DOI:
10.1039/D4AY02120G
(Paper)
Anal. Methods, 2025,
17, 2708-2717
Quantification of glucose in the plasma of healthy Chinese volunteers and a bioequivalence study using a surrogate matrix approach combined with UHPLC-MS/MS
Received
22nd November 2024
, Accepted 28th February 2025
First published on 12th March 2025
Abstract
Acarbose works by competitively inhibiting α-glucosidase, delaying the breakdown of starch into glucose. Thus, it plays an important role in treating type 2 diabetes. Acarbose exhibits unique pharmacokinetic characteristics, and the United States of America (USA) Food and Drug Administration (FDA) has focused on pharmacodynamic parameters rather than pharmacokinetic ones in acarbose bioequivalence studies since issuing its 2009 draft guidance, which was formalized in 2017. A literature review on glucose measurement shows that glucose concentration data are primarily derived from serum matrices. In the 2022 draft guidance, the FDA reaffirmed that plasma glucose concentration is the most suitable pharmacodynamic endpoint for acarbose. This study employed a surrogate matrix method combined with ultra-high performance liquid chromatography-MS/MS (UHPLC-MS/MS) to measure plasma glucose levels. Because glucose is an endogenous substance, directly measuring its concentration in biological matrices does not effectively reflect the impact of acarbose on blood glucose levels. This study used Phosphate Buffered Saline (PBS) as the surrogate matrix to determine glucose concentrations and accurately achieve precise glucose quantification. Moreover, glucose and fructose are structural isomers that are difficult to quickly separate chromatographically, which can affect detection efficiency and introduce interference. Therefore, we aim to develop a rapid, simple, and accurate method for quantifying glucose concentration in human plasma using a surrogate matrix approach combined with UHPLC-MS/MS. Plasma samples were processed using protein precipitation, with glucose-[U-13C6] as the internal standard. Chromatographic separation was performed using a Waters ACQUITY UPLC BEH amide column (100 × 2.1 mm, 1.7 μm), and gradient elution was carried out at a flow rate of 0.4 mL min−1 with a mobile phase of 0.05% aqueous ammonia and 0.05% ammonia in acetonitrile. Electrospray ionization (ESI) in negative ion multiple reaction monitoring (MRM) mode was used for detection, with a total analysis time of 6 min. Glucose exhibited good linearity in the concentration range of 2.00 to 20.00 mmol L−1 (r2 = 0.9980), with a precision and accuracy within and between batches of less than 10%. The method was fully validated to ensure data accuracy. This method was successfully applied to a bioequivalence study of acarbose tablets in healthy Chinese subjects in the fasting state, providing valuable data for evaluating the consistency of acarbose formulations.
1 Introduction
Type 2 diabetes is a prevalent chronic condition, and its global incidence is increasing.1 Conventional treatments often fail to achieve adequate control of blood glucose, frequently resulting in noticeable changes. With continued advancements in clinical research on type 2 diabetes, acarbose tablets have been increasingly adopted in disease management. Acarbose works by competitively inhibiting α-glucosidase, delaying the breakdown of starch into glucose, thus achieving a hypoglycemic effect.2
Acarbose exhibits unique pharmacokinetic characteristics, with minimal absorption following oral administration (less than 2% of acarbose is absorbed as a locally acting drug).3,4 Its serum protein binding rate is low, and it primarily acts within the gastrointestinal tract without being considerably absorbed into the bloodstream, meaning plasma drug concentrations are not directly related to its clinical efficacy. Since issuing its draft guidance in 2009, which was later formalized in 2017, the FDA has prioritized pharmacodynamic parameters over pharmacokinetic ones in acarbose bioequivalence studies due to the uniqueness of its mechanism. These studies assessed the bioequivalence based on changes in serum glucose concentration. In the 2022 draft guidance, the FDA reaffirmed that plasma glucose concentration is the most suitable pharmacodynamic endpoint for acarbose.5 A literature review on glucose measurement shows that glucose concentration data are primarily derived from serum matrices when employing techniques such as enzyme-linked colorimetric assays, microelectrode methods, oxygen rate methods, hexokinase assays, o-toluidine methods, glucose dehydrogenase assays, minimally invasive techniques, and isotope dilution mass spectrometry (gas chromatography-isotope dilution mass spectrometry, GC-IDMS; liquid chromatography-isotope dilution mass spectrometry, LC-IDMS).6–16 However, enzymatic assays may be influenced by interference from complex biological matrices, leading to significant inter-laboratory and temporal variability in the results, making these methods unsuitable for bioequivalence studies. The o-toluidine method is also problematic due to the corrosive nature of the reagents and the suspected carcinogenicity of o-toluidine, which could lead to both health risks and potential damage to instruments. Minimally invasive methods are more suitable for continuous glucose monitoring in diabetic patients but are inappropriate for plasma glucose analysis. Isotope dilution mass spectrometry (GC-IDMS, LC-IDMS) requires complex derivatization during sample preparation, making it cumbersome. Following the M10 Methods for Analytes that are also in the Endogenous Molecules Guidelines,17 this study employed a surrogate matrix method combined with UHPLC-MS/MS to measure plasma glucose levels, reflecting glycemic fluctuations and evaluating the bioequivalence of hypoglycemic agents.
Because glucose is an endogenous substance, directly measuring its concentration in biological matrices does not effectively reflect the impact of acarbose on blood glucose levels. However, finding a suitable blank matrix for endogenous substance analysis is challenging. A suitable surrogate matrix must be selected to determine glucose concentrations accurately. This study used PBS as the surrogate matrix to achieve precise glucose quantification. Moreover, glucose and fructose are structural isomers that are difficult to quickly separate chromatographically, which can affect detection efficiency and introduce interference. By optimizing the instrument acquisition method, we selected a suitable chromatographic column to successfully separate glucose from fructose and effectively eliminate any analytical error caused by the signal from fructose.
