DOI:
10.1039/D3YA00482A
(Paper)
Energy Adv., 2024,
3, 614-624
In situ surface monitoring of energy materials during processing: impact of defect disorder on surface versus bulk semiconducting properties of photocatalytic hematite (Fe2O3)
Received
29th September 2023
, Accepted 28th January 2024
First published on 31st January 2024
Abstract
Rational design of surface properties of oxide semiconductors for energy conversion requires in situ surface characterization. This work considers in situ monitoring of surface semiconducting properties of hematite (Fe2O3) during isothermal oxidation and reduction at 1140 K, and the related charge transfer at the Fe2O3–O2 interface using the measurements of work function (WF) changes during successive oxidation and reduction experiments under two extreme oxygen activities of 44 kPa and 220 Pa. The obtained results indicate that prolonged annealing of Fe2O3 leads to the formation of an n-type surface layer on top of the bulk phase of hematite that exhibits intrinsic semiconducting properties. The derived theoretical model shows that the reactivity of Fe2O3 with oxygen involves two parallel processes: (i) fast bulk diffusion of defects associated with penetration of oxygen activity into the oxide lattice, and (ii) slow surface segregation of defects, derived from the bulk phase, and resulting in the formation of a quasi-isolated surface structure (QISS) that plays a critical role in the performance of energy materials. The obtained results led to derivation of theoretical models that explain the role of the QISS in the performance of energy materials.
1. Introduction
Background
The performance of materials for electrochemical energy conversion systems, such as solar cells for water oxidation, is profoundly affected by surface defect disorder.1 Therefore, the development of novel energy materials requires surface defect engineering (SDE) that allows imposition of an optimal surface defect disorder required for desired performance. The main hurdle in addressing this objective is that the science of defect chemistry is derived at elevated temperatures, corresponding to defect equilibria,2–4 while the performance of energy conversion systems, such as photocatalysts for solar water splitting, takes place at room temperature. Therefore, the development of SDE for energy materials requires expansion of defect chemistry into surface defect chemistry in order to assess defect equilibria within the surface layer.
The present work uses the hematite phase (Fe2O3) as a template for photosensitive oxide semiconductors (POSs) for in situ surface monitoring during oxidation and reduction at elevated temperatures. Here we show that the reactivity of POSs with oxygen should be considered in terms of two parallel processes: (i) oxygen activity penetration from the surface to the bulk phase, and (ii) surface segregation of defects transported in the opposite direction. The latter process results in building-up of a quasi-isolated surface structure (QISS) that plays a critical role in the charge transfer associated with energy conversion or any other surface activated process.
Defect chemistry
The influence of structural defects on the reactivity of ionic solids resulted in the development of defect chemistry that is limited to the bulk phase.2–4 The progress in defect chemistry of metal oxides made so far allows assessment of defect disorder, and the related semiconducting properties, within a wide range of oxygen activities and temperatures. Recent studies, however, indicate that the reactivity of solids is determined by defect disorder of the surface layer.1,5–7 These studies signal the need for expansion of defect chemistry into the surface layer and the development of SDE for processing of novel energy materials with desired properties required for specific applications. Such studies, however, require a tool that has the capacity to assess surface defect disorder at elevated temperatures corresponding to defect equilibria. Efforts to build such a tool, led to the development of the high-temperature electron probe (HTEP), that is based on work function (WF) measurements.7–15
The HTEP has been used in studies of surface properties of NiO,8 FeO,9,10 YSZ,11,12 CoO,13 BaTiO314 and TiO2.15 These studies indicate that progress in the development of SDE requires recognition that defect disorder is well defined only in gas/solid equilibrium, when the surface layer is in equilibrium with both the gas phase and the bulk phase.7,15 The hematite phase, Fe2O3, is not an exception.
Defect chemistry from surface perspective
Studies of surface properties of solids, such as metal oxides, are commonly preceded by meticulous surface cleaning leading to removal of surface contaminations.16 However, the surface cleaning procedures, commonly performed in vacuo and applied before surface characterizations, result in changes of the intrinsic defect disorder within the surface layer. Therefore, the key advantage of surface studies in gas/solid equilibrium is the opportunity to consider surface properties in thermodynamic terms when defect-related properties of metal oxides are determined by the temperature and oxygen activity of the surrounding gas phase. So far, little is known about the surface properties of metal oxides in gas/solid equilibrium when defect disorder is governed by thermodynamic terms.
Rationale of high-temperature studies
Defect chemistry considers defect-related properties at elevated temperatures corresponding to gas/solid equilibrium when defect disorder is governed by thermodynamic terms and is free of kinetic terms. While most energy conversion devices operate around room temperature when the kinetic terms assume substantial values, studies at elevated temperatures allow an understanding of the impact of thermodynamic terms on changes of properties in performance conditions. So far, little is known about the impact of thermodynamic terms on surface properties. In other words, modifications of defect-related surface properties in a controlled manner require elevated temperatures that are indispensable for SDE. Therefore, the objective of this work is to assess the impact of thermodynamics on the surface properties of hematite as a template for nonstoichiometric compounds.
Applied aspects
Hematite is one of the most abundant oxides, which has been identified as a promising photocatalytic material17–21 owing to its relatively narrow band gap of 2.1 eV. While photocatalytic reactivity of oxide semiconductors is profoundly affected by light absorption, which is related to the width of bandgap, recent studies indicate that it is mostly determined by surface population of defects acting as active sites.7,15,22 The hematite phase exhibits a very low non-stoichiometry and intrinsic semiconducting properties.23–28 This, consequently, suggests that the bulk phase of hematite is almost stoichiometric.23 So far, however, little is known about the surface defect disorder of Fe2O3 and its impact on reactivity. While the reactivity-related applied aspects of solids are determined by surface defect disorder, the related reports on properties of hematite are limited to the bulk phase. Here we show that the HTEP can offer a quantitative assessment of surface semiconducting properties and provide some hints regarding its local defect disorder.
