Assessing microplastics, per- and polyfluoroalkyl substances (PFAS), and other contaminants of global concern in wadable agricultural streams in Iowa†
Received
4th December 2024
, Accepted 21st March 2025
First published on 28th March 2025
Abstract
Microplastics, per- and polyfluoroalkyl substances (PFAS), antibiotic resistance genes (ARGs), pharmaceuticals and personal care products (PPCPs), and pesticides may lead to unintended environmental contamination through many pathways in multiple matrices. This statewide, multi-matrix study of contaminants of global concern (CGCs) in agricultural streams across Iowa (United States) is the first to examine multiple CGCs in water, bed sediment, and fish to understand their occurrence in small streams located in regions of intense agriculture activity. Iowa plays a pivotal role in agriculture, with more than 85% of Iowa's landscape devoted to agriculture, making it an ideal location for determining the prevalence of CGCs to provide critical baseline exposure data. Fifteen sites were sampled across a range of predominant land uses (e.g., poultry, swine); all sites had detections of microplastics in all matrices. Concentrations of PFAS varied but were detected in water and sediment; all fish had detections of perfluorooctanesulfonate (PFOS), a type of PFAS. More than 50% of water and bed sediment samples had detections of ARGs. The most frequently detected PPCP was metformin. No sites had a cumulative exposure activity ratio greater than 1.0 for chemical exposures; 13 sites were above the 0.001 precautionary threshold. Toxicity quotients calculated using Aquatic Life Benchmarks were below the 0.1 moderate risk threshold for chemical exposures for all but one site. For fish, all sites exceeded the moderate and high-risk thresholds proposed for microplastic particles for food dilution (both chronic and acute exposures) and all sites exceeded the microplastic moderate threshold proposed for chronic tissue translocation, and two sites exceeded the threshold for acute tissue translocation.
Environmental significance
This statewide, multi-matrix study of contaminants of global concern (CGCs) in agricultural streams across Iowa (USA) is the first to examine multiple CGCs in water, bed sediment, and fish to understand their occurrence in small streams located in regions of intense agriculture activity. The CGCs microplastics, per- and polyfluoroalkyl substances, antibiotic resistance genes, pharmaceuticals and personal care products, and pesticides cause unintentional environmental contamination through many pathways in multiple matrices. Iowa plays a pivotal role in agriculture, with more than 85% of Iowa's landscape devoted to agriculture, making it an ideal location for determining the prevalence of CGCs to provide critical baseline exposure data.
|
Introduction
Mounting evidence indicates that anthropogenic actions and products intended to improve the quality of life are causing unintentional environmental contamination on a global scale.1,2 The persistence, mobility, and bioaccumulation potential of these materials has translated to their presence in all physical and biological environmental compartments. Such contaminants of global concern (CGCs) include microplastics, per- and polyfluoroalkyl substances (PFAS), antibiotic resistance genes (ARGs), pharmaceuticals and personal care products (PPCPs), and pesticides. Previous research has documented that the simultaneous presence of a complex mixture of a wide range of contaminants often occurs.3,4 Contaminants can contribute synergistically to toxicity even if they are present below their own individual effect threshold.5 In addition, such contaminant mixtures can cause surface waters to be biologically active (e.g., estrogen, androgen, glucocorticoid activity).6,7
Plastics play an important role in many aspects of modern human life, and their transformation products are often problematic. As produced plastics become microplastics (less than [<] 5 mm in size)8via physical or mechanical weathering, they enter the “natural” environment, with a nebulous fate. Microplastics are ubiquitous in the environment, including in oceans,8 rivers,9 lakes,10 sediments,11,12 biota,13,14 humans,15,16 atmosphere,17,18 and even remote areas far-removed from anthropogenic sources.19–21 By 2050, it is projected that 12
000 million metric tons of total plastic waste will have accumulated in landfills or the environment.22 Although much microplastic research to date has been conducted in marine settings, growing evidence indicates lotic systems are instrumental in transporting microplastics,23,24 with particles prevalent in both stream water and bed sediment.25–27 Although the bulk of freshwater microplastic research is focused on urban activity, agricultural production also contributes microplastics to the environment28–30 through various management practices, including municipal biosolid applications to farmland.31,32
As with plastics, PFAS play a decades-long role in modern society with innumerable uses including firefighting foams, water and stain repellents, and PPCPs. Optimization for consumer and industrial applications also allows them to enter the environment and be transported through a variety of pathways33 and accumulate in plant, animal, and human tissues.34–38 PFAS are linked to a range of deleterious health effects including low birth weights, hormone interference, reduced immune response, and various cancers.39–42
Concern regarding antimicrobial resistance (AMR) has increased due to the extensive use of antimicrobials for both human and agricultural purposes. Approximately 80% of the antibiotics used in the United States are for food animal production.43 Antibiotic overuse has led to AMR now being recognized as a global threat to human health with the serious potential of a post-antibiotic era where antibiotics are rendered obsolete.44 It is projected that resistant bacterial infections could become the leading cause of global deaths by 2050.44 Antibiotic resistant bacteria (ARB) and associated antibiotic resistance genes (ARGs) are now recognized as environmental contaminants.45 Numerous mechanisms and pathways for environmental transfer of ARB and ARGs have been identified, with water considered an important transmission pathway.46,47 Recent investigations found that antibiotic resistant enterococci and staphylococci were common in Iowa streams, with stream bed sediment an important reservoir for ARGs.48
PPCPs are organic chemicals that have been developed to enhance the quality of human life; however, evidence indicates that environmental exposure to PPCPs and their associated transformation products such as guanylurea can have deleterious effects on ecosystems.49,50 PPCP consumption worldwide is rising due to an increasing global population with a rising median age.51 As the demand for PPCPs increases, corresponding discharge through wastewater treatment plants (WWTPs) and manufacturing facilities into the environment also increases.52–54 PPCPs have both urban and agricultural inputs to streams55–61 and have been found in streams globally on all seven continents.1
Pesticides are exceedingly common, particularly in agricultural production in the United States, for which more than 226 million kilograms of pesticides62 were land applied annually between 2013 and 2017.63 This intensive use and related exposure to nontarget organisms is of increasing global concern.64 Although pesticides have commonly been used in agriculture since the 1950s, conventional agriculture has increasingly adopted prophylactic technologies using pesticides at or before planting65 that is concomitant with a rise in the global use of pesticides.66 Mixtures of pesticides62 and pesticide transformation products67 are increasingly common in urban and agricultural streams across the United States, often with deleterious effects to aquatic and terrestrial organisms.68–72
Rural streams have a long history of research on contaminants traditionally associated with agriculture. These contaminants, often from land-applications, waste lagoons, and confined animal feeding operations, include nutrients, pesticides, and bacteria that move into streams and groundwater through a variety of mechanisms, such as overland flow.73–75 The ecosystem services of rural lotic environments are often overlooked, despite the critical value of the habitat.76 Much research exists on the presence of non-traditional contaminants such as pharmaceuticals in streams in agricultural areas.77 Conversely, there is a paucity of information on contaminant exposures in non-aqueous matrices and biota. To address the research gap, we conducted a statewide, multi-matrix study of CGCs in wadable, agricultural streams across Iowa (United States). Iowa plays a pivotal role in agricultural in the United States,78 with more than 85% of Iowa's landscape devoted to agriculture, leading the nation in corn, soybean, swine, and egg production,79 and consequently is ideal for determining baseline exposure data regarding the prevalence of CGCs in agricultural streams. To our knowledge, this is the first study to simultaneously examine multiple classes of CGCs in multiple compartments (e.g., water, sediment, and fish) to better understand their occurrence in small streams located in regions of intense agriculture activity.
Materials and methods
Site selection & sample collection
Fifteen stream sites were selected across Iowa based on basin characteristics, previous water quality data, and proximity to established U.S. Geological Survey (USGS) gaging stations (Table S1†). Of the 15 sites sampled, 6 were in basins dominated by a specific livestock type (2 each for cattle, poultry, and swine), 3 sites had mixed livestock (livestock polyculture), 3 sites had substantial municipal biosolids applied to farmland in the basin, 2 sites were affected by WWTPs discharging directly upstream from sampling points, and 1 site was considered to be a reference site (dominated by forested land) with minimal agriculture in the basin (Fig. 1). The streams sampled had a wide range of characteristics including developed land use80 (1.9–62%), cultivated land80 (14.4–93.3%), animal units81 (AU; 0–643 AU per square kilometer [km2]), human population82 (2–739 people per km2), and WWTPs83 (0–10).
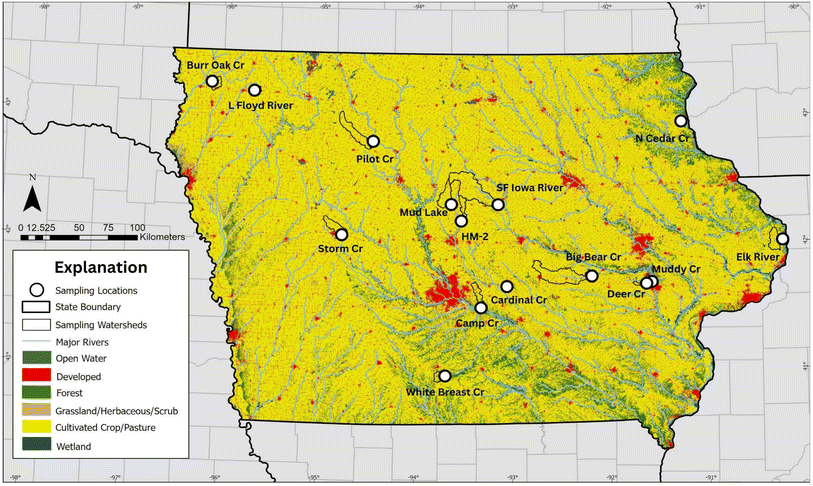 |
| Fig. 1 Site locations for 15 Iowa streams, 2021. | |
Water, bed sediment, and fish samples were collected April through June 2021 from sites during base-flow conditions. Water samples were always collected first, sediment was then collected from areas of deposition upstream from the water sample, and then netted microplastics samples were collected in the center of flow. Fish sample collection was opportunistic, and fish were collected using a seine net with a mesh size of 6.4 millimeters (mm)84 within the sampling reach (within 100 feet of discrete sampling). Fish sampling was not meant to be an ecological assessment; rather, samples were meant to be part of the overall snapshot of the chemical burden of the stream during the few hours the crew was on-site. Lastly, in situ field properties and discharge measurements were made. Water samples were collected and analyzed for microplastics, PFAS, bacteria concentrations and ARG presence, pesticides, PPCPs, total estrogenicity, nutrients, and suspended sediment. Bed sediment samples were collected and analyzed for microplastics, PFAS, and bacteria concentrations and ARGs. Fish, typically Cyprinidae minnows, were collected at 13 sites; 2 sites were netted but did not yield fish. All 13 sites had fish analyzed for PFAS while only 5 of the 13 were able to be analyzed for microplastics. Whole fish samples were collected and placed in jars and/or bags as required by individual laboratories and analyzed for microplastics, PFAS, three pharmaceuticals, and one pesticide. The number and size of fish varied by site, with some sites having as few as two minnows per jar; jars were immersed in ice in coolers immediately post-collection85 and all fish sampling was in accordance with the State of Iowa scientific collector permitting procedures. All samples were stored on ice at 4 °C in the field and shipped to arrive within 24 hours of collection to the various laboratories, as necessary.
Whole water samples were collected as single verticals/center-of-flow (VCF) samples.86 Filtered inorganic water samples were processed streamside from water collected in a high-density polyethylene (HDPE) bottle using a 60-cc disposable Luer lock syringe and an Aquaprep 0.45 µm disk filter. Filtered organic water samples were processed by collecting water in the center of flow using a 30 milliliter (mL) polypropylene Luer lock syringe and were filtered using a Whatman 0.7 µm, 25 mm glass fiber syringe tip filter for analysis of 82 pesticides by the USGS. A 1 liter (L) amber glass bottle was used to collect water for pharmaceutical analysis, and a 500 mL amber glass bottle was used to collect water for pesticide analysis at the University of Iowa in Iowa City, Iowa.
Microplastic water samples were collected in duplicate in the center of flow. Microplastic samples were collected both as netted and whole water samples as a comparison between the two methods. Netted samples were collected using a Hydro-Bios 300 µm net with a 125 µm cod end. Nets were held in flow for 15 minutes or until the net had too much debris (whichever came first). Nets were rinsed with deionized water (DI) into the cod end, which was decanted into a quart-sized mason jar that had been triple rinsed with DI water, fired at 450 °C for 4 hours, and then wrapped in foil. Whole water samples were collected in the center of flow in a quart-sized mason jar, prepared as previously mentioned. Subsequent analysis documented that the net used for microplastic collection shed substantial amounts of microplastics when using a laboratory method with a lower-than-standard size detection limit (detailed in the Laboratory Methods section). Thus, only whole water samples were analyzed and are presented here. PFAS water samples were collected in three 2 mL microcentrifuge tubes and submitted to the USGS National Water Quality Laboratory (NWQL) in Denver, Colorado, for analysis.
In situ field properties (water temperature, specific conductance, pH, dissolved oxygen, and turbidity) were collected in the center of flow using a Yellow Springs Incorporated (YSI) EXO2 multiparameter sonde. For ungaged sites, discharge measurements were made immediately following sample collection using a SonTek FlowTracker Acoustic Doppler Velocimeter.
Bed sediment samples were collected with stainless-steel spoons from three to five areas of deposition within the sampling reach with only the top 3 centimeters (cm) of bed sediment being sampled. Microplastic and PFAS bed sediment samples were composited in one glass jar each; microbial samples were composited in sterile plastic specimen cups. All reuseable field equipment used for sampling (HDPE bottle and stainless-steel spoons) was cleaned by dilute phosphate-free detergent soak, tap water rinse, and DI rinse.