This study is the first to establish a UHPLC-MS/MS method for quantitatively determining the plasma glucose concentration using a surrogate matrix method. The method has several advantages, including fast analysis, high selectivity, high precision, high sensitivity, and minimal matrix effects. The method was fully validated to ensure data accuracy. This method was applied to a bioequivalence study of acarbose tablets in healthy Chinese subjects in the fasting state, providing valuable data for evaluating the consistency of acarbose formulations.
2 Experimental
2.1 Chemicals and reagents
The glucose reference standard (purity: 99.8%), glucose-[U-13C6] reference standard (purity: 98.0%, isotopic labeling purity: 99.0%), and fructose reference standard (purity 100%) were obtained from Beijing Manhage Bio-Technology Company (Beijing, China). HPLC-grade methanol (MeOH) and acetonitrile (ACN) were sourced from Merck KGaA (Hesse, Germany). Ammonia was of HPLC grade (Wilmington, DE, USA), and PBS buffer powder was from Dalian Meilum Biotechnology Co., Ltd (Dalian, China). Ultrapure water was produced in-house using a Milli-Q water purification system (Millipore Corp., Bedford, MA, USA). Blank human plasma and blood were sourced from the People's Hospital of Weifang High-tech Industrial Development Zone, with EDTA-K2 serving as the anticoagulant (Weifang, China).
2.2 Instruments and conditions
The liquid chromatography components of the UHPLC-MS/MS system included a high-performance liquid chromatography pump (LC-30AD), an automatic sampler (ExionLC-Rack Changer), and a column temperature box (ExionLC AD Column Oven). The mass spectrometer used was a SCIEX Triple Quad 5500 (AB SCIEX, Framingham, MA, USA). The data acquisition and quantification were conducted using Analyst™ MD 1.6.3 software (AB SCIEX, USA) and Watson 7.5 LIMS software (Thermo Fisher Scientific, USA). Chromatographic separation was performed on an LC-30AD (Shimadzu, Japan) using an HPLC Waters ACQUITY UPLC BEH amide column (100 × 2.1 mm, 1.7 μm) at a column temperature of 40 °C. The mobile phase consisted of 0.05% aqueous ammonia (A) and 0.05% ammonia in acetonitrile (B). The flow rate was set to 0.400 mL min−1, with an injection volume of 1 μL. The temperature of the automatic sampler was set to 8 °C. The initial concentration of pump B was set to 80%. The following gradient program was used for sample separation: from 0 to 4.0 min, 80–70% B; from 4.0 to 4.1 min, 70–80% B; from 4.1 to 6.0 min, 80% B. ESI was used in negative ion mode. The MRM model was utilized in detection mode, and the ion spray voltage was set to −4500 V. The turbo ion spray temperature was maintained at 500 °C, the curtain gas value was adjusted to 25.0 psi, and the collision gas (CAD) value was set to 10 psi. The nebulizing gas (gas 1) was set to a pressure of 55.0 psi, and the auxiliary gas, referred to as gas 2, was set to a pressure of 50.0 psi. The entrance potential (EP) was adjusted to −10.0 V, the collision cell exit potential (CXP) was set to −16 V, and the acquisition time for mass spectrometry was 6.0 min. The optimized mass spectrometric parameters are detailed in Table 1.
Table 1 Optimized mass spectrometric parameters
Compound name |
Multiple reaction monitoring (MRM) |
Dwell time (mms) |
Declustering power (DP) (volts) |
Collision energy (CE) (volts) |
Chromatographic retention Time (min) |
Glucose |
179.1 → 59.0 |
200 |
−40 |
−25 |
2.00 |
Glucose-[U-13C6] |
185.2 → 92.2 |
200 |
−40 |
−25 |
1.99 |
Fructose |
179.1 → 59.0 |
200 |
−40 |
−25 |
1.73 |
2.3 Stock standard solutions and working standard solutions
Accurately weighed glucose was dissolved in methanol–water (1
:
1, v/v) to prepare a stock solution with a 800.00 mmol L−1 concentration. This stock solution was then diluted with methanol–water (1
:
1, v/v) to create a range of calibration standard working solutions with concentrations of 40.00, 80.00, 120.00, 160.00, 240.00, 280.00, 320.00, and 400.00 mmol L−1. These solutions were stored at −20 °C in a freezer.
Similarly, the quality control (QC) working solutions were prepared using the same method, with glucose concentrations of 40.00, 114.00, 200.00, and 300.00, mmol L−1 and stored in a freezer at −20 °C.
Accurately weighed glucose-[U-13C6] was dissolved in methanol–water (1
:
1, v/v) to prepare a stock solution at a 21.62 mmol L−1 concentration. This stock solution was then diluted with methanol to prepare an internal standard (IS) working solution at a concentration of 0.24 mmol L−1, which was stored at −20 °C in a freezer. Accurately weighed fructose was dissolved in methanol–water (1
:
1, v/v) to make a stock solution with a 800.00 mmol L−1 concentration. This stock solution was then diluted with methanol–water (1
:
1, v/v) to prepare a working solution at a concentration of 40.00 mmol L−1, which was stored at −20 °C in a freezer.
2.4 Calibration standards and QC samples
2.4.1 Matrix preparation.
The study used phosphate-buffered saline (PBS) as the surrogate matrix, and EDTA-K2 human plasma was used as the actual matrix (referred to as human plasma or original matrix). Alternative matrix: PBS buffer powder was added to 1 L of ultrapure water to prepare a PBS buffer solution. The PBS buffer solution was stored at 2–8 °C when not in use. Actual matrix: human plasma from at least two healthy donors was mixed to prepare the real matrix, which was stored at −80 °C when not in use.
2.4.2 Calibration standards.
To prepare the calibration standards, we added 190 μL of PBS to a 2 mL EP tube, followed by 10 μL of calibration standard working solution. The mixture was vortexed at 25 ± 5 °C to obtain final concentrations of 2.00, 4.00, 6.00, 8.00, 12.00, 14.00, 16.00, and 20.00 mmol L−1.