Significance of the emerging area of surface defect chemistry
As postulated earlier, reactivity of ionic solids, such as metal oxides, is determined by surface properties, particularly defect disorder and the related semiconducting properties.7 However, most of surface sensitive tools operate at room temperature and in vacuum.16 On the other hand, the rational design of energy conversion systems requires an in situ surface studies in the conditions of the gas phase and temperature that are relevant to specific applications.29 This study, which represents a pioneering approach for in situ monitoring of surface semiconducting properties of hematite at elevated temperatures (corresponding to defect equilibria) opens new areas of high-temperature surface science and defect engineering. The latter, which results in processing of new energy conversion systems based on crystalline solids, is expected to result in enhanced energy security and reduction of global warming.
Objectives
This work aims to understand the effect of prolonged annealing of the hematite phase on surface defect disorder and the resulting reactivity with oxygen. The WF data will be considered in terms of surface properties reflecting changes in both (i) the position of Fermi level at the surface, and (ii) a defect-related parameter (exponent of oxygen activity) that can be compared to the analogous parameter derived for the bulk phase.7 The experimental part of this study is preceded by a definition of terms and concepts, and the discussion of the physical meaning of WF changes at elevated temperatures.
2. Defect chemistry of hematite
Basic concept of defect chemistry
Defect disorder in oxides is commonly studied by monitoring gas/solid equilibration under controlled oxygen activity.2–4 Typically, the equilibration process in the metal oxide/oxygen system can be described as propagation of new oxygen activity within the oxide lattice upon altering the oxygen activity in the surrounding gas phase. The progress of equilibration is commonly monitored by measurements of bulk defect-related properties, such as electrical conductivity, and these may be used to determine diffusion data.2–4,24 The models usually employed assume that the gas/solid equilibration is rate-controlled by chemical diffusion within the bulk phase while the surface reaction is much faster.2–4,30 However, verification of this assumption requires performing gas/solid equilibration from the surface perspective. Hence, the purpose of this work is to address this objective by analyzing the WF changes of Fe2O3 during oxidation and reduction at elevated temperatures.
Defect chemistry of hematite
Studies of hematite's bulk defect disorder indicate that its predominant ionic defects are oxygen vacancies.23–28 Chang and Wagner23 reported that the concentration of intrinsic ionic defects in Fe2O3 is much lower than that of electronic defects and that its semiconducting properties in the range 1223–1695 K are intrinsic. This conclusion was supported by both Gleitzer et al.,27 showing that the electrical properties of α-Fe2O3 are largely independent of oxygen activity, and Wang et al.28 who observed that the electrical properties of hematite at moderate temperatures (523–673 K) are largely p(O2) independent. The ab initio calculations of Lee and Han31 and Shousha et al.32 show that both iron interstitials and iron vacancies are also present in pure Fe2O3. Kraushofer et al.33 reported extensive surface studies of Fe2O3 (1102) single crystal using low energy electron diffraction (LEED), X-ray photoelectron spectroscopy (XPS), ultraviolet photoelectron spectroscopy (UPS), scanning tunneling microscopy (STM), and atomic force microscopy (AFM), during oxidation and reduction. These results collectively highlight the complications inherent in the characterization of surface defect-related properties that are typically examined in a controlled environment of ultra-high vacuum and ambient temperature. Such conditions are incommensurate with the actual surroundings of Fe2O3 employed for practical applications. Studies of surface semiconducting properties of hematite by Adam et al.34 dealt with the effect of prolonged annealing on the contact potential difference (CPD) between the surface of hematite and platinum covered with a thin layer of PtO2. Since surface monitoring of the charge transfer at the Fe2O3–O2 interface is expected to offer an insight into the role of surface defect disorder in reactivity, this work aims to convert the CPD data reported before into WF changes of Fe2O3.
High-temperature electron probe
The reactivity of metal oxides is determined by their ability to transfer charge across the gas/solid interface. The related key quantity has been considered in terms of the Fermi level, EF, that is commonly determined by the measurements of thermoelectric power. However, the EF determined in this way corresponds to the bulk phase.35 Because the reactivity of solids depends on surface defect disorder,1,5,7 the ability to transfer charge should be considered in terms of the surface position of the Fermi level. The HTEP may be used to determine the effect of oxygen activity on the change of surface Fermi level position between two gas–solid equilibrium states.7 Consequently, the HTEP is uniquely positioned for direct diagnosis of surface reactivity in gas–solid equilibrium in terms of the WF changes that can be considered as an unequivocal surface property that is well reproducible and independent of surface cleaning procedures, if any.
3. Reactivity mechanism
The reactivity of metal oxides with oxygen may be considered in terms of oxygen chemisorption, oxygen incorporation into the surface layer and the transport through this layer, and oxygen transport within the bulk phase.7,15 Progression of oxygen chemisorption is determined by the charge transfer between the physically adsorbed oxygen species and the surface layer, leading to the formation of differently ionized oxygen species. The related charge neutrality condition requires that the charge of chemisorbed (molecular and atomic) oxygen species is compensated by the space charge within the boundary layer. At elevated temperatures, chemisorbed oxygen species are quickly incorporated into the surface layer in the form of doubly ionized oxygen ions,7,15 then these species migrate through the electric fields within the space charge layer. It has been shown that these fields have a substantial impact on surface segregation of defects, which is rate controlled by diffusion.36 So far, little is known about the impact of the segregation-induced electric field on reactivity and the charge transfer at the gas/solid interface. The challenge in understanding the mechanism of this process requires in situ surface monitoring that allows assessment of the local equilibration kinetics within the surface layer. Such monitoring can be performed with the HTEP, thanks to its unique ability to operate at elevated temperatures and in the gas phase of controlled oxygen activity.