Laboratory methods
Water and bed sediment samples for microplastic analysis were stored at room temperature, and fish were stored in a freezer; all samples were delivered to the University of Illinois, Illinois Sustainable Technology Center in Urbana, Illinois, after all sampling was complete. Full microplastic analytical methods are published in Scott and Green;87 the method is a Modified NOAA Method88 wherein water samples are concentrated by wet sieving (5 µm stainless steel sieve), then undergo wet peroxide oxidation (Fenton reagent), density separation, filtration to 0.45 µm, and then examined with a Zeiss SteREO Discovery V20 microscope for sizing, as well as notation of particle type (round, fiber, or fragment) and color. To measure the area of the particles, the x and y axes were measured with the Zeiss microscope and analyzed with the Zeiss Zen Blue software package. The z-axis was assumed to be 1 µm, a conservative value; detailed sizing methods are available in Prada.89 Microplastic analysis of bed sediment samples was similar to water, but started with density separation, wet sieving, wet peroxide oxidation, density separation, filtration to 0.45 µm, and then microscope examination as with water samples. Whole fish sample processing started with wet peroxide oxidation, wet sieving, and then oxidized and sieved again until free of visual biological and organic matter. Samples were then sorted by density, filtered to 0.45 µm, and then examined by microscope as with water samples. This modified method yields a lower detection limit (20 µm × 20 µm) and larger densities (greater than [>] 1.7 grams per cubic centimeter), which allow for more polymer types than the standard analytical method (Table S2†).
Water samples were analyzed for 34 PFAS at the NWQL using liquid chromatography-tandem mass spectrometry (LC/MS-MS).90 Sediment and whole fish samples were analyzed for 28 PFAS using a modified version of EPA Method 533 by SGS Axys in British Columbia, Canada.91
Water and bed sediment samples were enumerated for heterotrophic bacteria counts (HPC), total coliforms, Escherichia coli (E. coli), and enterococci at the USGS Michigan Bacteriological Research Laboratory (MI-BaRL) in Lansing, Michigan, using the IDEXX Quanti-Tray/2000 System (Westbrook, Maine) with either HPC, Colilert, or Enterolert tests (IDEXX; Westbrook, Maine). Growth from IDEXX HPC incubations was aseptically extracted from the tray system and plated on Reasoner's 2A (R2A) agar and extracted DNA from subsequent growth used to determine the presence of ARGs (Tables S3 and S4†). Polymerase chain reaction (PCR) was used to detect the presence of 25 bacterial gene markers (BGMs), including a Bacteria 16S rRNA gene, 20 ARGs, 3 virulence genes (femA, invA, and spvC), and 1 integrase gene (int) as described in Givens.48
Water samples were analyzed for pesticides at the NWQL and UIowa, and water and whole fish samples were analyzed for PPCPs at UIowa. Water samples submitted to the NWQL were analyzed for 82 pesticides and transformation products using direct aqueous injection-LC/MS/MS-(DAI LC-MS/MS).92 Water samples submitted to UIowa were analyzed for 14 PPCPs and 6 pesticides. The samples were vacuum filtered (Whatman glass microfiber filters [GF/F], nominal 0.7 µm) and frozen at −20 °C until extraction. Water samples were processed with solid-phase extraction (SPE) to increase detection limits and then analyzed using an Agilent LC-MS/MS. Whole fish samples were analyzed for three PPCPs and one pesticide. The fish samples were solvent extracted using a modified method from LeFevre93 and analyzed with the same Agilent LC-MS/MS.
Water samples were prepped for total estrogenicity analysis at the USGS Functional and Molecular Bioassay Laboratory in Kearneysville, West Virginia, by the USGS Organic Geochemistry Research Laboratory in Lawrence, Kansas. Samples were extracted within 36 hours of arrival, 1 L samples were filtered with a 0.7 µm glass-fiber, solid phase extracted to give 100 µL of extract, and stored at −20 °C until analysis. Sample extracts were screened for total estrogenicity using the bioluminescent yeast estrogen screen (BLYES) as previously described by Ciparis94 and Sanseverino.95 In brief, 20 µL of sample extracts were added in triplicate to the wells of white, solid-bottom 96-well microtiter plates and evaporated at room temperature. Following evaporation, 200 µL of a 48 hour culture of strain BLYES adjusted to 0.4 (OD600) in Yeast Minimal Media (YMM leu-, ura-)96 was added to each well. A 12-point standard curve of 17β-estradiol (E2; Sigma-Aldrich Co.) was included on each plate, and plates were covered and incubated in the dark at 30 °C for 4 hours. Luminescence was quantified using a SpectraMax M4 microplate reader (Molecular Devices) in luminescence mode, and estrogen equivalents (E2Eq) of each sample were determined via interpolation to a 4-parameter curve using SoftMax Pro 7.1.0 (Molecular Devices). The detection limit for this assay was 0.244 ng L−1 estradiol equivalents (E2Eq) BLYES.7,97
Quality assurance/quality control
Two field blanks were processed on-site using Fisher Scientific organic-free blank water for organic constituents and sterile DI water for microbial samples. Multiple blanks were processed for microplastics: a DI machine source blank was processed in the USGS Iowa City Water Quality Laboratory, a DI blank from a glass jug used to transport DI water and used in the field was processed in the Iowa City laboratory; a field blank was processed using DI water at a sampling site; water and sediment travel blanks provided by the University of Illinois Prairie Research Institute were analyzed. As necessary, blank water was run through the same equipment used to collect the environmental samples. All blank samples had low-level detections of microplastics (DI instrument: 11 counts per liter (CPL); DI blank: 3 CPL; field equipment blank: 10 CPL; travel blank, water: 9 CPL; travel blank, sediment: 3 counts per gram [CPG]). All other blanks were free of the target constituents. In addition to field quality control samples, three PFAS samples were randomly chosen and run as duplicates; there were no PFAS detections in these samples. Microbial laboratory blanks consisting of sterile DI water were processed along with the workflow of IDEXX enumeration, DNA extraction, and PCR. Aside from blanks, microplastic positive control spikes were run on three DI spike samples analyzed with each matrix batch with the recovery percentages ranging from 88–119.
Statistical analysis and risk assessment
All data analysis was done using RStudio statistical software, version 4.4.0.98 Initially, data were evaluated using Spearman's rank correlation coefficient (rho) to evaluate relations between laboratory results and basin characteristics including land use, basin size, and animal units within the watershed. This nonparametric statistical test was used because the analytical results contained left-censored data (i.e., non-detections). Results were considered significant when p < 0.05 and rho values were >0.50. The assumptions of normality and constant variance for the particle size of detected microplastics were evaluated by examining individual histograms for each site and sample median. Additionally, the Shapiro–Wilk test was applied to statistically assess normality, and the Fligner-Killeen test was used to evaluate homogeneity of variances.99 Because the normality and constant variance assumptions were not met, a one-factor permutation test, which does not rely on these assumptions, was used to assess differences in mean particle size across sites and primary land use types (based on land use in the basin) for each sample median. Results were considered significant when p < 0.05. A pairwise comparison of particle size by site and primary land use type between surface water and bed sediment samples was conducted using the Wilcoxon rank-sum test, with a Benjamini–Hochberg correction applied to control the family-wise error rate.100
Cumulative-exposure effects of potential human-health interest were screened using two bioactivity-weighted approaches: the cumulative Exposure-Activity Ratios (ΣEAR), and the cumulative toxicity quotient (ΣTQ). Both approaches are (1) constrained by the analytical scope of this study, which greatly underestimate the organic chemicals in commercial use and, by extension, in the environment;101 (2) limited to the available weighting-factors of ToxCast activity concentration at cutoff (ACC) and human-health benchmarks;102 and (3) assume cumulative effects are reasonably approximated by concentration addition.103–105 The ΣEAR were computed using chemical analytical results for pesticides, pharmaceuticals, and PFAS water samples by site using toxEval version 1.3.0
106 to determine potential biological effects of contaminants found in each sample. The ΣEARs were of potential concern when results were greater than or equal to (≥) 0.001. Lastly, toxicity quotients (TQ) were calculated using chemical results for pesticides as well as the physical results for microplastics for the stream water samples. Toxicity quotients were calculated in RStudio98 using Aquatic Life Benchmarks (ALB) established by the U.S. Environmental Protection Agency's Office of Pesticide Program to determine risk to freshwater fish from acute and chronic exposure to pesticides and their transformation products.107 Benchmarks do not currently exist for microplastics data, so thresholds proposed in Mehinto108 were adjusted for the purposes of this study. Mehinto108 proposed four thresholds for two fish health outcomes (food dilution and tissue translocation); this study used thresholds 1 and 2 as the benchmark criteria for chronic health issues and thresholds 3 and 4 for acute health issues for each health outcome. For food dilution, this study followed Mehinto108 using a benchmark of 0.3 microplastic particles per liter (PPL) for chronic health issues and 5 microplastic PPL for acute health issues with a particle size threshold of less than 25
000
000 square micrometers (µm2), to be referred to as 0.25 centimeters squared (cm2) in this study. Still following Mehinto,108 this study used a tissue translocation benchmark of 60 microplastic PPL for chronic health issues and 890 PPL for acute health issues with a particle size threshold of 83 µm, which is <6,889 µm2 when converting to area. Toxicity quotient results of ≥1 were considered significant. For the purposes of this study and these data, all estimated data values were included in all analyses.
Results and discussion
Contaminants ubiquitously present in stream water
Complex mixtures of chemical and microbial contaminants were present in the rural agricultural streams sampled in this study, which indicate unexpected anthropogenic sources. Microplastics, PFAS, ARGs, PPCPs, and pesticides were found in all streams sampled with 86 of 164 (about 52%) target contaminants being detected in water from at least one stream.109 Additionally, 28 target contaminants were detected in at least 50% of water samples, and 8 contaminants were detected in water from every stream sampled. Contaminants detected in every stream were E. coli, enterococci, heterotrophic bacteria, total coliforms, microplastics, atrazine, 2-hydroxyatrazine (OIET), and metolachlor sulfonic acid (SA). The number of contaminants in individual stream water samples ranged from 17 to 54 (Tables 1, S5 and S6†).109 Although the presence of pesticides (including pesticide transformation products), nitrate, and bacteria and ARGs was expected based on known agricultural inputs48,67,73,75 and previous research indicates that transport of PFAS and ARGs to streams does occur,48,90 such ubiquity for microplastics, PFAS, ARGs, and PPCPs in these small agricultural streams was unexpected (Fig. 2).
Table 1 Number of contaminant detections, percent detections, and minimum, median, and maximum concentrations by sample medium for 15 Iowa streams, 2021. Water sample concentration units are nanograms per liter (ng L−1); bed sediment and fish sample concentrations are in nanograms per kilogram (ng kg−1)a
Contaminant |
Type |
Water samples |
Bed sediment samples |
Fish samples |
Detect |
Percent detection |
Minimum |
Median |
Maximum |
Detect |
Percent detection |
Minimum |
Median |
Maximum |
Detect |
Percent detection |
Minimum |
Median |
Maximum |
ARG, antibiotic resistance genes; PFAS, per- and polyfluoroalkyl substances; PPCP, pharmaceutical and personal care products; nd, not detected; —, not applicable; UI, University of Iowa.