2.4.3 QC samples.
Two sets of QC samples were prepared using the surrogate matrix (PBS) and the actual matrix (human plasma). The first set of QC samples was prepared by adding QC working solutions to the surrogate matrix, resulting in six replicates each for the lower limit of quantification (LLOQ, 2.00 mmol L−1), low-concentration QC (LQC, 5.70 mmol L−1), medium-concentration QC (MQC, 10.0 mmol L−1), and high-concentration QC (HQC, 15.0 mmol L−1). The accuracy and precision of these QC samples were evaluated using the surrogate matrix calibration curve. The second set of QC samples was obtained by adding the QC working solution to the actual matrix (human plasma) or diluting the actual matrix with PBS. First, the endogenous glucose concentration in human plasma was measured using the calibration curve derived from the surrogate matrix (n = 8). The endogenous glucose concentration in the actual matrix was 5.70 mmol L−1, which was used as a LQC sample for the actual matrix. The LLOQ QC sample (2.00 mmol L−1) was prepared by diluting the actual matrix with the surrogate matrix (PBS). The MQC (10.0 mmol L−1) and HQC (15.0 mmol L−1) were prepared by adding an appropriate amount of QC working solution to the actual matrix. Six replicates were prepared for each QC concentration sample.
2.5 Sample preparation
We accurately pipetted 50 μL of the sample into a 2 mL deep-well 96-well plate. Then, 450 μL of internal standard (IS) working solution (0.24 mmol L−1, dilution solution: methanol) was added, and the sample was vortexed for a duration of 5 min and subsequently centrifuged at a temperature of 4 °C and 4750 rpm for 10 min. Then, 20 μL of the supernatant from each well was transferred to a 96-well plate containing 480 μL of acetonitrile/water (40
:
60, v/v), followed by vortex mixing for 5 min. Finally, 1 μL of the supernatant was injected into the LC-MS/MS system for analysis.
2.6 Method validation
Because glucose is an endogenous substance, obtaining a blank matrix free from endogenous glucose is challenging. Therefore, this study developed a surrogate matrix method using PBS to replace blank plasma to quantify glucose accurately. The validation process adhered to the M10 guideline standards for bioanalytical method validation.17
2.6.1 Selectivity.
After examining the background of normal blank plasma from six different sources of normal blank plasma, one source of hemolyzed blank plasma, and one source of hyperlipemia blank plasma, MQC samples were prepared using blank plasma to evaluate the selectivity. The accuracy for the MQC plasma samples was maintained within a range of ±15% of the nominal value, and the precision of the measurements did not exceed 15%.
The interference between the analyte and IS was evaluated by preparing plasma samples at the upper limit of quantification (ULOQ) without the IS, ULOQ surrogate matrix samples, LLOQ plasma samples, LLOQ surrogate matrix samples, and zero concentration surrogate matrix samples. The acceptance criteria were as follows. The response of the IS at the retention time in ULOQ plasma and ULOQ surrogate matrix samples should be less than 5% of the average IS response in the zero concentration surrogate matrix sample. Moreover, the response at the analyte's retention time in the zero concentration surrogate matrix sample should be less than 20% of the average analyte response in LLOQ plasma and LLOQ surrogate matrix samples. The average accuracy of the LLOQ plasma and LLOQ surrogate matrix samples should fall within ±20% of the nominal value.
2.6.2 Specificity.
This study prepared a plasma sample containing both fructose and glucose. The fructose concentration was set to be consistent with the upper limit of quantification (ULOQ, 20.00 mmol L−1) of glucose sample concentration, and the glucose concentration was made equivalent to that of the sample at the lower limit of quantification (LLOQ, 2.00 mmol L−1) to evaluate the interference of fructose with glucose quantification. The average accuracy of the LLOQ samples was within ±20% of the nominal value, and the precision did not exceed 20%.
2.6.3 Carryover.
Carryover was assessed in each analytical run by analyzing double blank samples (without an analyte and IS) after the calibration standard of the ULOQ samples. The carry-over in double blank samples following the ULOQ samples should not exceed 20% of the analyte response in LLOQ samples and 5% of the IS response.
2.6.4 Calibration curve.
Standard curve samples at eight concentration levels were prepared using PBS, with two replicates for each concentration. The standard curve was fitted in each analytical run using two calibration points. Calibration curves were generated using 1/X2 weighted least squares linear regression models, which plotted the peak-area ratios of each analyte to its corresponding IS against the nominal analyte concentrations. The calibration curve's correlation coefficient (r2) was above 0.9900, and the signal-to-noise ratio (S/N) for the LLOQ was above 10. The accuracy of the back-calculated concentrations of each calibration standard should be within ±20% of the nominal concentration at the LLOQ and ±15% at all other levels.
2.6.5 Precision and accuracy.
The precision and accuracy for both the surrogate matrix and actual matrix were determined by analyzing QC samples within each analytical run (within runs) and in different runs (between runs). Within-run precision and accuracy should be assessed by analyzing a minimum of five replicates at each QC concentration level in each analytical run. Within-run precision and accuracy were assessed by analyzing six replicates of QC samples at each concentration level in every run. Between-run precision and accuracy were evaluated by analyzing each QC concentration level in at least three analytical runs over at least two days. The overall accuracy at each concentration level should be within ±15% of the nominal concentration, except for the LLOQ, where it should be within ±20%. The precision (%CV) of the concentrations determined at each level should not exceed 15%, except at the LLOQ, where it should not exceed 20%.
2.6.6 Matrix effect.
The influence of matrix effects in the surrogate and actual matrices were evaluated. The matrix effect was evaluated by analyzing at least three replicates of LQC and HQC samples, each prepared utilizing matrices from six different sources of normal blank plasma, one source of hemolyzed blank plasma, one source of hyperlipemia blank plasma, and one source of the surrogate matrix. For each matrix source, the accuracy should be maintained within ±15% of the nominal concentration, and the precision (percent coefficient of variation (%CV)) should not exceed 15% in all individual matrix sources.