4. Definition of terms and concepts
Effect of oxygen activity on semiconducting properties
Ionization of ionic defects results in the formation of quasi-free electrons. Their concentration in equilibrium can be derived from the mass-action law within a framework of the theoretical defect disorder model:3 | 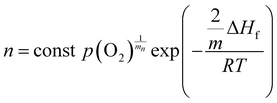 | (1) |
where 1/mn is the parameter related to defect disorder and ΔHf is the enthalpy related to the formation of defects. Verification of selected models involves the measurements of defect-related properties, such as electrical conductivity (σ), which is a bulk property, or WF (Φ), which is a defect-related surface property. Typically, examination of the isothermal effect of oxygen activity on these properties may be expressed by eqn (2) and (3), respectively:7 | 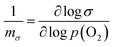 | (2) |
| 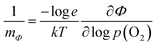 | (3) |
where e is the Euler number, p(O2) is oxygen activity, and the parameters 1/mσ and 1/mΦ correspond to defect disorder in the bulk phase and at the surface, respectively. The WF of a semiconductor is defined in Fig. 1 in terms of WF components including, the external WF, χ, the WF related to surface charge, ΦS, and the internal WF component related to the Fermi level, Φin.7
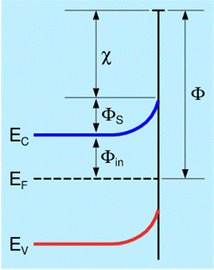 |
| Fig. 1 Definition of work function for an n-type semiconductor. | |
As seen in Fig. 1, the change in WF reflects the change in surface position of the Fermi level, EF, when the surface crystalline structure remains unchanged and the component χ is constant, and the change of the chemisorption related WF component ΦS can be ignored. The latter assumption is usually correct at elevated temperatures. Then, we have:
where Δ
Φ denotes WF changes during oxidation or reduction. The HTEP instrument measures the changes in contact potential difference (CPD) between the studied specimen and the reference electrode, which in this case is made of platinum covered with a thin layer of PtO
2.
14 Therefore, the specimen's WF changes can be obtained from the following expression:
where
Φref denotes WF of the reference specimen,
e is an elementary charge, and CPD is the contact potential difference. The component Δ
Φref can be determined from
eqn (3) assuming that the parameter
mΦ of the surface layer of PtO
2 is equal to 4.
14 Possible presence of the surface charge during oxidation and reduction experiments at elevated temperatures affects the WF component
ΦS, which at these temperatures is related only weakly to oxygen chemisorption that results in a decrease of surface energy. At the same time, surface segregation enriches the surface layer in selected lattice elements derived from the bulk phase. On the other hand, the potential change of the external WF component, χ, signals formation of the surface structure on top of the bulk phase.
Surface cleaning versus heating to equilibrium
Commonly applied surface cleaning procedures include exposure to vacuum or ion bombardment. While these procedures are critical for removal of adsorbed impurities,16 their key disadvantage is imposition of a surface defect disorder that is affected by a range of effects, such as (i) time exposure to vacuum, (ii) oxygen activity of the applied vacuum, and (iii) the time and energy of species used for bombardment that are not well-defined. Therefore, the results of defect-related surface sensitive tools, such as scanning tunnelling microscopy (STM), are reflective of surface properties that are profoundly affected by the applied cleaning procedures rather than intrinsic surface properties. An alternative type of surface processing of oxides is heating to the level of gas/solid equilibrium. Then the surface layer is well-defined by the gas/solid equilibrium. The advantage of this type of procedure is an outstanding reproducibility of surface properties that are well-defined by conditions of the equilibrium. Consequently, this kind of surface characterization results in unequivocal surface properties. However, this experimental approach requires a surface sensitive tool that allows surface characterization at elevated temperatures corresponding to gas/solid equilibrium.
Surface sensitive tool operating at elevated temperatures corresponding to defect equilibria
Here we report the use of the HTEP in surface monitoring of Fe2O3 at 1140 K and consider charge transfer at the Fe2O3/O2 interface during establishment of the gas/solid equilibrium. This experimental approach aims to understand the gas/solid equilibration from surface perspective.
5. Aim
Gas/solid equilibration from surface perspective
While it has been commonly assumed that the gas/solid equilibration of the metal oxide/oxygen system is rate determined by bulk diffusion and the surface reaction is fast,2–4,30 the use of WF measurements in surface monitoring of the charge transfer during oxidation of metal oxides indicates that the equilibration is rate controlled by surface segregation of defects derived from the bulk phase.7,15 The objective of this work is to examine the gas/solid equilibration for the Fe2O3–O2 system using the HTEP.
Effect of prolonged annealing on surface properties
In this paper we use the CPD data of the Fe2O3–PtO2 system reported earlier34 to determine WF changes of the Fe2O3 phase using the defect-related parameter of the surface layer of PtO2, 1/mΦ [eqn (3)].14 The previous work was focused on the characterization of the effect of surface mechanical treatment, such as grinding and polishing, in terms of the CPD data of the studied system. It has been shown that irreversible surface defects, such as grooves, have a profound effect on surface properties. The present work aims to perform a quantitative analysis of surface semiconducting properties of Fe2O3 and the related disorder of point defects, which are thermodynamically reversible. The analysis is focused on properties of the surface layer that is free of grooves as they were removed during the initial stage of annealing. Here we show that prolonged annealing of Fe2O3 leads to the formation of a segregation-induced n-type surface layer on top of the intrinsic bulk phase.
Thermodynamic framework
This work aims to assess surface properties in terms of the thermodynamic framework when the surface layer is equilibrated with both the gas phase and the bulk phase. This is a pioneering approach to in situ surface monitoring because it investigates the influence of thermodynamics on surface properties during prolonged annealing.
Adsorption versus surface segregation
Surface characterization is commonly preceded by surface contaminations caused by adsorption of species derived from the gas phase.16 Surface segregation of species derived from the bulk phase is a phenomenon that is governed by the same law. So far, however, little is known about the effect of segregation on surface properties due to the lack of a tool that allows in situ surface monitoring during segregation-induced changes in surface properties. Here we show that the HTEP instrument is uniquely positioned to determine the effect of segregation-induced surface enrichment.
6. Experimental
Specimen
The polycrystalline specimen of Fe2O3 was supplied by Merck. Powder of average grain size of approximately 50 μm was initially pressed into slabs (1 mm × 8 mm × 8 mm), then sintered at 1673 K in air for 10 h, and finally polished with 0.1 μm diamond powder. SEM images of the polished specimens before annealing have been reported elsewhere.34
Instrument
The description of the HTEP instrument has been provided before.7 The studied specimen is placed on the lower electrode and forms a dynamic capacitor with the upper reference Pt electrode attached to the vibration system.
Procedure
Desired oxygen activity in the reaction chamber was imposed by the argon–oxygen mixture of controlled composition flowing through the reactor. Isothermal rapid changes of oxygen activity result in a CPD change that assumes stable values within 10–20 min. A zirconia oxygen sensor was employed to determine the ambient oxygen activity in the gas phase. The raw CPD results for the Fe2O3–PtO2 system determined during annealing at 1140 K in the gas phase of controlled oxygen activity have been initially reported elsewhere.34Eqn (5) was used to convert the CPD changes into WF changes of hematite during the prolonged annealing and the associated experiments of oxidation and reduction at 1140 K.