|
bla
ACC
|
ARG |
9/15 |
60 |
— |
— |
— |
7/14 |
50 |
— |
— |
— |
— |
— |
— |
— |
— |
bla
FOX
|
ARG |
9/15 |
60 |
— |
— |
— |
12/14 |
86 |
— |
— |
— |
— |
— |
— |
— |
— |
bla
CIT
|
ARG |
5/15 |
33 |
— |
— |
— |
6/14 |
43 |
— |
— |
— |
— |
— |
— |
— |
— |
bla
CTX-M
|
ARG |
4/15 |
27 |
— |
— |
— |
7/14 |
50 |
— |
— |
— |
— |
— |
— |
— |
— |
bla
DHA
|
ARG |
4/15 |
27 |
— |
— |
— |
6/14 |
43 |
— |
— |
— |
— |
— |
— |
— |
— |
bla
EBC
|
ARG |
4/15 |
27 |
— |
— |
— |
5/14 |
36 |
— |
— |
— |
— |
— |
— |
— |
— |
flo
ST
|
ARG |
4/15 |
27 |
— |
— |
— |
9/14 |
64 |
— |
— |
— |
— |
— |
— |
— |
— |
inv A |
ARG |
4/15 |
27 |
— |
— |
— |
2/14 |
14 |
— |
— |
— |
— |
— |
— |
— |
— |
van A |
ARG |
4/15 |
27 |
— |
— |
— |
6/14 |
43 |
— |
— |
— |
— |
— |
— |
— |
— |
bla
MOX
|
ARG |
3/15 |
20 |
— |
— |
— |
3/14 |
21 |
— |
— |
— |
— |
— |
— |
— |
— |
qnr A |
ARG |
3/15 |
20 |
— |
— |
— |
1/14 |
7 |
— |
— |
— |
— |
— |
— |
— |
— |
qnr B |
ARG |
3/15 |
20 |
— |
— |
— |
10/14 |
71 |
— |
— |
— |
— |
— |
— |
— |
— |
qnr S |
ARG |
2/15 |
13 |
— |
— |
— |
3/14 |
21 |
— |
— |
— |
— |
— |
— |
— |
— |
spv C |
ARG |
2/15 |
13 |
— |
— |
— |
5/14 |
36 |
— |
— |
— |
— |
— |
— |
— |
— |
tet M |
ARG |
2/15 |
13 |
— |
— |
— |
4/14 |
29 |
— |
— |
— |
— |
— |
— |
— |
— |
int |
ARG |
13/15 |
87 |
— |
— |
— |
12/14 |
86 |
— |
— |
— |
— |
— |
— |
— |
— |
bla
CMY-2
|
ARG |
1/15 |
7 |
— |
— |
— |
9/14 |
64 |
— |
— |
— |
— |
— |
— |
— |
— |
erm B |
ARG |
1/15 |
7 |
— |
— |
— |
1/14 |
7 |
— |
— |
— |
— |
— |
— |
— |
— |
van B |
ARG |
1/15 |
7 |
— |
— |
— |
0/15 |
0 |
nd |
nd |
nd |
— |
— |
— |
— |
— |
bla
CTX-M-32
|
ARG |
0/15 |
0 |
— |
— |
— |
3/14 |
21 |
— |
— |
— |
— |
— |
— |
— |
— |
bla
TEM
|
ARG |
0/15 |
0 |
— |
— |
— |
3/14 |
21 |
— |
— |
— |
— |
— |
— |
— |
— |
fem A |
ARG |
0/15 |
0 |
— |
— |
— |
1/14 |
7 |
— |
— |
— |
— |
— |
— |
— |
— |
BLYES |
Estrogenicity |
5/15 |
33 |
0.34 |
0.72 |
2.34 |
— |
— |
— |
— |
— |
— |
— |
— |
— |
— |
Escherichia coli
|
Microbial |
15/15 |
100 |
260 |
2450 |
18 270 |
12/14 |
86 |
10.0 |
103 |
512 |
— |
— |
— |
— |
— |
Enterococci |
Microbial |
15/15 |
100 |
50.0 |
2600 |
11 235 |
— |
— |
— |
— |
— |
— |
— |
— |
— |
— |
Heterotrophic bacteria |
Microbial |
15/15 |
100 |
9090 |
77 100 |
>2 419 600 |
14/14 |
100 |
5475 |
>241 960 |
>241 960 |
— |
— |
— |
— |
— |
Total coliforms |
Microbial |
15/15 |
100 |
4430 |
77 100 |
120 980 |
14/14 |
100 |
10.0 |
1821 |
129 970 |
— |
— |
— |
— |
— |
Microplastics |
Microplastics |
15/15 |
100 |
112 |
220 |
17 033 |
15/15 |
100 |
3782 |
43 668 |
109 435 |
5/5 |
100 |
199 |
301 |
1168 |
Atrazine (UI) |
Pesticide |
13/15 |
87 |
7.87 |
41.82 |
321.64 |
— |
— |
— |
— |
— |
4/13 |
31 |
0.10 |
1.20 |
1.20 |
2,4-D |
Pesticide |
9/15 |
60 |
39.33 |
78.27 |
305.90 |
— |
— |
— |
— |
— |
— |
— |
— |
— |
— |
Sulfentrazone |
Pesticide |
9/15 |
60 |
19.66 |
50.97 |
185.13 |
— |
— |
— |
— |
— |
— |
— |
— |
— |
— |
OIAT |
Pesticide |
8/15 |
53 |
1.09 |
8.70 |
15.61 |
— |
— |
— |
— |
— |
— |
— |
— |
— |
— |
Azoxystrobin |
Pesticide |
7/15 |
47 |
1.20 |
2.17 |
6.17 |
— |
— |
— |
— |
— |
— |
— |
— |
— |
— |
Bentazon |
Pesticide |
6/15 |
40 |
4.87 |
6.41 |
20.65 |
— |
— |
— |
— |
— |
— |
— |
— |
— |
— |
CEAT |
Pesticide |
6/15 |
40 |
10.51 |
27.56 |
74.74 |
— |
— |
— |
— |
— |
— |
— |
— |
— |
— |
Dimethenamid |
Pesticide |
6/15 |
40 |
1.54 |
18.12 |
187.32 |
— |
— |
— |
— |
— |
— |
— |
— |
— |
— |
Prometon |
Pesticide |
6/15 |
40 |
1.81 |
2.99 |
17.61 |
— |
— |
— |
— |
— |
— |
— |
— |
— |
— |
Acetochlor SA |
Pesticide |
5/15 |
33 |
124.7 |
170.67 |
1329.53 |
— |
— |
— |
— |
— |
— |
— |
— |
— |
— |
Metalaxyl |
Pesticide |
5/15 |
33 |
5.48 |
7.47 |
10.04 |
— |
— |
— |
— |
— |
— |
— |
— |
— |
— |
Propazine |
Pesticide |
5/15 |
33 |
0.97 |
4.75 |
13.63 |
— |
— |
— |
— |
— |
— |
— |
— |
— |
— |
Diketonitrile isoxaflutole |
Pesticide |
4/15 |
27 |
4.24 |
9.86 |
37.42 |
— |
— |
— |
— |
— |
— |
— |
— |
— |
— |
Propiconazole |
Pesticide |
4/15 |
27 |
2.67 |
5.30 |
52.55 |
— |
— |
— |
— |
— |
— |
— |
— |
— |
— |
Tebuconazole |
Pesticide |
4/15 |
27 |
4.55 |
7.96 |
11.51 |
— |
— |
— |
— |
— |
— |
— |
— |
— |
— |
Bromacil |
Pesticide |
3/15 |
20 |
5.09 |
8.72 |
20.28 |
— |
— |
— |
— |
— |
— |
— |
— |
— |
— |
Carbendazim |
Pesticide |
3/15 |
20 |
2.92 |
10.79 |
18.54 |
— |
— |
— |
— |
— |
— |
— |
— |
— |
— |
Imidacloprid |
Pesticide |
3/15 |
20 |
17.89 |
36.86 |
70.00 |
— |
— |
— |
— |
— |
— |
— |
— |
— |
— |
Metribuzin |
Pesticide |
3/15 |
20 |
7.95 |
20.63 |
20.13 |
— |
— |
— |
— |
— |
— |
— |
— |
— |
— |
Fipronil |
Pesticide |
2/15 |
13 |
8.10 |
— |
10.27 |
— |
— |
— |
— |
— |
— |
— |
— |
— |
— |
Phenylpyrazole |
Pesticide |
2/15 |
13 |
8.10 |
— |
10.28 |
— |
— |
— |
— |
— |
— |
— |
— |
— |
— |
Simazine |
Pesticide |
2/15 |
13 |
10.01 |
— |
17.61 |
— |
— |
— |
— |
— |
— |
— |
— |
— |
— |
Atrazine |
Pesticide |
15/15 |
100 |
9.43 |
67.77 |
1386.29 |
— |
— |
— |
— |
— |
— |
— |
— |
— |
— |
Metolachlor SA |
Pesticide |
15/15 |
100 |
197.09 |
595.26 |
1596.23 |
— |
— |
— |
— |
— |
— |
— |
— |
— |
— |
OIET |
Pesticide |
15/15 |
100 |
30.82 |
60.97 |
152.93 |
— |
— |
— |
— |
— |
— |
— |
— |
— |
— |
CAAT |
Pesticide |
14/15 |
93 |
26.12 |
100.45 |
316.37 |
— |
— |
— |
— |
— |
— |
— |
— |
— |
— |
CIAT |
Pesticide |
14/15 |
93 |
8.27 |
42.11 |
199.68 |
— |
— |
— |
— |
— |
— |
— |
— |
— |
— |
Metolachlor |
Pesticide |
14/15 |
93 |
3.78 |
77.91 |
612.07 |
— |
— |
— |
— |
— |
— |
— |
— |
— |
— |
Acetochlor |
Pesticide |
12/15 |
80 |
3.32 |
65.52 |
608.56 |
— |
— |
— |
— |
— |
— |
— |
— |
— |
— |
Dechlorometolachlor |
Pesticide |
12/15 |
80 |
2.27 |
7.34 |
41.09 |
— |
— |
— |
— |
— |
— |
— |
— |
— |
— |
Acetochlor OA |
Pesticide |
11/15 |
73 |
36.22 |
203.51 |
1251.32 |
— |
— |
— |
— |
— |
— |
— |
— |
— |
— |
Clothianidin |
Pesticide |
10/15 |
67 |
6.77 |
10.99 |
25.28 |
— |
— |
— |
— |
— |
— |
— |
— |
— |
— |
Hydroxymetolachlor |
Pesticide |
10/15 |
67 |
3.18 |
12.70 |
38.65 |
— |
— |
— |
— |
— |
— |
— |
— |
— |
— |
Imazethapyr |
Pesticide |
10/15 |
67 |
4.14 |
18.81 |
69.97 |
— |
— |
— |
— |
— |
— |
— |
— |
— |
— |
Metolachlor OA |
Pesticide |
10/15 |
67 |
113.28 |
266.09 |
688.61 |
— |
— |
— |
— |
— |
— |
— |
— |
— |
— |
Desulfinylfipronil |
Pesticide |
1/15 |
7 |
0.75 |
— |
— |
— |
— |
— |
— |
— |
— |
— |
— |
— |
— |
Dinotefuran |
Pesticide |
1/15 |
7 |
16.05 |
— |
— |
— |
— |
— |
— |
— |
— |
— |
— |
— |
— |
Fipronil amide |
Pesticide |
1/15 |
7 |
7.00 |
— |
— |
— |
— |
— |
— |
— |
— |
— |
— |
— |
— |
Sulfometuron methyl |
Pesticide |
1/15 |
7 |
3.48 |
— |
— |
— |
— |
— |
— |
— |
— |
— |
— |
— |
— |
Tebupirimfos |
Pesticide |
1/15 |
7 |
0.47 |
— |
— |
— |
— |
— |
— |
— |
— |
— |
— |
— |
— |
Tetraconazole |
Pesticide |
1/15 |
7 |
3.03 |
— |
— |
— |
— |
— |
— |
— |
— |
— |
— |
— |
— |
Thiamethoxam |
Pesticide |
1/15 |
7 |
17.50 |
— |
— |
— |
— |
— |
— |
— |
— |
— |
— |
— |
— |
Triclopyr |
Pesticide |
1/15 |
7 |
49.19 |
— |
— |
— |
— |
— |
— |
— |
— |
— |
— |
— |
— |
PFPeA |
PFAS |
3/15 |
20 |
7.00 |
E15.0 |
36.8 |
0/15 |
0 |
nd |
nd |
nd |
0/13 |
0 |
nd |
nd |
nd |
PFHpA |
PFAS |
0/15 |
0 |
— |
— |
— |
0/15 |
0 |
nd |
nd |
nd |
0/13 |
0 |
nd |
nd |
nd |
PFBA |
PFAS |
6/15 |
40 |
6.70 |
12.9 |
E25.2 |
2/15 |
13 |
220 |
— |
380 |
0/13 |
0 |
nd |
nd |
nd |
HFPO DA |
PFAS |
0/15 |
0 |
— |
— |
— |
0/15 |
0 |
nd |
nd |
nd |
0/13 |
0 |
nd |
nd |
nd |
6:2-FTS |
PFAS |
0/15 |
0 |
— |
— |
— |
2/15 |
13 |
206 |
— |
2600 |
1/13 |
8 |
2430 |
— |
— |
PFBS |
PFAS |
1/15 |
7 |
9.10 |
— |
— |
1/15 |
7 |
40.5 |
— |
— |
1/13 |
8 |
164 |
— |
— |
N-EtFOSAA |
PFAS |
0/15 |
0 |
— |
— |
— |
3/15 |
20 |
46.6 |
114 |
314 |
1/13 |
8 |
102 |
— |
— |
PFOA |
PFAS |
2/15 |
13 |
2.00 |
— |
36.7 |
2/15 |
13 |
50.3 |
— |
50.3 |
2/13 |
15 |
119 |
— |
451 |
PFHxS |
PFAS |
0/15 |
0 |
— |
— |
— |
1/15 |
7 |
197 |
— |
— |
2/13 |
15 |
106 |
— |
227 |
N-MeFOSAA |
PFAS |
0/15 |
0 |
— |
— |
— |
2/15 |
13 |
58.0 |
— |
495 |
2/13 |
15 |
121 |
— |
145 |
PFOSA |
PFAS |
0/15 |
0 |
— |
— |
— |
1/15 |
7 |
65.2 |
— |
— |
3/13 |
23 |
149 |
313 |
355 |
PFDS |
PFAS |
0/15 |
0 |
— |
— |
— |
1/15 |
7 |
190 |
— |
— |
4/13 |
31 |
160 |
171.5 |
203 |
PFTrDA |
PFAS |
0/15 |
0 |
— |
— |
— |
1/15 |
7 |
766 |
— |
— |
4/13 |
31 |
111 |
168 |
265 |
PFTDeDA |
PFAS |
0/15 |
0 |
— |
— |
— |
1/15 |
7 |
834 |
— |
— |
5/13 |
38 |
101 |
168 |
292 |
PFHxA |
PFAS |
0/15 |
0 |
— |
— |
— |
5/15 |
33 |
47.3 |
71.40 |
102 |
6/13 |
46 |
220 |
371 |
1740 |
PFNA |
PFAS |
0/15 |
0 |
— |
— |
— |
1/15 |
7 |
61.4 |
— |
— |
6/13 |
46 |
113 |
189 |
431 |
PFDoDA |
PFAS |
0/15 |
0 |
— |
— |
— |
2/15 |
13 |
57.2 |
— |
585 |
6/13 |
46 |
103 |
229 |
803 |
PFDA |
PFAS |
0/15 |
0 |
— |
— |
— |
2/15 |
13 |
78.7 |
— |
186 |
8/13 |
61 |
110 |
533 |
9620 |
PFUnDA |
PFAS |
0/15 |
0 |
— |
— |
— |
3/15 |
20 |
46.9 |
81.8 |
330 |
10/13 |
77 |
103 |
241 |
620 |
PFOS |
PFAS |
0/15 |
0 |
— |
— |
— |
8/15 |
53 |
45.3 |
55.25 |
413 |
13/13 |
100 |
413 |
3040 |
8580 |
Citalopram |
PPCP |
0/15 |
0 |
— |
— |
— |
— |
— |
— |
— |
— |
1/13 |
8 |
1 |
— |
— |
Venlafaxine |
PPCP |
3/15 |
20 |
6.63 |
141.47 |
143.21 |
— |
— |
— |
— |
— |
2/13 |
15 |
0.80 |
— |
2.8 |
Bupropion |
PPCP |
2/15 |
13 |
3.49 |
— |
17.71 |
— |
— |
— |
— |
— |
2/13 |
15 |
1.1 |
— |
5.7 |
Sucralose |
PPCP |
8/15 |
53 |
133.68 |
271.28 |
782.42 |
— |
— |
— |
— |
— |
— |
— |
— |
— |
— |
1H-Benzotriazole |
PPCP |
6/15 |
40 |
1.69 |
33.74 |
233.62 |
— |
— |
— |
— |
— |
— |
— |
— |
— |
— |
4/5-Methyl-1H-benzotriazole |
PPCP |
6/15 |
40 |
4.88 |
37.17 |
230.72 |
— |
— |
— |
— |
— |
— |
— |
— |
— |
— |
Carbamazepine |
PPCP |
4/15 |
27 |
9.22 |
24.47 |
90.98 |
— |
— |
— |
— |
— |
— |
— |
— |
— |
— |
Desvenlafaxine |
PPCP |
4/15 |
27 |
13.66 |
217.40 |
594.47 |
— |
— |
— |
— |
— |
— |
— |
— |
— |
— |
Guanylurea |
PPCP |
4/15 |
27 |
40.33 |
1046.72 |
2038.24 |
— |
— |
— |
— |
— |
— |
— |
— |
— |
— |
Sulfamethoxazole |
PPCP |
4/15 |
27 |
10.26 |
30.17 |
90.85 |
— |
— |
— |
— |
— |
— |
— |
— |
— |
— |
Fexofenadine |
PPCP |
3/15 |
20 |
86.45 |
105.94 |
570.36 |
— |
— |
— |
— |
— |
— |
— |
— |
— |
— |
Fluconazole |
PPCP |
3/15 |
20 |
10.08 |
15.44 |
18.73 |
— |
— |
— |
— |
— |
— |
— |
— |
— |
— |
Methocarbamol |
PPCP |
3/15 |
20 |
9.60 |
17.80 |
74.33 |
— |
— |
— |
— |
— |
— |
— |
— |
— |
— |
Lidocaine |
PPCP |
2/15 |
13 |
16.22 |
— |
85.68 |
— |
— |
— |
— |
— |
— |
— |
— |
— |
— |
Metformin |
PPCP |
13/15 |
87 |
113.33 |
822.50 |
8990 |
— |
— |
— |
— |
— |
— |
— |
— |
— |
— |
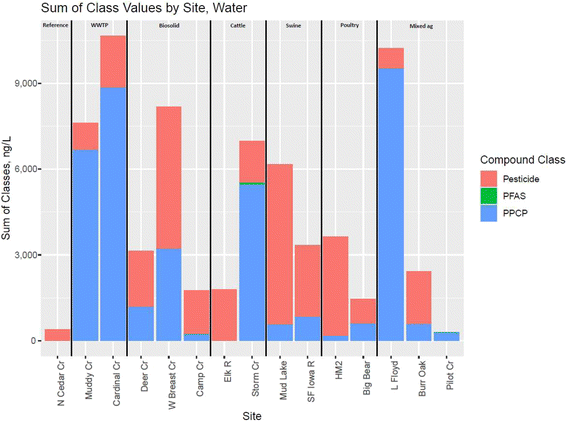 |
| Fig. 2 Sum of compound class values in nanograms per liter by site for 15 Iowa streams, 2021 [ng L−1, nanograms per liter; PFAS, per- and polyfluoroalkyl substances; PPCP, pharmaceuticals and personal care products; refer to Table S1† for full site names and information; Cr, Creek; R, River]. | |
The PPCPs metformin and sucralose have previously been associated with urban wastewater;61,110 thus, their presence in agriculturally dominated streams is particularly notable. For example, metformin, detected in 13 of 15 sites (Table S5†), had detections in the Little Floyd and Storm Creek at estimated values of 9,000 ng L−1 and 5500 ng L−1, respectively.109 In WWTPs, roughly 90% of metformin is microbially transformed to guanylurea;111 the Little Floyd has a single WWTP in its watershed, and Storm Creek has five. Neither site had noteworthy guanylurea detections (40 ng L−1 at Little Floyd Creek and no measurable concentrations at Storm Creek109). This indicates that metformin (and presumably detected PPCPs) is not likely derived from the WWTPs in the watershed, but instead may be from substandard septic systems in these areas.112,113 Consistent with this hypothesis, two sites that were sampled directly downstream from WWTPs had substantial concentrations of both metformin and guanylurea of 2435 ng L−1 and 2038 ng L−1, respectively (Muddy Creek), and 4820 ng L−1 and 2009 ng L−1, respectively (Cardinal Creek).109 Sucralose was detected at several sites (8 of 15), with detections ranging from about 134 ng L−1 to about 782 ng L−1. Although the presence of sucralose does not generally confirm the presence of untreated sewage (such as through a septic system or other point source) as with metformin, sucralose may be used as an indicator of human waste.114 These detections may point towards leaking or ineffective/substandard septic systems115 as sources of PPCPs detected at sites other than those directly associated with WWTPs in this study.