2.6.7 Extraction recovery.
Extraction recovery in both the surrogate and actual matrices was evaluated. Three concentration levels of QC samples (LQC: 5.70 mmol L−1, MQC: 10 mmol L−1, and HQC: 15 mmol L−1) (n = 3) were used. The peak areas of glucose or glucose-[U-13C6] in the extracted QC samples were compared with that of the extracted blank surrogate and actual matrix samples containing the corresponding concentration of glucose or glucose-[U-13C6] with a requirement of CV ≤ 15%. While 100% recovery is not required, the extraction recovery in the surrogate matrix and actual matrix should be consistent.
2.6.8 Dilution integrity.
The dilution integrity of the DQC samples (40.0 mmol L−1) was evaluated after a five-fold dilution using the surrogate matrix with six replicates. The final concentration must fall within the linear range of the standard curve. The average accuracy of the dilution QC samples should be within ±15% of the nominal value, with a precision (%CV) not exceeding 15%.
2.6.9 Stability.
The stability of the analyte under various conditions in the stock solution, working solution, and matrix was assessed by simulating the environments to which samples might be exposed during analysis.
The stabilities of the stock and working solutions for the analyte were evaluated using the lowest and highest concentrations. The deviation between the reference and control samples should not exceed 10.0%. The stability of the analyte in the matrix was tested with three replicates at two concentration levels (LQC and HQC). The stability tests included the analyte's stability in whole blood, the short-term and long-term stabilities of the analyte in the matrix, the freeze–thaw stability (from −80 °C to 25 ± 5 °C for at least three freeze–thaw cycles), the stability of processed samples in an autosampler, and the reinjection reproducibility.
The stability QCs are analyzed against a calibration curve obtained from freshly spiked calibration standards in a run with its corresponding freshly prepared QCs or QCs for which stability was previously confirmed. The mean concentration at each QC level should be within ±15% of the nominal concentration.
2.7 Incurred sample re-analysis (ISR)
Using calibration standards and QC samples in method validation cannot fully simulate the actual study samples, so the actual study samples must be analyzed in a separate analytical batch. ISR samples should ideally cover each subject and each study period, with samples selected from time points around the peak concentration (Cmax) and during the elimination phase. The percent difference between the initial concentration and the concentration measured during the repeat analysis should be calculated using the formula: %Difference = ((repeat value − initial value)/mean value) × 100, where the mean value is the average of the initial and repeat values. The percent difference should not exceed 20% for at least two-thirds of the repeats.
2.8 Pharmacodynamics study
This study enrolled 16 healthy Chinese male and female subjects aged 18 years and above with a body mass index (BMI) between 19.0 and 24.0 kg m−2. All subjects voluntarily provided informed consent after discussing the details with the investigators. Each subject's eligibility was confirmed by reviewing their medical history, complete blood count results, electrocardiogram (ECG), fasting blood glucose, 2 h postprandial blood glucose, glycated hemoglobin (HbA1c), and physical examination.
This study followed a single-center, single-dose, randomized, open-label, two-period, two-sequence, crossover design to assess bioequivalence under fasting conditions, with a 7-day washout period between doses. Subjects were admitted to the research center 2 days before each dosing period. After achieving a standardized and balanced diet, the subjects fasted overnight. On the morning of day 1 in each period, subjects received a sucrose solution containing 75 g of sucrose dissolved in 150 mL of water on an empty stomach, and subsequently, pharmacodynamics blood samples were collected for up to 4 h post-ingestion. On an empty stomach the following morning, while seated, the subjects took either the test or reference formulation of acarbose tablets (two tablets of 50 mg) with the same sucrose solution, and subsequently, pharmacodynamic blood samples were collected again for 4 h post-dosing. Venous blood (3 mL) was drawn via an indwelling catheter 20 min before and a10, 20, 30, 40, 50, 60, 70, 80, 90, 100, 110, 120, 150, 180, 210, and 240 min after administration of the sucrose solution or sucrose/acarbose cotreatment. Blood samples were collected into EDTA-K2 anticoagulant vacuum tubes. Whole blood was centrifuged within 1 h of collection (2000g, 4 °C, 10 min), and the separated plasma samples were stored in an ultra-low temperature freezer (≤−60 °C) until further analysis.
3 Results and discussion
3.1 Method development and optimization
While LC-MS/MS provides high selectivity and sensitivity, several challenges remain in analyzing glucose and fructose. For instance, glucose and fructose have identical molecular weights, making it impossible for a mass spectrometer to distinguish them based on their parent ions. Moreover, their ion fragments are identical, meaning the mass spectrometer cannot differentiate them based on fragmentation patterns. Therefore, accurate quantification of glucose requires effective chromatographic separation from its isomers. In this study, an amide column with a smaller particle size (1.7 μm) was used to separate glucose from its isomers, offering better resolution and retention than an amide column with a larger particle size (3 μm). In addition to the stationary phase, the composition of the mobile phase—particularly the organic solvent type and ammonia concentration—was critical for achieving good resolution. Acetonitrile showed superior separation capability for glucose and its isomers compared to methanol, and was thus selected as the organic solvent. Through optimization, we found that the ammonia concentration considerably influenced the separation, with the best separation and highest sensitivity achieved at an ammonia concentration of 0.05%. Thus, with a slow gradient elution, the mobile phase consisted of 0.05% aqueous ammonia and 0.05% ammonia in acetonitrile. Satisfactory separation of glucose and fructose was achieved under these optimized conditions.