Experimental testing and characterization
The testing procedure applied in this work involves the following characterizations:
(1) The determination of the effect of oxygen activity on changes in the surface position of the Fermi level, defined by eqn (4), which is considered as a collective surface property.
(2) The determination of the effect of prolonged annealing on defect-related quantities for the bulk phase, 1/mσ, and the surface layer, 1/mΦ, which are considered as local properties associated with the predominant type of defects.
7. Results
Earlier work
The raw CPD results, corresponding to prolonged annealing of the Fe2O3–PtO2 system at 1140 K, including both oxidation and reduction experiments, were obtained earlier.34 These studies sought to understand the effects of polishing of the hematite specimen on its surface properties during prolonged annealing. It has been shown that the surface reactivity with oxygen involves two steps: a preliminary step, lasting 30 h, associated with removal of microscopic grooves left after polishing, and a subsequent step of equilibration with the gas phase for the surface free of grooves. These studies indicate that the reactivity mechanisms for these two types of surfaces are different.
Present work
The present work evaluates the reactivity mechanism at the Fe2O3–O2 interface and the related charge transfer for the specimen with an annealed surface that is free of grooves. The objective is to assess the effect of prolonged annealing of the hematite specimen on the WF changes at 1140 K and the associated changes of both the surface Fermi level and the defect-related parameter 1/mΦ at t > 30 h. This, consequently, requires conversion of the previously reported CPD data34 into WF changes of the hematite surface and analysis of the results.
Present results
The obtained WF changes induced by isothermal oxidation and reduction experiments performed during the prolonged annealing at 1140 K are represented in Fig. 2(a).
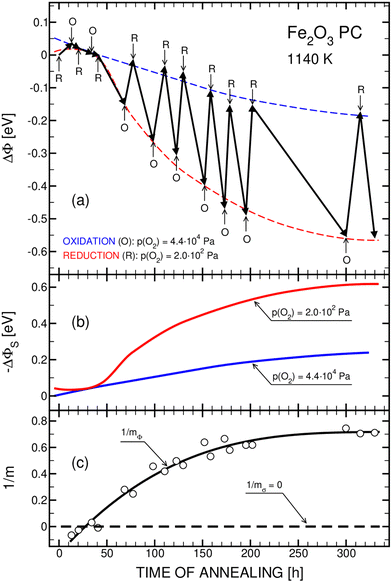 |
| Fig. 2 Surface properties of polycrystalline specimen of the hematite phase during prolonged annealing at 1140 K, including (a) the excursions in WF during successive oxidation (O) and reduction (R) experiments, (b) variations in the WF component ΦS in both oxidizing and reducing conditions, and (c) changes in the defect-related parameter for the surface layer 1/mΦ determined experimentally in the present work, and the defect-related parameter, 1/mσ, for the bulk phase corresponding to the intrinsic defect disorder.23,27 | |
As seen in Fig. 2(a), long annealing results in the following character of the WF changes (dashed lines) with time, t:
(1) For t < 30 h, oxidation and reduction results in insignificant WF changes that have been considered before.34
(2) At around 30 h, oxidation and reduction practically do not result in WF changes.
(3) For t > 30 h oxidation and reduction lead to WF decrease in both cases, although the change is more pronounced for reduction runs.
The WF changes within oxidation and reduction experiments are shown in Fig. 2(a) as thick arrows. The related data were assumed to be stable when they did not change substantially within 10–20 min.34 Each arrow represents a single oxidation or reduction run, starting with a rapid change of oxygen activity in the gas phase. The arrow's head-points represent the final “stable” values at the end of an individual run. It is easy to notice that those “stable” values, interpolated with blue and red dashed lines in Fig. 2(a), are not really stable but exhibit a slow drift. Only after over 300 h annealing the WF changes assumes constant values, indicating a state of thermodynamic equilibrium. The WF changes shown in Fig. 2(a), are further represented in terms of (i) the WF components involving both the surface charge, ΔΦS, and the external WF changes, Δχ, and (ii) the defect-related surface parameter, 1/mΦ, in Fig. 2(b) and 2(c), respectively.
8. Discussion
The obtained WF data that have been determined in gas/solid equilibrium can be considered as unequivocal intrinsic surface properties that are not affected by surface processing procedures, such as cleaning. These results are considered in terms of (i) surface equilibration mechanism, (ii) surface defect disorder, and (iii) surface semiconducting properties.
Influence of temperature on diffusion rate
The effect of temperature on diffusion can be described according to the following expression:6 | 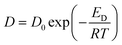 | (6) |
where: | 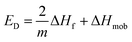 | (7) |
where ΔHf and ΔHmob are enthalpy terms related to the formation and the mobility of defects, D0 involves entropy terms and other parameters, such as frequency of jumps, and m is a defect disorder-related quantity. The self-diffusion coefficient, D, is related to the chemical diffusion coefficient, Dchem, according to the following expression: | 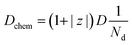 | (8) |
where z is the valency of diffusing defect species, and Nd is the concentration of the defect species. Eqn (6) leads to the conclusion that at lower temperatures, when defect disorder is quenched, diffusion is determined by defect's mobility. However, at higher temperatures, when changes in oxygen activity result in the formation/removal of defects and their transport, the effect of temperature on diffusion should be considered in terms of both enthalpy terms mentioned above. Eqn (8) defines the chemical diffusion coefficient that characterizes the transport of defects in concentration gradients.
Surface equilibration mechanism
Stationary versus equilibrium states.
The arrows representing the WF changes during oxidation and reduction experiments (Fig. 2(a)), indicate that the final WF data is considered as stable. However, the observed drift of the WF changes representing the effect of annealing on surface properties, demonstrates that the “stable” WF data at the end of a specific run represent a stationary state, rather than a true equilibrium. The prolonged drift of WF changes suggests the progression of a slow surface reaction that is associated with segregation of defects, as it has been observed during prolonged annealing of TiO2.15 The full gas/solid equilibrium in the Fe2O3–O2 system is established after 300 h at 1140 K.