The presence of complex mixtures of contaminants in water may drive biological endpoints, including total estrogenicity. One-third of the stream water samples (5 of 15) for this study had measurable estrogenicity with 20% (3 water samples, one each from South Fork Iowa River, Muddy Creek, and Cardinal Creek) having estrogenicity within the range of potential environmental concern (exceeding 0.5 ng E2Eq/L).109 Estrogenicity in surface water may be influenced by various organic contaminants including but not limited to steroidal estrogens (e.g., estrone), phytoestrogens, and mycotoxins.6,116 These estrogenic compounds are known to be present in streams in this area through land application of animal manure and multiple mechanisms of transport such as overland flow and tile drainage, among others.117–119 Additionally, the presence of plastics in streams may influence estrogenicity, as phthalates are known to leach from plastics.120 Phthalates may also be present in other personal care products and are known to accumulate in the environment.121
The number of ARGs present in individual water samples (2–10) and bed sediment samples (2–13) (Table S6†) was highly variable by site. Storm Creek (n = 9) and Camp Creek (n = 10) had the highest number of ARGs detected in the water, whereas the highest number of ARGs detected in the bed sediment (n = 13) were from the Little Floyd River, Big Bear Creek, and Burr Oak Creek. As in Givens,48 a higher number of ARGs were detected in the bed sediment than the water, indicating that bed sediment may enhance the environmental persistence of antibiotics, ARB, and ARGs in the environment.48 The integrase (int) gene was detected in bacteria growth cultivated from either bed sediment or water from all samples except one site (HM-2). The prevalence of this ARG occurrence is notable as this gene indicates a potential for horizontal gene transfer between environmental bacteria and consequently the spread of environmental antibiotic resistance.122–124
The presence of PFAS in water samples was not unexpected and found in 9 of 15 sites, with concentrations ranging from 6.70 to 36.8 ng L−1 (Table 1).109 Additionally, PFAS were detected in 12 of 15 sediment samples (40.5–2600 nanograms per kilogram [ng kg−1]) and all fish samples collected at 13 sites (101–9620 ng kg−1). PFAS detections in water were found to be significantly positively correlated to detections of an insecticide, imidacloprid, specifically, as well cumulative insecticide detections (Table S7†). Both inert ingredients in pesticide formulations and leaching from containers can contribute to associations between pesticide and PFAS concentrations.125–127
Microplastics prevalent in all physical and biological compartments
Microplastics were detected at all sites in water and bed sediment samples and in fish samples from five sites (Table S8†). Statistically significant relations were observed between water clarity (field turbidity), suspended sediment mass, and suspended sediment concentration and microplastic counts in water samples (Table S7†). While counts and sizes of particles varied by site and medium, the bulk of the particles were smaller than 5000 µm2 (Table 2); the percentage of water sample microplastic particles <5000 µm2 ranged from about 49 to 91 by site (median 72; Table 2). Microplastics were generally smaller in sediment samples, with about 78 to 99.9% (median 98.6%) <5000 µm2 (Table 2). The water sample with the lowest number of particles <5000 µm2 was North Cedar Creek at about 49% (55 of 112 particles), but the associated sediment sample had about 97% of particles <5000 µm2 (51
788 of 53
437 particles; Table 2). For all five fish samples analyzed for microplastics, the percentages of particles <5000 µm2 was more comparable to the water samples with a range of about 60 to 95% (median 79.4; Table 2).
Table 2 Modified National Oceanic and Atmospheric Administration (NOAA) microplastics analysis method counts as compared to NOAA microplastics analysis method (90
000 µm2/0.3 mm threshold) by site and sample medium for 15 Iowa streams, 2021. Per size distribution charts, most particles were under 5000 µm2,a
Sites |
Water samples |
Bed sediment samples |
Fish samples |
90 000 µm cutoff |
5000 µm cutoff |
90 000 µm cutoff |
5000 µm cutoff |
90 000 µm cutoff |
5000 µm cutoff |
Count |
% |
Mass |
Count |
% <5000 µm |
Count |
% |
Count |
% <5000 µm |
Counts |
% |
Count |
% <5000 µm |
E, estimated; #, number; —, not applicable; %, percent; <less than; µm, micrometer.
|
Big Bear Creek at Ladora, Iowa |
E4124 |
— |
— |
E4124 |
— |
3/109 435 |
0.003 |
108 185/109 435 |
0.989 |
0/301 |
0.000 |
239/301 |
0.794 |
Burr Oak Creek near Perkins, Iowa |
1/159 |
0.629 |
0.118 |
85/159 |
0.535 |
0/18 633 |
0.000 |
18 610/18 633 |
0.999 |
— |
— |
— |
— |
Camp Creek near Runnells, Iowa |
E17033 |
— |
— |
E17 033 |
— |
3/27 951 |
0.011 |
27 558/27 951 |
0.986 |
— |
— |
— |
— |
Deer Creek near Coralville, Iowa |
0/246 |
0.000 |
0.000 |
148/246 |
0.602 |
119/35 589 |
0.334 |
30 686/35 589 |
0.862 |
0/1168 |
0.000 |
981/1168 |
0.840 |
Elk River near Almont, Iowa |
3/143 |
2.098 |
0.433 |
78/143 |
0.545 |
7/62 362 |
0.011 |
61 680/62 362 |
0.989 |
— |
— |
— |
— |
HM-2 |
1/396 |
0.253 |
0.114 |
347/396 |
0.876 |
2/43 668 |
0.005 |
43 279/43 668 |
0.991 |
— |
— |
— |
— |
Little Floyd River near Sanborn, Iowa |
5/577 |
0.867 |
0.672 |
508/577 |
0.880 |
93/51 216 |
0.182 |
39 860/51 216 |
0.778 |
— |
— |
— |
— |
Mud Lake near Jewell, Iowa |
0/358 |
0.000 |
0.000 |
304/358 |
0.849 |
1/3782 |
0.026 |
3746/3782 |
0.990 |
— |
— |
— |
— |
Muddy Creek near Coralville, Iowa |
2/201 |
0.995 |
0.205 |
162/201 |
0.806 |
9/9551 |
0.094 |
8758/9551 |
0.917 |
3/199 |
1.508 |
120/199 |
0.603 |
North Cedar Creek near Clayton, Iowa |
0/112 |
0.000 |
0.000 |
55/112 |
0.491 |
3/53 437 |
0.006 |
51 788/53 437 |
0.969 |
— |
— |
— |
— |
Pilot Creek at County Highway P15 near Rolfe, Iowa |
3/171 |
1.754 |
0.390 |
121/171 |
0.708 |
4/61 799 |
0.006 |
59 229/61 799 |
0.958 |
0/295 |
0.000 |
201/295 |
0.681 |
Cardinal Creek below Newton, Iowa |
0/207 |
0.000 |
0.000 |
149/207 |
0.720 |
6/88 664 |
0.007 |
87 389/88 664 |
0.986 |
— |
— |
— |
— |
South Fork Iowa River near New Providence, Iowa |
4/220 |
1.818 |
0.959 |
143/220 |
0.650 |
4/17 193 |
0.023 |
16 419/17 193 |
0.955 |
0/877 |
0.000 |
840/877 |
0.958 |
Storm Creek near Glidden, Iowa |
0/468 |
0.000 |
0.000 |
396/468 |
0.846 |
32/10 974 |
0.292 |
9632/10 974 |
0.878 |
— |
— |
— |
— |
South White Breast Creek near Osceola, Iowa |
1/592 |
0.169 |
0.091 |
540/592 |
0.912 |
2/43 668 |
0.005 |
43 279/43 668 |
0.991 |
— |
— |
— |
— |
Had the microplastic analysis been conducted using the standard NOAA method,88 the particle counts by sample would have been fundamentally different. For all sites and results across matrices, the standard NOAA method would have only detected 2% of particles compared to the modified methods. Similarly, the standard NOAA method would have only accounted for up to 0.4% of particle mass (only quantified for water samples). For example, the water sample for Deer Creek had a total count of 246 with particle sizes ranging from 433–63
700 µm2 (Table S7†).109 The size threshold for the standard NOAA method is 0.3 mm (90
000 µm2), which would have missed all 246 particles at this site. Likewise, the bed sediment sample from Deer Creek had particle sizes ranging from 41.9–556
000 µm2, and all but 119 of 35
589 particles would have been missed by the standard NOAA method (Table 2). Fish samples from the same site had all 1,168 particles below the standard NOAA method threshold. The standard NOAA method would have resulted in a gross underestimation of the influence of microplastics at Deer Creek (Fig. 3 and S9†).88 These numbers are similar for all sites (Table 2) and matrices (water 2%, sediment 0.33%, fish 1.5%). Notably, recent research has documented that microplastics below the standard NOAA threshold can have significant environmental effects.128 Using a microscope, all sample particles were evaluated for their shape and identified as either a fiber, fragment, or round. When evaluating by shape, most sites were dominated by plastic fragments, with fibers as secondary (ranging from 2–31%). The exception to this was the Little Floyd River, which had only fragments (64%) and particle rounds (35.7%) identified.109 For perspective, the highest percentage of rounds for other sites was 0.8% at South White Breast Creek and 0.4% at Storm Creek.
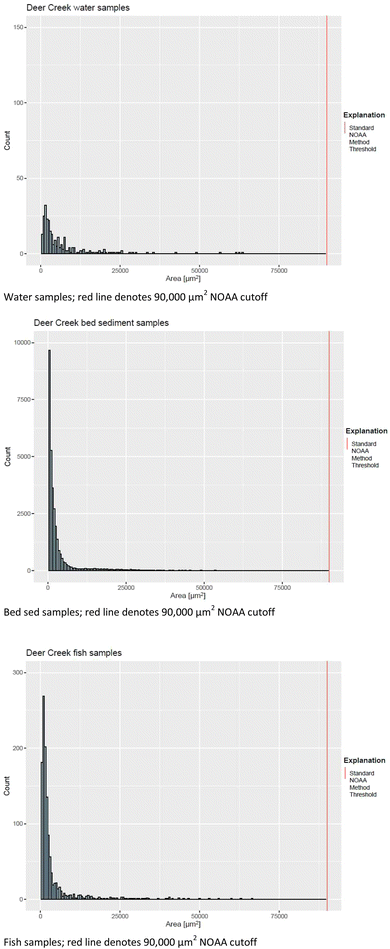 |
| Fig. 3 Size distribution of microplastic particles by medium at Deer Creek, Iowa, 2021 [µm2, square micrometers]. | |
All sites, in all sample mediums, had right-skewed distributions in the particle size of detected microplastics (Fig. S10, Table S11†). Results from Fligner-Killeen tests also found statistically significant differences in the variance across sites, with p < 0.05. Using a one-factor permutation test,99 statistically significant differences in the mean particle size were found in water samples for both site and primary land use type. Similar results were found for bed sediment samples by both site and primary land use type. The mean microplastic particle size in surface water samples was significantly larger than that of the bed sediment samples for all primary land use types and all but two sites, with p values <0.05. There were not statistically significant differences in water versus bed sediment samples at the Little Floyd River and Storm Creek. Further, the size of particles found in water samples from the Little Floyd River were smaller than all other samples (Fig. 4).