Traditional sample preparation methods often involve multiple steps, such as adding ISs and extraction solvents, and vortexing, which limits throughput. In this study, we utilized a high-throughput protein precipitation method using a 96-well plate, allowing for rapid parallel preparation of multiple samples, improving efficiency and reducing costs. Methanol was used for protein precipitation in plasma samples, and the IS working solution was directly incorporated into the precipitant, simplifying the procedure while minimizing organic solvent use. The use of an isotopic IS considerably improved the method's durability. Additionally, the supernatant was diluted 25-fold with 40% acetonitrile in water after protein precipitation to minimize matrix effects.
3.2 Method validation
3.2.1 Selectivity and carryover.
The accuracy deviation range for MQC samples prepared from six different sources of normal blank plasma, one source of high-fat blank plasma, and one source of hemolyzed blank plasma was between −0.2% and 1.5%, with a maximum precision (%CV) of 0.9%, indicating that the method's selectivity was acceptable. No interference was observed between the analyte and the IS. The average accuracy deviation for LLOQ plasma and LLOQ surrogate matrix samples was within 20%. Fig. 1 presents representative MRM chromatograms of double blank surrogate matrix samples, QC0 samples containing a surrogate matrix, LLOQ samples prepared with a blank surrogate matrix containing both analyte and IS double blank plasma samples, and plasma samples collected 20 min after oral administration of the acarbose tablets. The retention times for glucose, glucose-[U-13C6], and fructose were 1.99, 1.99, and 1.73 min, respectively. At the retention times of the analyte and the IS, interference peaks were not observed in the surrogate matrix, and the IS did not interfere with the analyte. The carryover for the analyte was less than 20% of the LLOQ, and the carryover for the IS was less than 5%, confirming that the method's carryover was acceptable.
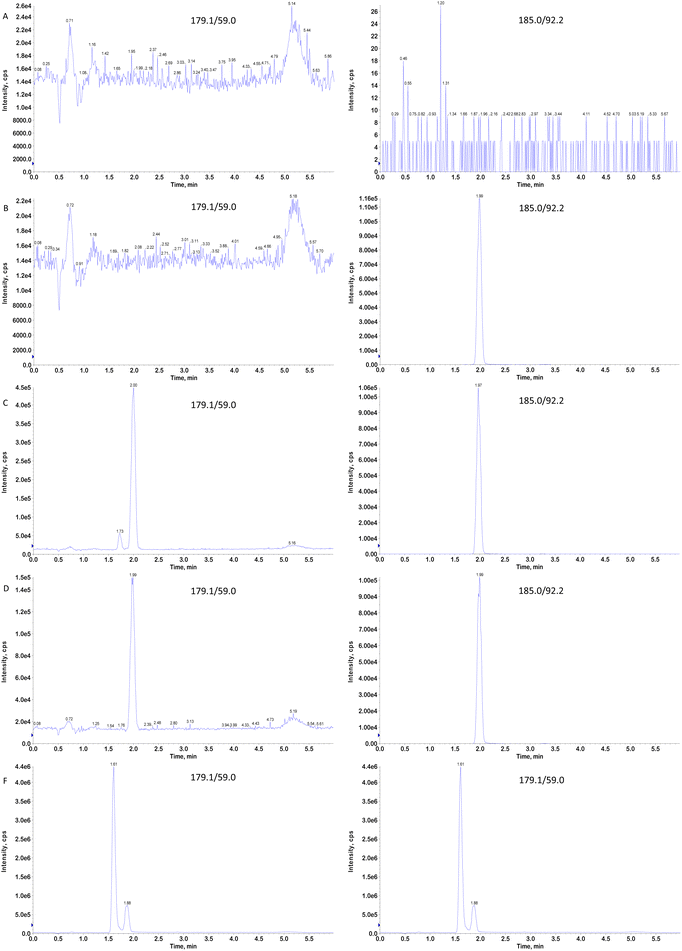 |
| Fig. 1 Mass chromatograms of (A) the blank sample, (B) the QC0 sample, (C) the LLOQ sample of glucose (2.00 mmol L−1), (D) a subject's sample at 6.10 mmol L−1 concentration and (F) samples containing both glucose and fructose. | |
3.2.2 Specificity.
Fig. 1(F) clearly shows a good separation between fructose and glucose. The average accuracy deviation for the LLOQ samples ranged from −2.5% to 6.5%, with a maximum precision (%CV) of 4.8%, demonstrating that fructose does not interfere with glucose quantification in this method.
3.2.3 Linearity and sensitivity.
Across all analytical batches, linearity was assessed using the calibration curves established during method validation, with a concentration range of 2.00 to 20.00 mmol L−1. All standard curves demonstrated excellent linearity, with correlation coefficients (r) greater than 0.9950. The average linear equation was y = 0.524877x + −0.0440105. The back-calculated concentrations for the standard curves had deviations ranging from −7.1 to 11.9%, with precisions (%CV) between 2.2 and 3.4%.
3.2.4 Precision and accuracy.
The accuracy and precision results for the QC samples are presented in Table 2. For both the surrogate matrix and actual matrix QC samples, the within-run and between-run accuracy deviations ranged from −3.8 to 7.5%, with a maximum precision (%CV) of 4.4% for both within-run and between-run measurements.
Table 2 Within-run and between-run accuracies and precisions
Analyte |
|
QC samples (mmol L−1) |
LLOQ (2.00) |
LQC (5.70) |
MQC (10.00) |
HQC (15.00) |
Within-run 1 (n = 6) |
Mean |
2.06 |
6.02 |
10.01 |
15.73 |
CV (%) |
3.9 |
1.8 |
0.8 |
1.0 |
Bias (%) |
1.0 |
1.1 |
1.0 |
1.0 |
Within-run 2 (n = 6) |
Mean |
2.02 |
5.65 |
9.78 |
14.78 |
CV (%) |
2.5 |
2.3 |
1.8 |
1.3 |
Bias (%) |
1.0 |
1.0 |
1.0 |
1.0 |
Within-run 3 (n = 6) |
Mean |
1.96 |
5.57 |
9.62 |
14.45 |
CV (%) |
1.0 |
0.9 |
1.4 |
0.9 |
Bias (%) |
−2.0 |
−2.3 |
−3.8 |
−3.7 |
Between-run (n = 18) |
Mean |
2.01 |
5.75 |
9.80 |
14.99 |
CV (%) |
2.4 |
4.2 |
2.0 |
4.4 |
Bias (%) |
1.0 |
2.9 |
4.9 |
7.5 |
3.2.5 Matrix effect.
The matrix effect was evaluated using LQC and HQC across six different normal blank matrix sources and one surrogate matrix source. The accuracy deviation for both LQC and HQC ranged from 1.0 to 1.4%, with maximum precisions (%CV) of 1.7 and 0.1%, respectively. The results are presented in Table 3. These results confirm that matrix effects do not considerably affect glucose quantification across different matrix types.