Equilibration kinetics.
Imposing of a new oxygen activity in the reaction chamber at 1140 K results in a rapid change of surface potential within minutes, which is followed by a slow drift.34 The effect shown in Fig. 2, which reflects surface reaction, is in apparent disagreement with the present understanding of the reactivity of metal oxides assuming that surface reactions are typically rapid and not rate-controlling.2–4,30 The most feasible explanation of this effect is the formation of the segregation induced QISS.7,15
The key question here is: what is the reason for the exceptionally long time required for surface equilibration of ionic solids, such as hematite? The answer to this question requires recognition that equilibration of metal oxides during oxidation and reduction involves two parallel processes (Fig. 3):
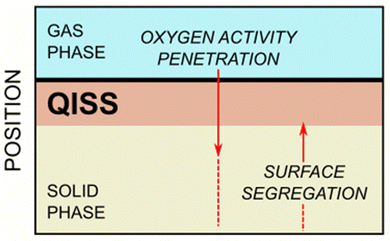 |
| Fig. 3 Schematic representation of transport processes during gas/solid equilibration of ionic solids. | |
(1) Penetration of oxygen activity from the gas/solid interface towards the bulk phase, and
(2) Segregation of lattice species derived from the bulk phase and migrating to the surface.
Effect of segregation on gas/solid equilibration.
The reported studies agree that the bulk phase of hematite is an intrinsic ionic conductor, and that its predominant defects are oxygen vacancies and iron vacancies at comparable concentrations.23–28 The results obtained in this work, which show that prolonged annealing results in WF decrease in both oxidizing and reducing conditions (Fig. 2(a) and (b)), indicate that the surface layer during the annealing process is enriched with donor-type defects, such as oxygen vacancies and iron interstitials. Moreover, the observed evolution of the parameter 1/mΦ from zero at t = 30 h, to 0.7 after 300 h of annealing at 1140 K (Fig. 2(c)) indicates that the defects within the surface layer after the annealing cannot be considered in terms of the ideal defect model. It is reasonable to expect that the increased concentration of defects within the surface layer results in increased interactions between defects, leading to the formation of complex structural lateral features that can be considered as QISS. Consequently, surface segregation of donor-type defects in Fe2O3, such as oxygen vacancies or iron interstitials, instigates a build-up of a surface layer that exhibits n-type semiconducting properties on top of the intrinsically conducting bulk phase of hematite. The mechanism of segregation is schematically represented in Fig. 4, showing the transport of iron ions from the lattice sites in the bulk phase to interstitial sites at the surface (Fig. 4(a)), and diffusion of oxygen vacancies derived from the bulk phase to the surface via the substitutional mechanism (Fig. 4(b)).
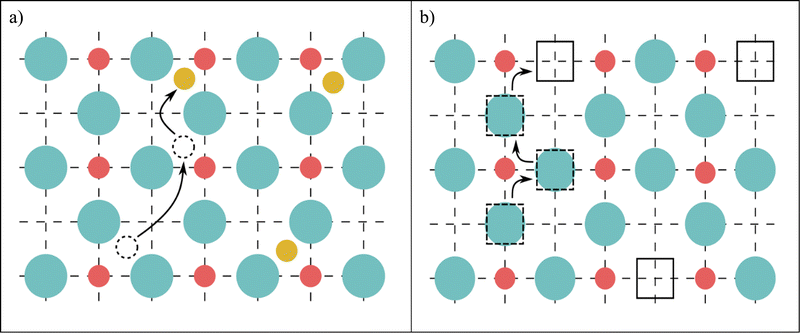 |
| Fig. 4 Schematic representation of surface segregation of (a) cation interstitials, and (b) oxygen vacancies in ionic solids, where yellow points represent Fe interstitials and squares are oxygen vacancies. | |
While the driving force of oxygen penetration is the difference in the chemical potential of oxygen between the gas phase and the lattice, the driving force in defect segregation is the difference in the chemical potential of segregating species between the bulk phase and the surface layer. In both cases, the difference diminishes with time until the driving force disappears. The observed long period of time required for surface equilibration, compared to the time of bulk equilibration, has been observed for both single crystals and polycrystalline specimens.7 Oxygen penetration from the gas phase can be treated as occuring in semi-infinite system from constant oxygen activity. Segregation, on the other hand, proceeds from a finite system, limited by the size of the specimen. Here, the concentration of species available for migration decreases with time within the bulk phase. Additionally, the expected appearance of the QISS with different bulk defect disorder changes the local environment of the segregating species, and hence their chemical potential as well. It is likely that these factors together lead to a much weaker segregation driving force and longer times necessary to achieve equilibrium. Owing to the dynamic character of the driving force of segregation, the equilibrium state is being approached asymptotically over a long period of time.
Surface defect disorder
Defect-related surface properties.
The observed WF changes shown in Fig. 2(a) and (b), which reflect an increase of the surface Fermi level position during oxidation and reduction experiments, by 0.2 eV and 0.6 eV, respectively, suggest that the applied prolonged annealing results in building-up of an n-type surface layer on top of the intrinsic bulk phase of hematite. As shown in Fig. 2(c), the defect-related parameter 1/mΦ, representing an average ionization degree of all defects within the surface layer, increases during the annealing from the initial value of 1/mΦ = 0 at t = 30 h, to the final value of 1/mΦ = 0.7, and these changes are consistent with the formation of a surface local structure. At the same time, the bulk-related parameter 1/mσ remains constant. The character of the observed dependence of 1/mΦversus time (for t > 30 h), which is similar to the dependence observed for TiO2 during annealing,15 suggests that the applied prolonged oxidation and reduction result in building up of the QISS. This effect is consistent with the expected long-range lateral interactions between defects within the surface layer that is enriched by segregation.7 The most surprising aspect of the obtained results is (i) the exceptionally long time required for equilibration of the surface layer, which is substantially longer than that required for bulk equilibration, and (ii) the increased surface Fermi level as a result of increased oxygen activity in the surrounding gas phase. It is very surprising that oxidation of the bulk phase results in reduction of the surface layer.
Surface versus bulk defect disorder.
