 8812–8824 CrossRef CAS PubMed.
- A. D. Keefe, S. Pai and A. Ellington, Nat. Rev. Drug Discovery, 2010, 9, 537–550 CrossRef CAS PubMed.
- T. N. Zamay, O. S. Kolovskaya, Y. E. Glazyrin, G. S. Zamay, S. A. Kuznetsova, E. A. Spivak, M. Wehbe, A. G. Savitskaya, O. A. Zubkova, A. Kadkina, X. Wang, D. Muharemagic, A. Dubynina, Y. Sheina, A. B. Salmina, M. V. Berezovski and A. S. Zamay, Nucleic Acid Ther., 2014, 24, 160–170 CrossRef CAS PubMed.
- Y. Wu, B. Lin, Y. Lu, L. Li, K. Deng, S. Zhang, H. Zhang, C. Yang and Z. Zhu, Angew. Chem., Int. Ed., 2023, 62, e202218106 CrossRef CAS.
- J. A. Doudna and E. Charpentier, Science, 2014, 346, 1258096 CrossRef.
- R. Rouet, B. A. Thuma, M. D. Roy, N. G. Lintner, D. M. Rubitski, J. E. Finley, H. M. Wisniewska, R. Mendonsa, A. Hirsh, L. de Oñate, J. Compte Barrón, T. J. McLellan, J. Bellenger, X. Feng, A. Varghese, B. A. Chrunyk, K. Borzilleri, K. D. Hesp, K. Zhou, N. Ma, M. Tu, R. Dullea, K. F. McClure, R. C. Wilson, S. Liras, V. Mascitti and J. A. Doudna, J. Am. Chem. Soc., 2018, 140, 6596–6603 CrossRef CAS.
-
S. Liras, V. Mascitti, B. A. Thuma, J. A. Doudna and R. Rouet, WO Pat., WO2017083368A9, 2016 Search PubMed.
- J. M. Tsai, R. P. Nowak, B. L. Ebert and E. S. Fischer, Nat. Rev. Mol. Cell Biol., 2024, 25, 740–757 CrossRef CAS PubMed.
- L. Zhao, J. Zhao, K. Zhong, A. Tong and D. Jia, Signal Transduction Targeted Ther., 2022, 7, 113 CrossRef CAS.
- S. M. Banik, K. Pedram, S. Wisnovsky, G. Ahn, N. M. Riley and C. R. Bertozzi, Nature, 2020, 584, 291–297 CrossRef CAS.
- G. Ahn, S. M. Banik, C. L. Miller, N. M. Riley, J. R. Cochran and C. R. Bertozzi, Nat. Chem. Biol., 2021, 17, 937–946 CrossRef CAS.
- D. F. Caianiello, M. Zhang, J. D. Ray, R. A. Howell, J. C. Swartzel, E. M. J. Branham, E. Chirkin, V. R. Sabbasani, A. Z. Gong, D. M. McDonald, V. Muthusamy and D. A. Spiegel, Nat. Chem. Biol., 2021, 17, 947–953 CrossRef CAS.
- Y. Zhou, P. Teng, N. T. Montgomery, X. Li and W. Tang, ACS Cent. Sci., 2021, 7, 499–506 CrossRef CAS.
-
D. Spiegel, D. Caianiello and M. Zhang, WO. Pat., WO2019199634A4, 2019 Search PubMed.
- G. Ahn, S. M. Banik, C. L. Miller, N. M. Riley, J. R. Cochran and C. R. Bertozzi, Nat. Chem. Biol., 2021, 17, 937–946 CrossRef CAS PubMed.
- T. C. Donahue, C. Ou, Q. Yang, R. Flinko, X. Zhang, G. Zong, G. K. Lewis and L. X. Wang, ACS Chem. Biol., 2023, 18, 1611–1623 CrossRef CAS PubMed.
- K. Wang, A. Yu, K. Liu, C. Feng, Y. Hou, J. Chen, S. Ma, L. Huang and X. Dai, Adv. Sci., 2023, 10, e2300288 CrossRef.
-
S. Liras, V. Mascitti, B. A. Thuma and R. Rouet, WO. Pat., WO2021155317A1, 2021 Search PubMed.
-
M. G. Saulnier, J. J. Chen, S. Karra, K. T. Sprott, J. A. Wiles and S. Ray, WO. Pat., WO2021155317, 2021 Search PubMed.
-
E. Tozzo, Presented in part at the 9th Drug Discovery Strategic Summit (DDSS), 2022 Search PubMed.
- M. Yu, J. Qin, X. Liu, D. Ramsden, B. Williams, I. Zlatev, D. Guenther, S. Matsuda, R. Tymon, J. Darcy, C. Wong, J. Tsung, P. Zawaneh, S. Chong, Ch. S. Theile, N. Taneja, A. Rogers, J. Liu, E. Castellanos-Rizaldos, S. Bond, K. So, J. Denoncourt, A. Castoreno, M. Manoharan, J.-T. Wu, K. Fitzgerald, M. A. Maier, V. Jadhav and J. K. Nair, Nucleic Acids Res., 2024, 52, 5423–5437 CrossRef CAS PubMed.
|
This journal is © The Royal Society of Chemistry 2025 |
Click here to see how this site uses Cookies. View our privacy policy here.