Table 3 Matrix effect results for glucose
Matrix |
LQC (5.70 mmol L−1) |
HQC (10.0 mmol L−1) |
Bias (%) |
CV (%) |
Bias (%) |
CV (%) |
Matrix 1 |
1.4 |
0.5 |
1.1 |
0.0 |
Matrix 2 |
1.0 |
0.5 |
1.0 |
0.1 |
Matrix 3 |
1.1 |
0.3 |
1.0 |
0.0 |
Matrix 4 |
1.1 |
0.7 |
1.0 |
0.0 |
Matrix 5 |
1.0 |
1.1 |
1.0 |
0.0 |
Matrix 6 |
1.0 |
1.7 |
1.0 |
0.1 |
Hemolysis |
1.0 |
0.6 |
1.0 |
0.0 |
Hyperlipemia |
1.0 |
0.7 |
1.0 |
0.0 |
3.2.6 Extraction recovery.
The mean peak areas of the LQC, MQC, and HQC samples were analyzed in both the surrogate and actual matrices. In the surrogate matrix, the extraction recoveries of glucose in LQC, MQC, and HQC were 110.2, 110.6, and 112.1%, respectively, with an overall %CV of 0.9%. The extraction recovery of the IS was 110.3%, with a %CV of 0.9%. Similarly, in the actual matrix (human plasma), the extraction recoveries of glucose in the MQC and HQC were 111.3 and 116.7%, with an overall %CV of 3.3%. The extraction recovery of the IS was 110.3%, with a %CV of 1.4% (Table 4).
Table 4 Recovery results for glucose and glucose-[U-13C6] IS (n = 3)
QC samples |
Surrogate matrix |
Actual matrix |
Surrogate matrix IS |
Actual matrix IS |
The endogenous glucose in the actual matrix was 5.70 mmol L−1, which was used as an LQC sample for the actual matrix. The extraction recovery rate calculation could not be performed.
|
LQC |
110.2 |
|
109.3 |
108.2 |
MQC |
110.6 |
111.3 |
110.5 |
110.5 |
HQC |
112.1 |
116.7 |
111.2 |
111.2 |
Mean |
111.0 |
114 |
110.3 |
110.0 |
CV (%) |
0.9 |
3.3 |
0.9 |
1.4 |
3.2.7 Dilution integrity.
The samples were evaluated following a five-fold dilution. The accuracy deviation ranged from 0.5 to 4.3%, and the precision (%CV) was 1.3%, which meets the acceptable criteria. Therefore, the method allows for a five-fold dilution.
3.2.8 Stability.
A stability test of glucose in actual matrix and surrogate matrix samples and solutions was performed under different conditions. These evaluations involved the short- and long-term stabilities of stock and working solutions kept at 25 ± 5 °C for 48 h and at −20 °C for 50 and 20 days, respectively, the stability of whole blood samples maintained in an ice bath for 2 h, the short-term stability of actual and surrogate matrix samples held at room temperature for 24 h, the long-term stability of actual matrix samples stored at −20 and −70 °C for 48 days, the stability through five freeze–thaw cycles for actual matrix samples at −20 and −80 °C, the stability of treated actual matrix and surrogate matrix samples placed in the autosampler (8 °C) for 105 h, and the reinjection reproducibility of prepared actual matrix and surrogate matrix samples placed in the autosampler (8 °C) for 95 h. Both accuracy and precision were required to be within 15%. All results fell within the acceptable range, as shown in Table 5.
Table 5 Stability study results for glucose under different conditions (n = 3)a
Storage conditions |
Matrix |
Concentration (mmol L−1) |
Bias (%) |
Precision (CV,%) |
NA: not applicable.