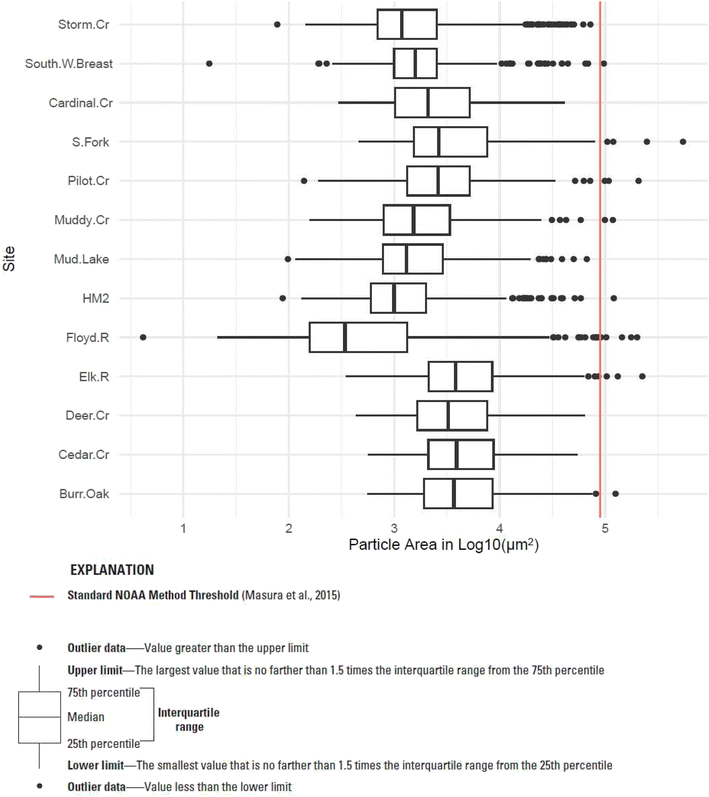 |
| Fig. 4 Log transformed areas of microplastic particles by site for water samples for 15 Iowa streams, 2021. Red line denotes NOAA method threshold (90 000 µm2). | |
Potential biological effects using exposure activity ratios (EAR)
As an assessment of the potential for biological effects from pesticide, pharmaceutical, and PFAS concentrations for water samples, EAR values were computed by site (Tables 3 and S12†). No sites exceeded the cumulative EAR (∑EAR) (sum of EAR for each contaminant) threshold of 1.0, a level at which biological effects may be expected. Thirteen sites did have ∑EAR of ≥0.001, a conservative threshold used to document samples of potential concern,129 with ∑EAR values ranging from 0.001–0.020. Pilot Creek had the highest ∑EAR value (0.020) and Elk River and North Cedar Creek had the lowest ∑EAR values (0.000003 and 0.00003, respectively). Individual EAR values were >0.001 for acetochlor (9 sites), metformin (3 sites), fipronil (2 sites each), and atrazine and metolachlor (1 site each) (Table S12†). Notably, of the 128 chemicals detected for this study, only 40 had available toxicity data necessary to calculate EAR. Thus, this assessment is likely conservative as it does not account for potential biological effects of chemicals without relevant toxicity data or for chemicals not in our suite of targeted analysis.
Table 3 Sum of exposure activity ratios (∑EAR) for water samples and toxicity quotients (∑TQ) for chronic and acute chemical exposures using the Aquatic Life Benchmarks (U.S. Environmental Protection Agency, 2023) by site (chemical exposures only) for 15 Iowa streams, 2021
Site |
∑EAR water |
∑TQ fish chronic |
∑TQ fish acute |
Big Bear Creek at Ladora, Iowa |
0.001 |
0.010 |
0.010 |
Burr Oak Creek near Perkins, Iowa |
0.002 |
0.022 |
0.010 |
Camp Creek near Runnells, Iowa |
0.001 |
0.015 |
0.022 |
Deer Creek near Coralville, Iowa |
0.004 |
0.018 |
0.015 |
Elk River near Almont, Iowa |
0.000* |
0.000 |
0.018 |
HM-2 |
0.003 |
0.029 |
0.000 |
Little Floyd River near Sanborn, Iowa |
0.005 |
0.007 |
0.029 |
Mud Lake near Jewell, Iowa |
0.006 |
0.054 |
0.007 |
Muddy Creek near Coralville, Iowa |
0.004 |
0.006 |
0.054 |
North Cedar Creek near Clayton, Iowa |
0.000* |
0.002 |
0.006 |
Pilot Creek at County Highway P15 near Rolfe, Iowa |
0.020 |
0.095 |
0.002 |
Cardinal Creek below Newton, Iowa |
0.007 |
0.053 |
0.095 |
South Fork Iowa River near New Providence, Iowa |
0.007 |
0.050 |
0.053 |
Storm Creek near Glidden, Iowa |
0.005 |
0.015 |
0.050 |
South White Breast Creek near Osceola, Iowa |
0.009 |
0.299 |
0.015 |
EAR |
≥0.001 potential concern |
1.0 high risk |
|
TQ |
0.1 moderate risk |
1.0 high risk |
|
|
* Rounded values |
|
|
Potential biological effects using toxicity quotients (TQs)
To assess potential cumulative-exposure effects from organic contaminants, ΣTQs were calculated using ALBs.107 A single site, White Breast Creek, exceeded the moderate risk TQ threshold of 0.1 for chronic and acute chemical exposures using the ALB analysis of pesticides and their transformation products in water samples. The highest ∑TQ using pesticide data was 0.299 at White Breast Creek, well below the established criteria (Tables 3 and S13†). When computing ∑TQs using microplastics data in water, however, the results are very different. Food dilution or false satiation may result from fish ingesting microplastic particles, which then may modify foraging efficiency. Insufficient nutrition including physical issues and depressed immune function can also result from the ingestion of non-food items.130 As the analysis of microplastic samples for this study allows for detection of very small particles, all particles were included in the analysis of TQs for food dilution (all particles under the method detection limit were considered estimated values; no particles were censored). All particle detections were under the threshold criteria for size and PPL for each site. As such, the TQs for both chronic exposure (37.3–5680, median 82) and acute exposure (3.29–501, median 7.24) are very high, all above the 1.0 threshold for high risk (Table 4). Tissue translocation is a scenario where particles can potentially cross organ boundaries, or create physical stress such as inflammation, which can lead to longer term health issues.131 When assessing tissue translocation, only the smallest fraction of particle sizes was included. In this instance, all particles larger than 6889 µm2 were censored out of the analysis. Two sites, Big Bear Creek and Camp Creek, had extremely high microplastics counts in water samples (4120 and about 17
000, respectively, Table 2), which did not allow for the computation of particle sizes. To compute TQs for these two sites, we made inferences on the percentage of particle sizes that would fit the size criteria to arrive at estimated (E) values. Of the 13 sites that did have particle size information, the percentage of particles in the smaller fraction ranged from 74 to 96% (Table 4). To remain conservative and allow for error, the lowest percentage (74%) was used to estimate the number of particles within the size criteria at Big Bear Creek and Camp Creek. All tissue translocation TQs for chronic exposure and two tissue translocation TQs for acute exposure exceed the high-risk threshold of 1.0. For chronic exposure, this range was 1.38–E210, median 3.1; for acute exposure, those values were 14.2 and 3.43. All other tissue translocation TQs for acute exposure exceed the moderate risk threshold of 0.1, with values ranging from 0.09–14.2, median 0.21 (Table 4).
Table 4 Toxicity quotients (TQs) for proposed microplastic benchmarks for chronic and acute exposures for two health outcomes. Results presented by site for 15 Iowa streams, 2021
Site |
Primary landuse |
Percentage less than 6889 µm2 |
Food dilution (FD) |
Tissue translocation (TT) |
FD MP countsc |
Chronic TQd |
Acute TQd |
TT MP countsb |
Chronic TQd |
Acute TQd |
Estimated based on lowest percentage of particles within threshold criteria for all sites (74%); benchmarks are in microplastics particles per liter (PPL); #E, estimated; µm2,micrometers squared.
Limited to microplastic particles <6889 µm2.
No size cutoff.
Italic = 0.1, moderate risk and bold = 1.0, high risk.
|
Big Bear Creek at Ladora, Iowa |
Poultry |
Unable to quantify |
4120 |
1370
|
121
|
E3050a |
E
210
|
14.2
|
Burr Oak Creek near Perkins, Iowa |
Mixed Ag |
75.4 |
159 |
53
|
4.68
|
120 |
2
|
0.135
|
Camp Creek near Runnells, Iowa |
Biosolid |
Unable to quantify |
17 000 |
5680
|
501
|
E12 600a |
E
50.9
|
3.43
|
Deer Creek near Coralville, Iowa |
Biosolid |
75.6 |
246 |
82
|
7.24
|
186 |
3.1
|
0.209
|
Elk River near Almont, Iowa |
Cattle |
77.6 |
143 |
47.7
|
4.21
|
111 |
1.85
|
0.125
|
HM-2 |
Poultry |
93.2 |
396 |
132
|
11.7
|
369 |
6.15
|
0.415
|
Little Floyd River near Sanborn, Iowa |
Mixed Ag |
92.7 |
577 |
192
|
17
|
535 |
8.92
|
0.601
|
Mud Lake near Jewell, Iowa |
Hog |
95.3 |
358 |
119
|
10.5
|
341 |
5.68
|
0.383
|
Muddy Creek near Coralville, Iowa |
WWTP |
92.0 |
201 |
46.3
|
4.09
|
185 |
3.08
|
0.208
|
North Cedar Creek near Clayton, Iowa |
Reference |
74.1 |
112 |
37.3
|
3.29
|
83 |
1.38
|
0.093 |
Pilot Creek at County Highway P15 near Rolfe, Iowa |
Mixed Ag |
87.7 |
171 |
57
|
5.03
|
150 |
2.5
|
0.169
|
Cardinal Creek below Newton, Iowa |
WWTP |
86.0 |
207 |
69
|
6.09
|
178 |
2.97
|
0.2
|
South Fork Iowa River near New Providence, Iowa |
Hog |
79.1 |
220 |
73.3
|
6.47
|
174 |
2.9
|
0.196
|
Storm Creek near Glidden, Iowa |
Cattle |
90.4 |
468 |
156
|
13.8
|
423 |
7.05
|
0.475
|
South White Breast Creek near Osceola, Iowa |
Biosolid |
96.1 |
592 |
197
|
17.4
|
569 |
9.48
|
0.639
|
|
|
|
|
Benchmark 0.3 |
Benchmark 5 |
|
Benchmark 60 |
Benchmark 890 |
As this study collected fish opportunistically, there was some variation between species, trophic levels, and feeding zones. All fish were small, with the largest fish not exceeding about 127 mm in length. Most fish were Cyprinid minnows, but white sucker (Catostomus commersonii) were also collected. Whole fish were analyzed, so information on where in or on the body the microplastics particles had accumulated is not available. All five fish samples analyzed had microplastics present, with particle counts ranging from 199 to 1168 per individual.109 Without information on the health of the fish analyzed (e.g., inflammation presence/absence, location of the particles, abnormalities) and if we extend the proposed benchmarks to apply to these fish, 100% of all particles found would be within the proposed benchmark cutoff for food dilution (<0.25 cm2). The tissue translocation results would be similar to the food dilution results for fish; using the particle size benchmark for tissue translocation of <6889 µm2, 74 to 97% of the particles found within the individual fish would fall within the benchmark.
Potential future work
As the target analytes were not identical between water, bed sediment, and fish samples due to method and funding availability, it would be beneficial for future work to include as much analysis cross-over as possible. For this study, microplastics and PFAS were measured in all three matrices (i.e., stream water, stream bed sediment, fish). Water sample results show that microplastics and PFAS were prevalent in wadable Iowa streams influenced by diverse agricultural activities and human wastewater contributions. A more complete understanding of environmental exposures, however, is obtained when additional environmental matrices are included. For example, the trends between sample types were substantially different for microplastics (bed sediment > water > fish) compared to that observed for total PFAS (fish > bed sediment > water). Although these two classes of CGCs are very different—microplastics are physical particles, PFAS are chemicals—they both were found to be ubiquitous in environmental compartments in wadable streams across Iowa. In future research, the collection of additional aquatic and terrestrial species to better understand their trophic transfer through the food web is warranted.35,132,133 Additionally, the present study has limitations based on the duration, scope, and resources available that may make future work valuable to build on the findings herein. For example, although this work focused on wadable streams throughout the agriculturally intensive state of Iowa, consideration of other land uses across different land use types and other regions, as well as other aquatic species to consider for biological endpoints is beneficial. For example, including ecological assessment of other aquatic species relevant to the trophic structure of wadable streams may expand the understanding of effects in agroecosystems. Specifically, the inclusion of additional biological endpoints, such as assessing the health of fish (e.g., inflammation or immune function), may enhance our understanding of the ecological impacts of these contaminants.134 Finally, more detailed assessments of potential sources of CGC and exposure routes in wadable streams in agroecosystems, including potential for eventual human exposure through water, food, and contact may also be beneficial. We frame this current work as the first concurrent, multi-matrix study of wadable streams in Iowa as a representative agriculturally intensive water system, but future efforts as described may improve the detailed understanding, implications, and generalizability of the findings.