Bulk properties of hematite in gas/solid equilibrium, within the oxygen activity range similar to that in this work, have been attested to as intrinsic semiconducting properties by both Change and Wagner23 in the temperature range 1223–1695 K, as well as Gleitzer et al.27 at 1253 K. Its intrinsic conduction is considered in terms of the Schottky-type defect disorder23 according to Kroger–Vink notation.37 | 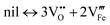 | (10) |
As seen in Fig. 2b, both reduction and oxidation experiments increase the surface Fermi level position. Such donor-like behavior could be explained by reaction (eqn (11)) occuring close to the surface:
| 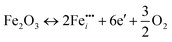 | (11) |
Alternatively, segregation-induced enrichment of the surface layer with donor-type defects may also be considered in terms of surface segregation of iron ions removed from their lattice sites in the bulk phase, where these defects are electrically neutral, and transported into interstitial positions in the surface layer where these ions act as donor-type defects:
| 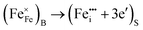 | (12) |
where the subscripts B and S correspond to the bulk and the surface layer, respectively. Surface segregation expressed by
eqn (12) results in imposition of negative surface charge.
Surface semiconducting properties
Work function as defect-related surface property.
Despite the complexity of defect disorder in crystals, the measurements of the WF changes allows to distinguish between the impact of donor- and acceptor-type defects on semiconducting properties. While the performed experiments included surface monitoring during oxidation and reduction, the determined WF changes indicate that in both cases the surface layer is reduced.
The obtained results imply that the surface of Fe2O3 is predominantly enriched with a donor-type defects, and their concentration exceeds their local solubility limit, hence triggering formation of the QISS.
Surface defect engineering (SDE)
Surface defect engineering.
The development of SDE is needed for the creation of active sites required for desired charge transfer.38 The obtained results indicate that the established time and p(O2) dependence of segregation may be used for the imposition of an optimal defect disorder that is required for desired performance by deliberate termination of the process at different stages of segregation.39 The analysis of the WF changes during prolonged annealing indicates that segregation of intrinsic defects results in the formation of the QISS. The results of this study demonstrate that the HTEP is uniquely positioned for in situ monitoring of surface segregation of intrinsic defects and the consequent impact on semiconducting properties. This case study on hematite and the reported effects may be used to tailor surface semiconducting properties and the associated reactivity. The insights gained in this work are relevant and directly applicable to further studies of surface defect properties and behaviour of intrinsically doped photoactive oxides in general.
Significance.
The significance of the reported WF data, representing the in situ monitoring of the gas/solid reactivity from surface perspective, can be considered in terms of the following points:
(1) The performance of energy materials, in terms of energy conversion efficiency, stability and reproducibility, requires recognition that their reactivity is profoundly affected by surface segregation that cannot be ignored even at room temperature.
(2) The reactivity of energy materials is determined by the QISS that is formed in the applied operational environment. Therefore, progress in processing of energy materials with desired performance requires SDE that can be guided by the HTEP instrument.
9. Surface restructures
Relevance of high-temperature studies to applications
The results, acquired at 1140 K, raise a pertinent question regarding their applicability to practical conditions, given that most devices do not operate at such elevated temperatures. It is generally true that many energy materials operate at ambient or moderate temperatures. However, a large majority of them are synthesized, fabricated, or processed at high temperatures and oxygen environments relevant to the experimental conditions employed in this study. Extended exposure to such temperatures and oxygen activities results in changes in their surface structure and properties as reported here. However, the significance of surface properties studied at elevated temperatures also involves the thermodynamic framework. So far, little is known about the impact of thermodynamics on surface properties. First, this framework allows the determination of surface properties that are independent of surface processing procedures, such as cleaning. The key significance of this approach is that for the first-time surface properties and bulk properties can be assessed in terms of a single concept of thermodynamics forming a bridge between solid-state science and surface science. The bridge opens access to the discovery of new materials with well-defined surface properties that can be predicted by thermodynamics. Moreover, this approach also opens-up new areas of high-temperature surface science and interface engineering.
Adsorption versus segregation
Surface reconstructions are commonly expected in recognition of the tendency of the surface to decrease the excess of surface energy. However, it has been commonly assumed that the key phenomenon associated with reduction of surface energy is adsorption of species derived from the gas phase. There is little information about surface segregation, which is the phenomenon of adsorption of species derived from the bulk phase. While the latter phenomenon has a substantial impact on surface properties, quantitative assessment of the related effect on surface properties is not a trivial matter. The results obtained in the present work indicate that the consequences of surface segregation of intrinsic defects on surface properties are substantial in terms of the resulting build-up of the QISS and the capacity to transfer charge. The reported data show that the HTEP is uniquely positioned for surface monitoring of the surface re-structure because of the QISS build-up. The latter effect indicates that:
(1) Surface segregation may be applied in SDE for modification of surface reactivity in a controlled manner,
(2) Surface segregation is profoundly affected by oxygen activity, and
(3) The rate of surface segregation, which is markedly slower than that of the gas/solid kinetics that is rate-controlled by lattice diffusion, imposes the need for a better understanding of the impact of the segregation-induced electric fields on surface restructure in operational conditions.
Impact of segregation on data reproducibility
It has been shown that metal oxides in the operational environment are covered by a segregation-induced low-dimensional quasi-isolated surface structure (QISS) formed on top of the bulk phase. Therefore, the reactivity of energy materials based on oxides is determined by this surface structure rather than the structure beneath the QISS. Moreover, processing of oxide semiconductors for solar water splitting entails recognition that (i) reproducible performance of solar cells requires the formation of a stable QISS, and (ii) the energy conversion efficiency is determined by its defect disorder. Consequently, the HTEP instrument that is positioned for in situ characterization of surface properties in gas/solid equilibrium, opens-up the area of high-temperature surface science dealing with unequivocal surface properties that are independent of surface processing procedures, such as cleaning.
10. Summary
The hematite phase
The in situ surface characterization of Fe2O3 by WF measurements in controlled oxygen activity indicates that surface semiconducting properties of the hematite phase change from intrinsic to n-type properties during annealing at 1140 K. This effect indicates a critical role of surface segregation of donor-type intrinsic defects (Fe interstitials and/or oxygen vacancies) in that transition, which are derived from lattice sites in the bulk phase. The equilibration within the Fe2O3–O2 system is rate-controlled by surface reaction, rather than bulk diffusion. The proposed surface reaction is the segregation-induced formation of the QISS that is charged negatively with respect to the bulk phase. Long annealing results in a slow drift of the defect-related parameter 1/mΦ. Its final value may be considered as an unequivocal surface property that is determined solely by the conditions of the equilibrium: oxygen activity and the temperature of annealing.