|
Whole blood, WI for 2 h |
Blood |
LQC 5.70 |
−0.4 |
NA |
HQC 15.0 |
1.8 |
NA |
Short-term, RT for 24 h |
Surrogate matrix |
LQC 5.70 |
0.8 |
0.9 |
HQC 15.0 |
1.1 |
1.4 |
Post-preparative in an autosampler, 8 °C for 105 h |
LQC 5.70 |
−2.3 |
0.5 |
HQC 15.0 |
−1.9 |
0.2 |
Reinjection reproducibility of prepared, 8 °C for 95 h |
LLOQ 2.00 |
−0.3 |
1.3 |
LQC 5.70 |
−2.5 |
0.5 |
MQC 10.0 |
−1.6 |
0.9 |
HQC 15.0 |
−1.6 |
1.1 |
Short-term, RT for 24 h |
Actual matrix |
LQC 5.70 |
4.4 |
0.5 |
HQC 15.0 |
5.2 |
0.4 |
Post-preparative in an autosampler, 8 °C for 105 h |
LQC 5.70 |
1.7 |
0.9 |
HQC 15.0 |
−0.4 |
0.6 |
Reinjection reproducibility of prepared, 8 °C for 95 h |
LLOQ 2.00 |
4.0 |
1.0 |
LQC 5.70 |
2.0 |
0.4 |
MQC 10.0 |
−0.3 |
0.6 |
HQC 15.0 |
−0.9 |
0.8 |
Five freeze–thaw cycles, −20 °C to RT |
LQC 5.70 |
3.6 |
0.5 |
HQC 15.0 |
−0.6 |
0.5 |
Five freeze–thaw cycles, −80 °C to RT |
LQC 5.70 |
2.6 |
0.9 |
HQC 15.0 |
0.1 |
1.0 |
Long-term, −20 °C for 48 days |
Actual matrix |
LQC 5.70 |
3.2 |
3.1 |
HQC 15.0 |
2.5 |
1.8 |
Long-term, −80 °C for 48 days |
LQC 5.70 |
1.8 |
1.6 |
HQC 15.0 |
−1.7 |
0.9 |
Short-term, RT for 48 h |
Stock standard solution |
800 |
−1.9 |
NA |
Long-term, −20 °C for 50 days |
800 |
−2.8 |
NA |
Short-term, RT for 48 h |
Working standard |
LLOQ 40.0 |
−0.6 |
NA |
ULOQ 400 |
−3.2 |
NA |
Long-term, −20 °C for 20 days |
LLOQ 40.0 |
−4.5 |
NA |
ULOQ 400 |
−4.9 |
NA |
3.2.9 ISR.
In this study, 110 samples were selected for re-analysis. The re-analyzed concentrations for all 110 samples were within ±20% of the original values, confirming the reproducibility of the method.
3.3 Pharmacodynamics study
Using PBS as a surrogate matrix, the optimized LC-MS/MS method was applied to measure glucose concentrations in human plasma to evaluate the bioequivalence of the test and reference formulations of acarbose tablets under fasting conditions. Time–concentration profiles for glucose were plotted, with time on the x-axis and plasma glucose concentration on the y-axis, for both formulations under fasting conditions (Fig. 2). The plasma glucose concentration–time profiles showed that the Cmax of plasma glucose occurred between 25 and 35 min after sucrose and reverted to the baseline level approximately 2 h later. The glucose curve during the 2–4 h period after sucrose consumption exhibited a glucose level that was lower than that at 0 h. The incorporation of acarbose reduced the time taken to reach the maximum concentration of plasma glucose between 15 and 25 min and decreased the Cmax of plasma glucose by approximately 17.9%. Unlike the sucrose load curve, the glucose level after acarbose administration remained relatively stable, fluctuating slightly around the baseline between 2 and 4 h post-dosing. The administration of acarbose also resulted in a shortened time taken to reach the maximum plasma glucose concentration and a decrease in the Cmax of plasma glucose. The pharmacodynamic parameters were calculated for both formulations utilizing DAS 2.0 software, and the summarized results are presented in Table 6. The 90% confidence intervals (CI) for the geometric mean ratios of these parameters were calculated. For the test and reference formulations of acarbose under fasting conditions, the 90% CI of the geometric mean ratios for Cmax and AUEC0–2h were 72.8–109.8% and 97.2–146.5%, respectively.
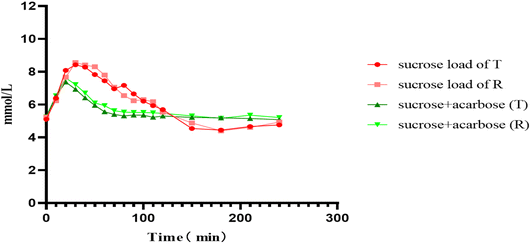 |
| Fig. 2 Mean plasma glucose concentration–time profiles after administration. | |
Table 6 Pharmacokinetic test and reference formulation data for healthy volunteers under fasting conditions (mean ± SD, n = 16)a
Parameters |
Geometric means |
Bioavailability |
T |
R |
Ratio% (T/R) |
90% CI |
C
max, maximum plasma glucose concentration after co-administration of sucrose and acarbose; Cmax,co, maximum plasma glucose concentration with the deduction of glucose concentration at 0 h; Cmax,f, maximum plasma glucose concentration after administration of sucrose; ΔCmax = Cmax,f − Cmax,co; AUC0–2h, the area under the plasma glucose concentration–time curve 0–2 h after co-administration of sucrose and acarbose; AUC0–2h,co, area under the concentration–time curve 0 to 2 h after co-administration of sucrose and acarbose with deduction of the baseline area under the concentration–time curve; AUC0–2h,f area under the plasma glucose concentration–time curve 0–2 h after administration of sucrose; AUEC0–2h = AUC0–2h,f − AUC0–2h,co.
|
C
max,co |
7.62 ± 0.95 |
7.79 ± 0.80 |
97.82 |
91.2–104.5 |
C
max
|
2.33 ± 0.85 |
2.53 ± 0.82 |
92.09 |
72.8–109.8 |
C
max,f |
9.28 ± 1.32 |
9.33 ± 1.53 |
99.46 |
95.1–104.5 |
ΔCmax |
1.66 ± 1.09 |
1.54 ± 1.64 |
107.8 |
97.2–146.5 |
AUC0–2h,co |
712.71 ± 64.12 |
736.17 ± 41.65 |
96.81 |
93.5–99.7 |
AUC0–2h |
77.46 ± 56.00 |
105.20 ± 48.14 |
73.63 |
60.3–86.92 |
AUC0–2h,f |
859.85 ± 82.72 |
863.42 ± 136.56 |
99.55 |
96.1–134.8 |
AUEC0–2h |
147.14 ± 71.07 |
127.41 ± 127.85 |
115.49 |
97.2–146.5 |
4 Conclusions
This study established a method for measuring glucose concentrations in human plasma using a surrogate matrix approach combined with UPLC-MS/MS technology. The method underwent complete validation following M10 guidelines,17 with the linearity, selectivity, matrix effect, recovery, stability, dilution reliability, precision, and accuracy all meeting acceptable standards. This study provides valuable reference data for bioequivalence studies of acarbose-related formulations and is suitable for pharmacodynamics studies of acarbose, offering a solid foundation for consistency evaluations of acarbose formulations. According to the FDA guidelines for the bioequivalence evaluation of acarbose,5 this study compared the BE between the test and reference drug. The 90% confidence interval for baseline-corrected pharmacodynamic endpoints Cmax and AUEC0–2h are not between 80 and 125%. The test and reference formulations were non-bioequivalent, which may be related to the preparation method for the two formulations.