Data availability
All data are available in the ESI,† a data release,109 and/or the U.S. Geological Survey National Water Information System (NWIS) database.135 All data from analyses for water done at the NWQL (pesticides, PFAS, and nutrients) and PFAS analyses for bed sediment and fish done at SGS Axys are available in NWIS using the site numbers in Table S1.† Data are presented as rounded to 3 significant figures in this publication.
Disclaimer
Any use of trade, firm, or product names is for descriptive purposes only and does not imply endorsement by the U.S. Government.
Author contributions
Shannon Meppelink: project conceptualization, data collection/analysis, writing – original draft preparation. Dana Kolpin: project conceptualization, data analysis, writing – original draft preparation, reviewing and editing. Gregory LeFevre: project conceptualization, laboratory analysis, original draft preparation, reviewing and editing. David Cwiertny: project conceptualization, writing – reviewing and editing, funding acquisition. Carrie Givens: project conceptualization, laboratory analysis, writing – reviewing and editing. Lee Ann Green: laboratory analysis, writing – reviewing and editing. Laura Hubbard: project conceptualization, writing – reviewing and editing. Luke Iwanowicz: laboratory analysis, writing – reviewing and editing. Rachael Lane: laboratory analysis, writing – reviewing and editing. Alyssa Mianecki: laboratory analysis, writing – reviewing and editing. Padraic O'Shea: statistical analysis, writing – reviewing and editing. Clayton Raines: laboratory analysis, writing – reviewing and editing. John Scott: laboratory analysis, writing – reviewing and editing. Darrin Thompson: project conceptualization, writing – reviewing and editing, funding acquisition. Michaelah Wilson: laboratory analysis, writing – reviewing and editing. James Gray: laboratory analysis, original draft preparation, reviewing and editing.
Conflicts of interest
All authors declare that they have no conflicts of interest.
Acknowledgements
This study originated as a pilot project funded by The University of Iowa Center for Health Effects of Environmental Contamination (CHEEC) through its funding from the State of Iowa and the Department of Natural Resources. The USGS Ecosystems Mission Area Environmental Health Program Food Resources Lifecycle Integrated Science Team, the Central Midwest Water Science Center, and the University of Iowa helped with project analytics and staffing. David Warweg (USGS), Tyler Monson (USGS), and Grant Hemphill (University of Iowa) assisted with fieldwork. Kendra Markland (USGS) and Miya Barr (USGS) assisted with data compilation and review. Kristin Romanok (USGS) provided ToxCast guidance.
References
- J. L. Wilkinson, A. B. A. Boxall, D. W. Kolpin, K. M. Y. Leung, R. W. S. Lai and C. Galbán-Malagón,
et al., Pharmaceutical pollution of the world's rivers, Proc. Natl. Acad. Sci. U. S. A., 2022, 119, e2113947119, DOI:10.1073/pnas.2113947119.
- Y. Yang, X. Zhang, J. Jiang, J. Han, W. Li and X. Li,
et al., Which micropollutants in water environments deserve more attention globally?, Environ. Sci. Technol., 2022, 56, 13–29, DOI:10.1021/acs.est.1c04250.
- P. M. Bradley, C. A. Journey, K. M. Romanok, L. B. Barber, H. T. Buxton and W. T. Foreman,
et al., Expanded target-chemical assessment reveals extensive mixed-organic-contaminant exposure in U.S. streams, Environ. Sci. Technol., 2017, 51, 4792–4802, DOI:10.1021/acs.est.7b00012.
- B. I. Escher, H. M. Stapleton and E. L. Schymanski, Tracking complex mixtures of chemicals in our changing environment, Science, 2020, 367, 388–392, DOI:10.1126/science.aay6636.
- A. Kortenkamp and M. Faust, Regulate to reduce chemical mixture risk, Science, 2018, 361, 224–226, DOI:10.1126/science.aat9219.
- G. T. Ankley, D. Feifarek, B. Blackwell, J. E. Cavallin, K. M. Jensen and M. D. Kahl,
et al., Re-evaluating the significance of estrone as an environmental estrogen, Environ. Sci. Technol., 2017, 51, 4705–4713, DOI:10.1021/acs.est.7b00606.
- J. M. Conley, N. Evans, M. C. Cardon, L. Rosenblum, L. R. Iwanowicz and P. C. Hartig,
et al., Occurrence and in vitro bioactivity of estrogen, androgen, and glucocorticoid compounds in a nationwide screen of United States stream waters, Environ. Sci. Technol., 2017, 51, 4781–4791, DOI:10.1021/acs.est.6b06515.
-
Proceedings of the International Research Workshop on the Occurrence, Effects and Fate of Microplastic Marine Debris, ed. C. Arthur, J. E. Baker and H. A. Bamford, University of Washington Tacoma, Tacoma, WA, USA, NOAA Technical Memorandum NOS-OR&R-30, 2009, https://repository.library.noaa.gov/view/noaa/2509 Search PubMed.
- C. Schmidt, T. Krauth and S. Wagner, Export of plastic debris by rivers into the sea, Environ. Sci. Technol., 2017, 51, 12246–12253, DOI:10.1021/acs.est.7b02368.
- R. N. Cable, D. Beletsky, R. Beletsky, K. Wigginton, B. W. Locke and M. B. Duhaime, Distribution and modeled transport of plastic pollution in the Great Lakes, the world's largest freshwater resource, Front. Environ Sci., 2017, 5, 1–18, DOI:10.3389/fenvs.2017.00045.
- T. J. Hoellein, A. R. McCormick, J. Hittie, M. G. London, J. W. Scott and J. J. Kelly, Longitudinal patterns of microplastic concentration and bacterial assemblages in surface and benthic habitats of an urban river, Freshw. Sci., 2017, 36, 491–507, DOI:10.1086/693012.
- E. C. Martin, C. Sorell, J. Avila, S. Behrens, D. Berry and L. Cona,
et al., Assessment of microplastics in the Great Plains: Comparing densities in water and benthic sediment across Kansas, Trans. Kans. Acad. Sci., 2019, 122, 281–287, DOI:10.1660/062.122.0315.
- M. A. Browne, A. Dissanayake, T. S. Galloway, D. M. Lowe and R. C. Thompson, Ingested microscopic plastic translocates to the circulatory system of the mussel, Mytilus edulis (L.), Environ. Sci. Technol., 2008, 42, 5026–5031, DOI:10.1021/es800249a.
- J. Zhou and R. Xia, Leafy vegetable assimilation of atmospheric microplastics/nanoplastics: An overlooked source in human food?, Environ. Sci. Technol. Lett., 2024, 11, 51–53, DOI:10.1021/acs.estlett.3c00887.
- S. Liu, X. Liu, J. Guo, R. Yang, H. Wang and Y. Sun,
et al., The association between microplastics and microbiota in placenta and meconium: The first evidence in humans, Environ. Sci. Technol., 2023, 57, 17774–17785, DOI:10.1021/acs.est.2c04706.
- L. Zhu, M. Ma, X. Sun, Z. Wu, Y. Yanyan and Y. Kang,
et al., Microplastics entry into the blood by infusion therapy: Few but a direct pathway, Environ. Sci. Technol. Lett., 2024, 11, 67–72, DOI:10.1021/acs.estlett.3c00905.
- X. Long, T. M. Fu, X. Yang, Y. Tang, Y. Zheng and L. Zhu,
et al., Efficient atmospheric transport of microplastics over Asia and adjacent oceans, Environ. Sci. Technol., 2022, 56, 6243–6252, DOI:10.1021/acs.est.1c07825.
- X. Xu, T. Li, J. Zhen, Y. Jiang, X. Nie and Y. Wang,
et al., Characterization of microplastics in clouds over Eastern China, Environ. Sci. Technol. Lett., 2024, 11, 16–22, DOI:10.1021/acs.estlett.3c00729.
- E. A. Hasenmueller, T. Baraza, N. F. Hernandez and C. R. Finegan, Cave sediment sequesters anthropogenic microparticles (including microplastics and modified cellulose) in subsurface environments, Sci. Total Environ., 2023, 893, 1–13, DOI:10.1016/j.scitotenv.2023.164690.
- C. L. Waller, H. J. Griffiths, C. M. Waluda, S. E. Thorpe, I. Loaiza and B. Moerno,
et al., Microplastics in the Antarctic marine system: an emerging area of research, Sci. Total Environ., 2017, 598, 220–227, DOI:10.1016/j.scitotenv.2017.03.283.
-
G. A. Wetherbee, A. K. Baldwin and J. F. Ranville, It is raining plastic, Open-File Report, U.S. Geological Survey, 2019, p. 1048, DOI:10.3133/ofr20191048.
- R. Geyer, J. R. Jambeck and K. L. Law, Production, use, and fate of all plastics ever made, Sci. Adv., 2017, 3, 1–5, DOI:10.1126/sciadv.1700782.
- A. K. Baldwin, S. R. Corsi and S. A. Mason, Plastic debris in 29 Great Lakes tributaries: Relations to watershed attributes and hydrology, Environ. Sci. Technol., 2016, 50, 10377–10385, DOI:10.1021/acs.est.6b02917.
- S. Wagner, P. Klöckner, B. Stier, M. Römer, B. Seiwert and T. Reemtsma,
et al., Relationship between discharge and river plastic concentrations in rural and an urban catchment, Environ. Sci. Technol., 2019, 53, 10082–10091, DOI:10.1021/acs.est.9b03048.
- R. A. Castaneda, S. Aviljas, M. A. Simard and A. Ricciardi, Microplastic pollution in St. Lawrence River sediments, Can. J. Fish. Aquat. Sci., 2014, 71, 1767–1771, DOI:10.1139/cjfas-2014-0281.
- P. L. Corcoran, S. L. Belontz, K. Ryan and M. J. Walzak, Factors controlling the distribution of microplastic particles in the benthic sediment of the Thames River, Canada, Environ. Sci. Technol., 2019, 54, 818–825, DOI:10.1021/acs.est.9b04896.
- Y. Gao, R. Li, D. Li, H. Gui, T. Chen and Z. Zhang,
et al., Spatial distribution of microplastics in water and sediments of main rivers in Taihu Lake basin, Environ. Sci.: Water Res. Technol., 2023, 3, 2151–2160, DOI:10.1021/acsestwater.2c00658.
- T. Jin, J. Tang, H. Lyu, L. Wang, A. B. Gillmore and S. M. Schaeffer, Activities of microplastics (MPs) in agricultural soil: A review of MPs pollution from the perspective of agricultural ecosystems, J. Agric. Food Chem., 2022, 70, 4182–4201, DOI:10.1021/acs.jafc.1c07849.
- E. L. Ng, L. E. Huerta, S. M. Eldridge, P. Johnston, H. W. Hu and V. Geissen,
et al., An overview of microplastic and nanoplastic pollution in agroecosystems, Sci. Total Environ., 2018, 627, 1377–1388, DOI:10.1016/j.scitotenv.2018.01.341.
- L. Nizzetto, M. Futter and S. Langaas, Are agricultural soils dumps for microplastics of urban origin?, Environ. Sci. Technol., 2016, 50, 10777–10779, DOI:10.1021/acs.est.6b04140.
- F. Radford, A. Horton, M. Hudson, P. Shaw and I. Williams, Agricultural soils and microplastics: Are biosolids the problem? Front, Soil Sci., 2023, 2, 1–14, DOI:10.3389/fsoil.2022.941837.
- S. Ziajahromi, N. Slynkova, J. Dwyer, M. Griffith, M. Fernandes, J. E. Jaeger and F. D. L. Leusch, Comprehensive assessment of microplastics in Australian biosolids: Abundance, seasonal variation and potential transport to agroecosystems, Water Res., 2024, 250, 121071, DOI:10.1016/j.watres.2023.121071.
- S. Kurwadkar, J. Dane, S. R. Kanel, M. N. Nadagouda, R. W. Cawdrey and B. Ambade,
et al., Per- and polyfluoroalkyl substances in water and wastewater: A critical review of their global occurrence and distribution, Sci. Total Environ., 2022, 809, 151003, DOI:10.1016/j.scitotenv.2021.151003.
- N. Barbo, T. Stoiber, O. V. Naidenko and D. Q. Andrews, Locally caught freshwater fish across the United States are likely a significant source of exposure to PFOS and other perfluorinated compounds, Environ. Res., 2023, 220, 115165, DOI:10.1016/j.envres.2022.115165.
- K. E. Hopkins, M. A. McKinney, A. Saini, R. J. Letcher, N. K. Karouna-Renier and K. J. Fernie, Characterizing the movement of per- and polyfluoroalkyl substances in an avian aquatic-terrestrial food web, Environ. Sci. Technol., 2023, 57, 20249–20260, DOI:10.1021/acs.est.3c06944.
- S. Lasee, S. Subbiah, W. A. Thompson, A. Karnjanapiboonwong, J. Jordan and P. Payton,
et al., Plant uptake of per- and polyfluoroalkyl acids under a maximum bioavailability scenario, Environ. Toxicol. Chem., 2019, 38, 2497–2502, DOI:10.1002/etc.4571.
- C. A. McDonough, W. Li, H. N. Bischel, A. O. De Silva and J. C. DeWitt, Widening the lens on PFASs: Direct human exposure to perfluoroalkyl acid precursors (pre-PFAAs), Environ. Sci. Technol., 2022, 56, 6004–6013, DOI:10.1021/2Facs.est.2c00254.
- F. Nielsen, F. C. Fischer, P. M. Leth and P. Grandjean, Occurrence of major perfluorinated alkylate substances in human blood and target organs, Environ. Sci. Technol., 2024, 58, 143–149, DOI:10.1021/acs.est.3c06499.
- E. Beglarian, E. Costello, D. I. Walker, H. Wang, T. L. Alderete and Z. Chen,
et al., Exposure to perfluoroalkyl substances and longitudinal changes in bone mineral density in adolescents and young adults: a multi-chohort study, Environ. Res., 2023, 244, 117611, DOI:10.1016/j.envres.2023.117611.
- A. C. Behr, C. Plinsch, A. Braeuning and T. Buhrke, Activation of human nuclear receptors by perfluoroalkylated substances (PFAS), Toxicol. In Vitro, 2020, 62, 104700, DOI:10.1016/j.tiv.2019.104700.