Other energy materials
The in situ monitoring of the charge transfer for other energy materials based on oxide semiconductors resulted in similar observations of surface reconstructions, leading to the formation of the QISS. It has been documented that isothermal oxidation and reduction at elevated temperatures lead to monotonous changes of defect-related surface quantities, including both 1/mΦ (eqn (3)) and EF (eqn (4)), over a prolonged period of time for NiO,8 FeO,9,10 Y-doped ZrO2,11,12 BaTiO314 and TiO2.15 In an exceptional case of CoO, imposition of monotonous changes of oxygen activity resulted in stepwise changes of the Fermi level suggesting the formation of a range of p(O2)-sensitive surface structures.13 In several instances, both single crystals and polycrystalline specimens have been studied.7 The observed effects confirm that the interpretation of the reactivity of energy materials requires recognition that (i) the phenomenon of surface segregation of species derived from the bulk phase leads to self-assembled QISS, and (ii) the reactivity and the related charge transfer are profoundly affected by the local defect disorder of this surface structure in operational conditions.
11. Conclusions
The established effect of surface defects on the performance of oxide materials in energy conversion, such as water oxidation, imposes the need to understand the impact of defects on the charge transfer associated with transitions of surface equilibria. Moreover, there is a need to develop SDE that allows imposition of an optimal surface defect disorder required for desired performance. Such studies require a defect-related surface sensitive tool that enables in situ characterization of the effect of surface defects on the charge transfer associated with surface reactions. The HTEP reported here is the tool that allows for advancement in the field of processing of energy materials. Furthermore, the HTEP offers unique capacity for in situ monitoring of the surface Fermi level during surface reactions, which allows experimental insight into the related charge transfer that is critical for the reaction progress. The related experimental studies show that:
(1) Surface semiconducting properties of oxide materials are profoundly affected by surface segregation leading to the formation of the QISS, which has a profound impact on the performance of materials for energy conversion devices, such as solar cells, fuel cells, catalysts and photocatalysts, and
(2) The HTEP is uniquely positioned for in situ surface monitoring of the effect of surface segregation of defects on surface semiconducting properties.
Abbreviations
HTEP | High-temperature electron probe |
CPD | Contact potential difference |
POS | Photosensitive oxide semiconductors |
QISS | Quasi-isolated surface structure |
SDE | Surface defect engineering |
WF | Work function |
Author contributions
Conceptualization (all); methodology (TB, JN); data processing (TB); Investigation (TB, JN); project administration (SAS, JN); writing – original draft (all); writing review and editing (all); illustration – TB & JN.
Conflicts of interest
The authors declare no conflict of interest.
Acknowledgements
This work has been performed within the UN program of Future Earth in partnership with Sustainable Energy Initiative Pty Ltd.
References
- Z. Zafar, S. Yi, J. Li, C. Li, Y. Zhu, A. Zada, W. Yao, Z. Liu and X. Yue, Recent Development in Defects Engineered Photocatalytis: An Overview of the Experimental and Theoretical Strategies, Energy Environ. Mater., 2022, 5, 68–114 CrossRef CAS.
-
P. Kofstad, Nonstoichiometry, Diffusion and Electrical Conductivity in Binary Metal Oxides, Wiley, New York, 1972 Search PubMed.
-
S. Mrowec, Defects and Diffusion in Solids, Elsevier, Amsterdam, 1980 Search PubMed.
- J. B. Wagner, Jr., Defects in Oxides, Am. Ceram. Soc. Bull., 1974, 53, 224–231 Search PubMed.
- W. Göpel, Chemisorption and Charge Transfer at Ionic Semiconductor Surface: Implication in Signing Sensors, Progr. Surf. Sci., 1985, 20, 9–103 CrossRef.
- M. Setvin, M. Wagner, M. Schmid, S. Parkinson and U. Diebold, Surface Point Defects on Bulk Oxides: Atomically-Resolved Scanning Probe Microscopy, Chem. Soc. Rev., 2017, 46, 1772–1784 RSC.
- J. Nowotny and T. Bak, Electron Probe for Surface Science and Surface Defect Engineering of Oxide Semiconductors for Sustainable Energy Conversion, J. Phys. Chem. C, 2020, 124, 20617–20642 CrossRef CAS.
- J. Nowotny, M. Sloma and W. Weppner, High-Temperature Kelvin Probe in Application to Ceramic Materials: Investigation of Nickel Oxide, J. Am. Ceram. Soc., 1989, 72, 564–570 CrossRef CAS.
- J. Nowotny and I. Sikora, Surface Electrical properties of the Wustite Phase, J. Electrochem. Soc., 1979, 125, 781–786 CrossRef.
- J. Nowotny and T. Bak, Surface versus Bulk Defect-Related Properties of the Wustite Phase (FeO) in Gas/Solid Equilibrium, ACS Appl. Energy Mater., 2020, 3, 9809–9816 CrossRef CAS.
- M. Yamawaki, T. Bak, M. K. Nowotny, J. Nowotny and C. C. Sorrell, Application of the High-Temperature Kelvin Probe for in situ Monitoring of the Charge Transfer at the Oxygen/Zirconia Interface. Oxygen Chemisorption Isobar, J. Phys. Chem. Solids, 2005, 66, 322–328 CrossRef CAS.
- T. Bak, E. D. Wachsman, K. E. Prince, K. A. Rahman and J. Nowotny,
In situ Surface Monitoring of Charge Transfer during Oxidation of Zirconia at Elevated Temperatures, ACS Appl. Energy Mater., 2019, 2, 2810–2817 CrossRef CAS.
- T. Bak, T. M. Gür, V. K. Sharma, J. Dodson, K. A. Rahman and J. Nowotny, Evidence of Low-dimensional Surface Structure for Oxide Materials: Impact on Energy Conversion, ACS Appl. Energy Mater., 2018, 1, 6469–6476 CrossRef CAS.