Data availability
The data that support the findings of the study are available on request from the corresponding author.
Author contributions
Xunjie Zhang: conceptualization, resources, writing – review & editing. Ke Li and Ruiqing Xian: formal analysis, methodology, validation. Pengfei You: data curation, formal analysis. Chaochao Liang: writing – original draft. Feng Shi: data curation, investigation. Baojian Hang: conceptualization, software. Liping Gong: funding acquisition, project administration, supervision.
Conflicts of interest
The authors declare no conflicts of interest.
Acknowledgements
The authors thank all the volunteers, investigators, and medical, nursing, and laboratory staff who participated in this study. We also thank the Xin Hua Hospital of Zhejiang Province for their cooperation. We thank LetPub (https://www.letpub.com.cn) for its linguistic assistance during the preparation of this manuscript.
References
- Z. Chen, J. Xu, C. Ge, L. Zhang and W. Jiang, Effect of free medication on the follow-up effect of patients with type 2 diabetes mellitus in Tianchang City: an empirical study based on propensity score matching(PSM), J. Nanjing Med. Univ, 2024, 4, 399–405 Search PubMed.
- Y. Yang and X. Yu, Status of metformin in the drug treatment for type 2 diabetes mellitus based on the update of guideline, Chin. J. Pract. Intern. Med., 2022, 42, 884–888 CAS.
- L. Que, Z. Qian, X. Xiang, Y. Ding, K. Huang, Y. Bai, H. Zhao and Q. He, Evaluation of Acarbose Bioequivalence in Healthy Chinese Populations Using Novel Pharmacodynamic End Points, Clin. Pharmacol. Drug Dev., 2024, 13, 233–239 CrossRef CAS PubMed.
- L. Que, K. Huang, Y. Ding, N. Chu, J. Yang, Z. Qian and Q. He, Acarbose bioequivalence: Exploration of eligible protocol design, J. Clin. Pharm. Ther., 2021, 46, 492–503 CrossRef CAS PubMed.
-
U.S. Food and Drug Administration. Draft Guidance on Acarbose. May 2022 Search PubMed.
- X. Dai, Y. Qi, M. Yang, B. Xu and X. Fang, determination methods of glucose in serum and its development, Chem. Anal. Meterage, 2008, 17, 78–80 CAS.
- Y. Chen, F. Guo, X. Wang, L. Liu, C. Yang, Y. Xiong and H. Zhang, Evaluation of the Bioequivalence of Acarbose in Healthy Chinese People, Clin. Pharmacol. Drug Dev., 2021, 10, 1225–1230 CrossRef CAS PubMed.
- C. Quan, L. Wu, X. Dai, B. Xu, R. Xu and M. Shao, CCQM –K11.1 international comparison on the determination of serum glucose, Chem. Anal. Meterage, 2006, 15, 50–53 CAS.
- Y. Chen, Q. Liu, S. Yong and T. Lee, High accuracy analysis of glucose in human serum by isotope dilution liquid chromatography-tandem mass spectrometry, Clin. Chim. Acta, 2012, 413, 808–813 CrossRef CAS PubMed.
- R. K. Matsunami, K. Angelides and D. A. Engler, Development and Validation of a Rapid (13)C6-Glucose Isotope Dilution UPLC-MRM Mass Spectrometry Method for Use in Determining System Accuracy and Performance of Blood Glucose Monitoring Devices, J. Diabetes Sci. Technol., 2015, 9, 1051–1060 CrossRef CAS PubMed.
- Z. Ding, C. Quan, S. Jin, H. Li, X. Dai and B. Xu, Determination of serum glucoseDby gas chromatography-mass spectrometry, J. Beijing Univ. Chem. Technol., 2010, 37, 109–112 CAS.
- L. Yu, C. Wen, X. Li, S. Fang, L. Yang, T. Wang and K. Hu, Simultaneous quantification of endogenous and exogenous plasma glucose by isotope dilution LC-MS/MS with indirect MRM of the derivative tag, Anal. Bioanal. Chem., 2018, 410, 2011–2018 CrossRef CAS PubMed.
- E. C. Wan and J. Z. Yu, Determination of sugar compounds in atmospheric aerosols by liquid chromatography combined with positive electrospray ionization mass spectrometry, J. Chromatogr. A, 2006, 1107, 175–181 CrossRef CAS PubMed.
- M. Huang, Q. Zhang, H. Xu, M. Wang and W. Zhou, Bioequivalence Evaluation of Acarbose Tablet in Healthy Chinese Volunteers with Pharmacodynamic Endpoints, Chin. Pharm. J., 2019, 54, 1323–1327 Search PubMed.
- Y. Shen, C. Quan and K. Ma, Accurate Determination of Serum Glucose by Liquid Chromatography-Isotope Dilution Mass Spectrometry, J. Chin. Mass Spectrom. Soc., 2011, 32, 211–215+228 CAS.
- Y. Chen, F. Guo, X. Wang, L. Liu, C. Yang, Y. Xiong and H. Zhang, Evaluation of the Bioequivalence of Acarbose in Healthy Chinese People, Clin. Pharmacol. Drug Dev., 2021, 10, 1225–1230 CrossRef CAS PubMed.
-
International Council for Harmonisation of Technical Requirements for Pharmaceuticals for Human Use (ICH). M10 Bioanalytical Method Validation and Study Sample Analysis Draft Version. 26 February 2019 Search PubMed.
|
This journal is © The Royal Society of Chemistry 2025 |
Click here to see how this site uses Cookies. View our privacy policy here.