- N. Hamid, M. Junaid, R. Manzoor, M. Sultan, O. M. Chuan and J. Wang, An integrated assessment of ecological and human health risks of per- and polyfluoroalkyl substances through toxicity prediction approaches, Sci. Total Environ., 2023, 905, 167213, DOI:10.1016/j.scitotenv.2023.167213.
- J. M. Madrigal, R. Troisi, H. M. Surcel, H. Öhman, J. Kivelä and H. Kiviranta,
et al., Prediagnostic serum concentrations of per- and polyfluoroalkyl substances and risk of papillary thyroid cancer in the Finnish Maternity Cohort, Int. J. Cancer, 2023, 154, 979–991, DOI:10.1002/ijc.34776.
- H. Y. Done, A. K. Venkatesan and R. U. Halden, Does the recent growth of aquaculture create antibiotic resistance threats different from those associated with land animal production in agriculture?, AAPS PharmSci, 2015, 17, 513–524, DOI:10.1208/s12248-015-9722-z.
-
J. O'Neill, Antimicrobial Resistance: Tackling a Crisis for the Health and Wealth of Nations, The Review on Antimicrobial Resistance, ed. J. O'Neill, 2014, https://amr-review.org/sites/default/files/AMR%20Review%20Paper%20-%20Tackling%20a%20crisis%20for%20the%20health%20and%20wealth%20of%20nations_1.pdf Search PubMed.
- A. Pruden, R. Pei, H. Storteboom and K. H. Carlson, Antibiotic resistance genes as emerging contaminants: Studies in northern Colorado, Environ. Sci. Technol., 2006, 40, 7445–7450, DOI:10.1021/es060413l.
- K. Liguori, I. Keenum, B. C. Davis, J. Calarco, E. Milligan, V. J. Harwood and A. Pruden, Antimicrobial resistance monitoring of water environments: a framework for standardized methods and quality control, Environ. Sci. Technol., 2022, 56, 9149–9160, DOI:10.1021/acs.est.1c08918.
- I. Schachner-Groehs, M. Koller, M. Leopold, C. Kolm, R. B. Linke and S. Jakwerth,
et al., Linking antibiotic resistance gene patterns with advanced faecal pollution assessment and environmental key parameters along 2300 km of the Danube River, Water Res., 2024, 252, 121244, DOI:10.1016/j.watres.2024.121244.
- C. E. Givens, D. W. Kolpin, L. E. Hubbard, S. M. Meppelink, D. M. Cwiertny and D. A. Thompson,
et al., Simultaneous stream assessment of antibiotics, bacteria, antibiotic resistance bacteria, and antibiotic resistance genes in an agricultural region of the United States, Sci. Total Environ., 2023, 904, 1–14, DOI:10.1016/j.scitotenv.2023.166753.
- C. Matthee, A. R. Brown, A. Lange and C. R. Tyler, Factors determining the susceptibility of fish to effects of human pharmaceuticals, Environ. Sci. Technol., 2023, 57, 8845–8862, DOI:10.1021/acs.est.2c09576.
- A. Omran, R. Baker and C. Coughlin, Differential bacteriostatic effects of sucralose on various species of environmental bacteria, ISRN Toxicol., 2013, 6, 415070, DOI:10.1155/2013/415070.
- O. I. González Peña, M. Á. López Zavala and H. Cabral Ruelas, Pharmaceuticals market, consumption trends and disease incidence are not driving the pharmaceutical research on water and wastewater, Int. J. Environ. Res. Public Health., 2021, 18, 1–37, DOI:10.3390/ijerph18052532.
- P. M. Bradley, C. A. Journey, D. T. Button, D. M. Carlisle, J. M. Clark and B. J. Mahler,
et al., Metformin and other pharmaceuticals widespread in wadeable streams of the southeastern United States, Environ. Sci. Technol. Lett., 2016, 3, 243–249, DOI:10.1021/acs.estlett.6b00170.
- R. P. Deo, Pharmaceuticals in the surface water of the USA: a review, Curr. Environ. Health Rep., 2014, 1, 113–122, DOI:10.1007/s40572-014-0015-y.
- A. Q. Naik, T. Zafar and V. K. Shrivastava, Environmental impact of the presence, distribution, and use of artificial sweeteners as emerging sources of pollution, J. Environ. Public Health., 2021, 1–11, DOI:10.1155/2021/6624569.
- A. B. A. Boxall, D. W. Kolpin, B. Halling-Sørensen and J. Tolls, Are veterinary medicines causing environmental risks?, Environ. Sci. Technol., 2003, 37, 286A–294A, DOI:10.1021/es032519b.
- E. R. Campagnolo, K. R. Johnson, A. Karpati, C. S. Rubin, D. W. Kolpin and M. T. Meyer,
et al., Antimicrobial residues in animal waste and water resources proximal to large-scale swine and poultry feeding operations, Sci. Total Environ., 2002, 299, 89–95, DOI:10.1016/S0048-9697(02)00233-4.
- A. D. DiPippa and X. Mou, Meta-analysis of the distribution of pharmaceuticals and personal care products in natural streams of United States and its correlations with anthropogenic factors, Environ. Sci.: Water Res. Technol., 2024, 4, 427–435, DOI:10.1021/acsestwater.3c00443.
- D. W. Kolpin, E. T. Furlong, M. T. Meyer, E. M. Thurman, S. D. Zaugg and L. B. Barber,
et al., Pharmaceuticals, hormones, and other organic wastewater contaminants in U.S. streams, 1999–2000: a national reconnaissance, Environ. Sci. Technol., 2002, 36, 1202–1211, DOI:10.1021/es011055j.
- L. Ma, Y. Liu, J. Xu, H. Sun, H. Chen and Y. Yao,
et al., Mass loading of typical artificial sweeteners in a pig farm and their dissipation and uptake by plants in neighboring farmland, Sci. Total Environ., 2017, 605–606, 735–744, DOI:10.1016/j.scitotenv.2017.06.027.
- Z. Wang, X. H. Zhang, Y. Huang and H. Wang, Comprehensive evaluation of pharmaceuticals and personal care products (PPCPs) in typical highly urbanized region of China, Environ. Pollut., 2015, 204, 223–232, DOI:10.1016/j.envpol.2015.04.021.
- H. Zhi, D. W. Kolpin, R. D. Klaper, L. R. Iwanowicz, S. M. Meppelink and G. H. LeFevre, Occurrence and spatiotemporal dynamics of pharmaceuticals in a temperate-region wastewater effluent-dominated stream: variable inputs and differential attenuation yield evolving complex exposure mixtures, Environ. Sci. Technol., 2020, 54, 12967–12978, DOI:10.1021/acs.est.0c02328.
- S. A. Covert, M. E. Shoda, S. M. Stackpoole and W. W. Stone, Pesticide mixtures show potential toxicity to aquatic life in U.S. streams, water years 2013–2017, Sci. Total Environ., 2020, 745, 141285, DOI:10.1016/j.scitotenv.2020.141285.
- S. Savary, L. Willocquet, S. J. Pethybridge, P. Esker, N. McRoberts and A. Nelson, The global burden of pathogens and pests on major food crops, Nat. Ecol. Evol., 2019, 3, 430–439, DOI:10.1038/s41559-018-0793-y.
- J. Riedo, C. Herzog, S. Banerjee, K. Fenner, F. Walder and M. G. A. van der Heijden,
et al., Concerted evaluation of pesticides in soils of extensive grassland sites and organic and conventional vegetable fields facilitates the identification of major input processes, Environ. Sci. Technol., 2022, 56, 13686–13695, DOI:10.1021/acs.est.2c02413.
- M. R. Douglas and J. F. Tooker, Large-scale deployment of seed treatments has driven rapid increase in use of neonicotinoid insecticides and preemptive pest management in U.S. field crops, Environ. Sci. Technol., 2015, 49, 5088–5097, DOI:10.1021/es506141g.
- R. D. Sargent, J. Carrillo and C. Kremen, Common pesticides disrupt critical ecological interactions, Trends Ecol. Evol., 2023, 38, 207–210, DOI:10.1016/j.tree.2022.12.002.
- B. J. Mahler, L. H. Nowell, M. W. Sandstrom, P. M. Bradley, K. M. Romanok and C. P. Konrad,
et al., Inclusion of pesticide transformation products is key to estimating pesticide exposures and effects in small U.S. streams, Environ. Sci. Technol., 2021, 55, 4740–4752, DOI:10.1021/acs.est.0c06625.
- L. B. Ellis, K. Molina, C. R. Robbins, M. Freisthler, D. Sgargi and D. Mandrioli,
et al., Adult organophosphate and carbamate insecticide exposure and sperm concentration: A systematic review and meta-analysis of the epidemiological evidence, Environ. Health Perspect., 2023, 131, 116001, DOI:10.1289/ehp12678.
- F. B. Green, E. M. Peterson, A. D. Emert, S. Subbiah and P. N. Smith, Bee pollinator mortality due to pesticide-laden particulate matter from beef cattle feedyards, Environ. Sci. Technol., 2023, 57, 14839–14848, DOI:10.1021/acs.est.3c03135.
- L. H. Nowell, P. W. Moran, I. R. Waite, T. S. Schmidt, P. M. Bradley and B. J. Mahler,
et al., Multiple lines of evidence point to pesticides as stressors affecting invertebrate communities in small streams in five United States regions, Sci. Total Environ., 2024, 915, 169634, DOI:10.1016/j.scitotenv.2023.169634.
- S. Thompson, B. Ritz, M. Cockburn and J. E. Heck, Prenatal ambient pesticide exposure and childhood retinoblastoma, Int. J. Hyg. Environ. Health., 2022, 246, 114025, DOI:10.1016/j.ijheh.2022.114025.
- G. Weber, N. Christmann, A. C. Thiery, D. Martens and J. Kubiniok, Pesticides in agricultural headwater streams in southwestern Germany and effects on macroinvertebrate populations, Sci. Total Environ., 2018, 619–620, 638–648, DOI:10.1016/j.scitotenv.2017.11.155.
- E. M. Thurman, D. A. Goolsby, M. T. Meyer, M. S. Mills, M. L. Pomes and D. W. Kolpin, A reconnaissance study of herbicides and their metabolites in surface water of the midwestern United States using immunoassay and gas chromatography/mass spectrometry, Environ. Sci. Technol., 1992, 26, 2440–2447, DOI:10.1021/es00036a016.
- S. K. Haack, J. W. Duris, L. R. Fogarty, D. W. Kolpin, M. J. Focazio and E. T. Furlong,
et al., Comparing wastewater chemicals, indicator bacteria concentrations, and bacterial pathogen genes as fecal pollution indicators, J. Environ. Qual., 2009, 8, 248–258, DOI:10.2134/jeq2008.0173.
- J. Wang, X. Liu, A. H. W. Beusen and J. J. Middelburg, Surface-water nitrate exposure to world populations has expanded and intensified during 1970–2010, Environ. Sci. Technol., 2023, 57, 19395–19406, DOI:10.1021/acs.est.3c06150.
- M. Bieroza, L. Hallberg, J. Livsey, L. A. Prischl and M. Wynants, Recognizing agricultural headwaters as critical ecosystems, Environ. Sci. Technol., 2024, 58, 4852–4858, DOI:10.1021/acs.est.3c10165.
- P. M. Bradley, C. A. Journey, K. M. Romanok, S. E. Breitmeyer, D. T. Button and D. M. Carlisle,
et al., Multi-region assessment of chemical mixture exposures and predicted cumulative effects in USA wadeable urban/agriculture-gradient streams, Sci. Total Environ., 2021, 773, 145062, DOI:10.1016/j.scitotenv.2021.145062.
- J. B. Tuthill and A. Kaleita, Mapping the nexus: A county-level analysis and visualization of Iowa's food-energy-water systems, Sustainability, 2024, 16, 5591, DOI:10.3390/su16135591.
-
U.S. Department of Agriculture, 2024 Iowa Agricultural Statistics, 2025, p. 109, https://www.nass.usda.gov/Statistics_by_State/Iowa/Publications/Annual_Statistical_Bulletin/2024-Iowa-Annual-Bulletin.pdf Search PubMed.
-
Multi-Resolution Land Characteristics Consortium, National Land Cover Database, 2023, https://www.mrlc.gov/data?f%5B0%5D=year%3A2022 Search PubMed.
-
Iowa Geospatial Data Clearinghouse, Animal Feeding Operations Siting Atlas, 2023, https://geodata.iowa.gov/app/iowadnr::animal-feeding-operations-siting-atlas Search PubMed.
-
U.S. Census Bureau, Census 2010 Directory, 2023, https://www2.census.gov/census_2010/ Search PubMed.
-
Iowa Department of Natural Resources, Facility Explorer, 2023, https://facilityexplorer.iowadnr.gov/ Search PubMed.
-
S. R. Moulton II, J. G. Kennen, R. M. Goldstein and J. A. Hambrook, Revised protocols for sampling algal, invertebrate, and fish communities as part of the National Water Quality Assessment Program, U.S. Geological Survey Open-File Report 02-150, 2002, DOI:10.3133/ofr2002150.
- J. M. Wilson, R. M. Bunte and A. J. Carty, Evaluation of rapid cooling and tricaine methanesulfonate (MS222) as methods of euthanasia in zebrafish (Danio rerio), J. Am. Assoc. Lab. Anim. Sci., 2009, 48, 785–789 CAS , https://www.researchgate.net/publication/40024258_Evaluation_of_Rapid_Cooling_and_Tricaine_Methanesulfonate_MS222_as_Methods_of_Euthanasia_in_Zebrafish_Danio_rerio.
-
U.S. Geological Survey, Collection of water samples. Techniques of Water-Resources Investigations, Chapter A4, 2006, https://pubs.usgs.gov/twri/twri9a4/twri9a4_Chap4_v2.pdf Search PubMed.
-
J. Scott and L. Green, Development and demonstration of a superior method for microplastics analysis: improved size detection limits, greater density limits, and more informative reporting, TR Series (Illinois Sustainable Technology Center), TR-078, 2020, https://www.ideals.illinois.edu/items/115402 Search PubMed.
-
J. Masura, J. E. Baker and G. D. Foster, Laboratory methods for the analysis of microplastics in the marine environment: recommendations for quantifying synthetic particles in waters and sediments, NOAA Technical Memorandum NOS-OR&R-48, 2015, https://repository.library.noaa.gov/view/noaa/10296 Search PubMed.
- A. F. Prada, J. W. Scott, L. Green and T. J. Hoellein, Microplastics and per- and polyfluoroalkyl substances (PFAS) in landfill-wastewater treatment systems: A field study, Sci. Total Environ., 2024, 954, 176751, DOI:10.1016/j.scitotenv.2024.176751.
- D. W. Kolpin, L. E. Hubbard, D. M. Cwiertny, S. M. Meppelink, D. A. Thompson and J. L. Gray, A comprehensive statewide spatiotemporal stream assessment of per- and polyfluoroalkyl substances (PFAS) in an agricultural region of the United States, Environ. Sci. Technol. Lett., 2021, 8, 981–988, DOI:10.1021/acs.estlett.1c00750.
-
SGS-Axys, SGS Axys Analysis & Sampling Support, 2024, https://www.sgsaxys.com/sampling-analysis/pfas/ Search PubMed.
-
M. W. Sandstrom, L. K. Kanagy, C. A. Anderson and C. J. Kanagy, Determination of pesticides and pesticide degradates in filtered water by direct aqueous-injection liquid chromatography-tandem mass spectrometry. U.S. Geol. Surv. Tech. Methods, 2015, p. 54, DOI:10.3133/tm5B11.
- G. H. LeFevre, C. E. Müller, R. J. Li, R. G. Luthy and E. S. Sattely, Rapid phytotransformation of benzotriazole generates synthetic tryptophan and auxin analogs in arabidopsis, Environ. Sci. Technol., 2015, 49, 10959–10968, DOI:10.1021/acs.est.5b02749.
- S. Ciparis, L. R. Iwanowicz and J. R. Voshell, Effects of watershed densities of animal feeding operations on nutrient concentrations and estrogenic activity in agricultural streams, Sci. Total Environ., 2012, 414, 268–276, DOI:10.1016/j.scitotenv.2011.10.017.
- J. Sanseverino, R. K. Gupta, A. C. Layton, S. S. Patterson, S. A. Ripp and L. Saidak,
et al., Use of Saccharomyces cerevisiae BLYES expressing bacterial bioluminescence for rapid, sensitive detection of estrogenic compounds, Appl. Environ. Microbiol., 2005, 71, 4455–4460, DOI:10.1128/aem.71.8.4455-4460.2005.
- E. J. Routledge and J. P. Sumpter, Estrogenic activity of surfactants and some of their degradation products assessed using a recombinant yeast screen, Environ. Toxicol. Chem., 1996, 15, 241–248, DOI:10.1002/etc.5620150303.
- B. Jarošová, L. Bláha, J. P. Giesy and K. Hilscherová, What level of estrogenic activity determined by in vitro assays in municipal waste waters can be considered safe?, Environ. Int., 2014, 64, 98–109, DOI:10.1016/j.envint.2013.12.009.
-
R Studio Team, RStudio: Integrated Development Environment for R (Version 4.4.0). RStudio, PBC, Boston, MA, 2024, https://rstudio.com/ Search PubMed.
-
D. R. Helsel, R. M. Hirsch, K. R. Ryberg, S. A. Archfield and E. J. Gilroy, Statistical methods in water resources. U.S. Geological Survey Techniques and Methods, 2020, p. 458, DOI:10.3133/tm4a3.
- Y. Benjamini and Y. Hochberg, Controlling the false discovery rate: A practical and powerful approach to multiple testing, J. R. Stat. Soc., B: Stat. Methodol., 1995, 57, 289–300, DOI:10.1111/j.2517-6161.1995.tb02031.x.
- M. Mehrotra, G. Wang and W. M. Johnson, Multiplex PCR for detection of genes for Staphylococcus aureus enterotoxins, exfoliative toxins, toxic shock syndrome toxin 1, and methicillin resistance, J. Clin. Microbiol., 2020, 38, 1032–1035, DOI:10.1128/jcm.38.3.1032-1035.2000.
-
U.S. Environmental Protection Agency, Toxicity Forecasting (ToxCast), 2024, https://www.epa.gov/comptox-tools/toxicity-forecasting-toxcast Search PubMed.
- N. Cedergreen, A. M. Christensen, A. Kamper, P. Kudsk, S. K. Mathiassen and J. C. Streibig,
et al., A review of independent action compared to concentration addition as reference models for mixtures of compounds with different molecular target sites, Environ. Toxicol. Chem., 2008, 27, 1621–1632, DOI:10.1897/07-474.1.
- J. Y. Liu and C. M. Sayes, Modeling mixtures interactions in environmental toxicology, Environ. Toxicol. Pharmacol., 2024, 106, 104380, DOI:10.1016/j.etap.2024.104380.
- M. Sigurnjak Bureš, Š. Ukić, M. Cvetnić, V. Prevarić, M. Markić and M. Rogošić,
et al., Toxicity of binary mixtures of pesticides and pharmaceuticals toward Vibrio fischeri: Assessment by quantitative structure-activity relationships, Environ. Pollut., 2021, 275, 115885, DOI:10.1016/j.envpol.2020.115885.
-
L. A. De Cicco, S. R. Corsi, D. L. Villeneuve, B. R. Blackwell and G. T. Ankley, toxEval: Evaluation of measured concentration data using the ToxCast high-throughput screening database or a user-defined set of concentration benchmarks, R package version 1.3.1., 2024, https://code.usgs.gov/water/toxEval Search PubMed.
-
U.S. Environmental Protection Agency, Office of Pesticide Programs-Aquatic Life Benchmarks and Ecological Risk Assessments for Registered Pesticides, 2023, https://www.epa.gov/pesticide-science-and-assessing-pesticide-risks/aquatic-life-benchmarks-and-ecological-risk Search PubMed.
- A. C. Mehinto, S. Coffin, A. A. Koelmans, S. M. Brander, M. Wagner and L. M. Thornton Hampton,
et al., Risk-based management framework for microplastics in aquatic ecosystems, Microplast. Nanoplast., 2022, 2, 1–11, DOI:10.1186/s43591-022-00033-3.
-
S. M. Meppelink, D. W. Kolpin, G. H. LeFevre, D. M. Cwiertny, C. E. Givens, L. A. Green, et al., Site information and compound concentrations for 15 Iowa streams, U.S. Geological Survey Data Release, 2021, DOI:10.5066/P14BTJR3.
- J. R. Masoner, D. W. Kolpin, I. M. Cozzarelli, L. B. Barber, D. S. Burden and W. T. Foreman,
et al., Urban stormwater: an overlooked pathway of extensive mixed contaminants to surface and groundwaters in the United States, Environ. Sci. Technol., 2019, 53, 10070–10081, DOI:10.1021/acs.est.9b02867.
- M. Scheurer, A. Michel, H. J. Brauch, W. Ruck and F. Sacher, Occurrence and fate of the antidiabetic drug metformin and its metabolite guanylurea in the environment and during drinking water treatment, Water Res., 2012, 46, 4790–4802, DOI:10.1016/j.watres.2012.06.019.
- M. L. Fork, J. B. Fick, A. J. Reisinger and E. J. Rosi, Dosing the coast: Leaking sewage infrastructure delivers large annual doses and dynamic mixtures of pharmaceuticals to urban rivers, Environ. Sci. Technol., 2021, 55, 11637–11645, DOI:10.1021/acs.est.1c00379.
- L. A. Schaider, K. M. Rodgers and R. A. Rudel, Review of organic wastewater compound concentrations and removal in onsite wastewater treatment systems, Environ. Sci. Technol., 2017, 51, 7304–7317, DOI:10.1021/acs.est.6b04778.
- B. J. Currens, A. M. Hall, G. M. Brion and A. E. Fryar, Use of acetaminophen and sucralose as co-analytes to differentiate sources of human excreta in surface waters, Water Res., 2019, 157, 1–7, DOI:10.1016/j.watres.2019.03.023.
- J. Spoelstra, S. L. Schiff and S. J. Brown, Septic systems contribute artificial sweeteners to streams through groundwater, J. Hydrol., 2020, 7, 1–8, DOI:10.1016/j.hydroa.2020.100050.
- B. Jarošová, J. Javurek, O. Adamovský and K. Hilscherová, Phytoestrogens and mycoestrogens in surface waters – Their sources, occurrence, and potential contribution to estrogenic activity, Environ. Int., 2015, 81, 26–44, DOI:10.1016/j.envint.2015.03.019.
- D. A. Alvarez, N. W. Shappell, L. O. Billey, D. S. Bermudez, V. S. Wilson and D. W. Kolpin,
et al., Bioassay of estrogenicity and chemical analyses of estrogens in streams across the United States associated with livestock operations, Water Res., 2013, 47, 3347–3363, DOI:10.1016/j.watres.2013.03.028.
- D. W. Kolpin, C. C. Hoerger, M. T. Meyer, F. E. Wettstein, L. E. Hubbard and T. D. Bucheli, Phytoestrogens and mycotoxins in Iowa streams: An examination of underinvestigated compounds in agricultural basins, J. Environ. Qual., 2010, 39, 2089–2099, DOI:10.2134/jeq2010.0121.
- D. W. Kolpin, J. Schenzel, M. T. Meyer, P. J. Phillips, L. E. Hubbard, T. M. Scott and T. D. Bucheli, Mycotoxins: Diffuse and point source contributions of natural contaminants of emerging concern to streams, Sci. Total Environ., 2014, 470–471, 669–676, DOI:10.1016/j.scitotenv.2013.09.062.
- A. Gonsioroski, V. E. Mourikes and J. A. Flaws, Endocrine disruptors in water and their effects on the reproductive system, Int. J. Mol. Sci., 2020, 21, 1929, DOI:10.3390/ijms21061929.
- K. M. Gani, V. K. Tyagi and A. A. Kazmi, Occurrence of phthalates in aquatic environment and their removal during wastewater treatment processes: a review, Environ. Sci. Pollut. Res., 2017, 24, 17267–17284, DOI:10.1007/s11356-017-9182-3.
- M. R. Gillings, S. Krishnan, P. J. Worden and S. A. Hardwick, Recovery of diverse genes for class 1 integron-integrases from environmental DNA samples, FEMS Microbiol. Lett., 2008, 287, 56–62, DOI:10.1111/j.1574-6968.2008.01291.x.
-
R. M. Hall, Mobile gene cassettes and integrons: moving antibiotic resistance genes in gram‐negative bacteria, in Ciba Foundation Symposium 207‐Antibiotic Resistance: Origins, Evolution, Selection and Spread, ed. D. J. Chadwick and J. Goode, Wiley Online Library, 2007, pp. 192–205, DOI:10.1002/9780470515358.ch12.
- M. Nardelli, P. M. Scalzo, M. S. Ramírez, M. P. Quiroga, M. H. Cassini and D. Centrón, Class 1 integrons in environments with different degrees of urbanization, PLoS One, 2012, 7, e39223, DOI:10.1371/journal.pone.0039223.
- N. Donley, C. Cox, K. Bennett, A. M. Tempkin, D. Q. Andrews and O. V. Naidenko, Forever pesticides: A growing source of PFAS contamination in the environment, Environ. Health Perspect., 2024, 132(7), 075003, DOI:10.1289/EHP13954.
- R. Jagani, H. Patel, J. Chovatiya and S. S. Andra, The rise and risks of fluorinated pesticides: A call for comprehensive research to address environmental and health concerns, J. Agric. Food Chem., 2025, 73, 2217–2220, DOI:10.1021/acs.jafc.4c12827.
- S. Lasee, K. McDermett, N. Kumar, J. Guelfo, P. Payton, Z. Yang and T. A. Anderson, Targeted analysis and total oxidizable precursor assay of several insecticides for PFAS, J. Hazard. Mater. Lett., 2022, 3, 1–7, DOI:10.1016/j.hazl.2022.100067.
- X. Qin, M. Cao, T. Peng, H. Shan, W. Lian and Y. Yu,
et al., Features, potential invasion pathways, and reproductive health risks of microplastics detected in human uterus, Environ. Sci. Technol., 2024, 58, 10482–10493, DOI:10.1021/acs.est.4c01541.
- S. R. Corsi, L. A. De Cicco, D. L. Villeneuve, B. R. Blackwell, K. A. Fay, G. T. Ankley and A. K. Baldwin, Prioritizing chemicals of ecological concern in Great Lakes tributaries using high-throughput screening data and adverse outcome pathways, Sci. Total Environ., 2019, 686, 995–1009, DOI:10.1016/j.scitotenv.2019.05.457.
- A. Mallik, K. A. M. Xavier, B. C. Naidu and B. B. Nayak, Ecotoxicological and physiological risks of microplastics on fish and their possible mitigation measures, Sci. Total Environ., 2021, 779, 146433, DOI:10.1016/j.scitotenv.2021.146433.
- M. Pirsaheb, H. Hossini and P. Makhdoumi, Review of microplastic occurrence and toxicological effects in marine environment: Experimental evidence of inflammation, Process Saf. Environ. Prot., 2020, 142, 1–14, DOI:10.1016/j.psep.2020.05.050.
- Q. Fu, C. Meyer, M. Patrick, V. Kosfeld, H. Rüdel, J. Koschorreck and J. Hollender, Comprehensive screening of polar emerging organic contaminants including PFASs and evaluation of the trophic transfer behavior in a freshwater food web, Water Res., 2022, 218, 118514, DOI:10.1016/j.watres.2022.118514.
- A. P. Roodt, M. Huszarik, M. H. Entling and R. Schulz, Aquatic-terrestrial transfer of neonicotinoid insecticides in riparian food webs, J. Hazard. Maters., 2023, 455, 131635, DOI:10.1016/j.jhazmat.2023.131635.
- J. Xiong and Z. Li, Predicting PFAS fate in fish: Assessing the roles of dietary, respiratory, and dermal uptake in bioaccumulation modeling, Environ. Res., 2024, 252, 119036, DOI:10.1016/j.envres.2024.119036.
-
U.S. Geological Survey, USGS water data for the Nation: U.S. Geological Survey National Water Information System database, 2024, DOI:10.5066/F7P55KJN.
|
This journal is © The Royal Society of Chemistry 2025 |
Click here to see how this site uses Cookies. View our privacy policy here.