- J. Nowotny, Electron Probe for Unequivocal Surface Characterization and Surface Defect Engineering of Energy Materials. Example of BaTiO3, J. Phys. Chem. A, 2020, 124, 1610–1618 CrossRef CAS PubMed.
- T. Bak, T. M. Gür and J. Nowotny, Electron Probe bridging Solid-State Chemistry and Surface Chemistry: Example of TiO2–O2 System, ACS Appl. Energy Mater., 2023, 6, 865–875 CrossRef CAS.
-
W. Hirschwald, Selected Experimental Methods in the Characterization of Oxide Surface, in Surface and Near-Surface Chemistry of Oxide Materials, ed. J. Nowotny and L.-C. Dufour, Elsevier, Amsterdam, 1988, pp. 61–187 Search PubMed.
- Y. Lin, G. Yuan, S. Sheehan and D. Wang, Hematite-Based Solar Water Splitting: Challenges and Opportunities, Energy Environ. Sci., 2011, 4, 4862–6869 RSC.
- K. Sivula, F. Le Formal and M. Grötzel, Solar Water Splitting Progress Using Hematite (α-Fe203) Photoelectrodes, ChemSusChem, 2011, 4, 432–449 CrossRef CAS PubMed.
- B. Iandolo, B. Wickman, I. Zoric and A. Hellman, The Rise of Hematite: Origin and Strategies to Reduce the High Onset Potential for the Oxygen Evolution Reaction, J. Mater. Chem. A, 2015, 3, 16896–16912 RSC.
- A. G. Tamirat, J. Ricj, A. A. Dubale, W. N. Su and B. J. Hwang, Using Hematite for Photoelectrochemical Water Splitting: A Review of Current Progress and Challenges, Nanoscale Horiz., 2016, 1, 243–267 RSC.
- M. Rioult, D. Stanescu, E. Fonda, A. Barbier and H. Mangan, Oxygen Vacancies Engineering of Iron Oxide Films for Solar Water Splitting, J. Phys. Chem. C, 2016, 120, 7482–7490 CrossRef CAS.
-
A. J. Atanacio, T. Bak, K. A. Rahman and J. Nowotny, Defect Engineering of Photosensitive Oxide Materials. Example of TiO2 Solid Solutions, Adv. Inorg. Materials, Materials for Sustainable Energy, 2018, vol. 72, pp. 1–47 Search PubMed.
- R. H. Chang and J. B. Wagner, Jr., Direct-Current Conductivity and Iron Tracer Diffusion in Hematite at High Temperatures, J. Am. Ceram. Soc., 1972, 55, 211–213 CrossRef CAS.
- D. S. Tannhauser, Conductivity of Iron Oxides, J. Phys. Chem. Solids, 1982, 23, 25 CrossRef.
- R. Dieckmann, Point Defects and Transport in Hematite (Fe2O3), Philos. Mag. A, 1993, 68, 725–745 CrossRef CAS.
- O. N. Salmon, High Temperature Thermodynamics of the Iron Oxide System, J. Phys. Chem., 1961, 65, 550–556 CrossRef.
- C. Gleitzer, J. Nowotny and M. Rekas, Surface and Bulk Electrical Properties of the Hematite Phase Fe2O3, Appl. Phys. A: Solids Surf., 1991, 53, 310–316 CrossRef.
- J. Wang, H. N. Perry, L. Guo, L. Vayssieres and H. L. Tuller, On the Theoretical and Experimental Control of Defect Chemistry and Electrical and Photoelectrochemical Properties of Hematite Nanostructures, ACS Appl. Mater. Interfaces, 2019, 11, 2031–2041 CrossRef CAS PubMed.
- G. Somorjai and Y. Li, Impact of Surface Chemistry, Proc. Natl. Acad. Sci. U. S. A., 2011, 108, 917–924 CrossRef CAS PubMed.
- D. L. Freeman and J. D. Doll, The Influence of Diffusion on Surface Reaction Kinetics, J. Chem. Phys., 1983, 78, 6002–6010 CrossRef CAS.
- J. Lee and S. Han, Thermodynamics of Native Point Defects in α-Fe2O3: an ab Initio Study, Phys. Chem. Chem. Phys., 2013, 15, 18906–18914 RSC.
- S. Shousha, S. Khalil and M. Youssef, A Complete Ab Initio Thermodynamic and Kinetic Catalogue of the Defect Chemistry of Hematite α-Fe2O3, its Cation Diffusion and Sample Donor Dopants, Phys. Chem. Chem. Phys., 2021, 23, 25518–25532 RSC.
- F. Kraushofer, Z. Jakub, M. Bichler, J. Hulva, P. Drmota, M. Weinold, M. Schmid, M. Setvin, U. Diebold, P. Blaha and G. S. Parkinson, Atomic-Scale Structure of the Hematite α-Fe2O3 (1
02) “R-Cut” Surface, J. Phys. Chem. C, 2018, 122, 1657–1669 CrossRef CAS PubMed.
- F. Adam, B. Dupre, C. Gleitzer, J. Janowski, J. Nowotny and M. Sloma, Effect of Surface Macrodefects on the Mechanism of Oxygen Interaction with the Boundary Layer of Fe2O3, React. Solids, 1990, 8, 41–50 CrossRef CAS.
- T. Bak, I. Jasiuk, W. Sigmund, M. Yamawaki and J. Nowotny, Defect Chemistry of Titanium Diooxide: Evidence of the n-p Transition during Cooling, J. Phys. Chem. C, 2022, 126, 5014–5021 CrossRef CAS.
- Z. Adamczyk and J. Nowotny, Effect of Segregation on Near-Surface and Bulk Transport Phenomena in Ionic Crystals, J. Phys. Chem. Solids, 1986, 47, 11–27 CrossRef CAS.
- F. A. Kröger and H. Vink, Relations Between the Concentrations of Imperfections in Crystalline Solids, Solid State Phys., 1956, 3, 307–435 Search PubMed.
- S. Liu, L. Kang, J. Zhang, E. Jung, S. Lee and S.-C. Jun, Structural Engineering and Surface Modifications of MOF-Derived Cobalt-Based Hybrid Nanosheets for Flexible Solid-State Supercapacitors, Energy Stor. Mater., 2020, 32, 167–177 Search PubMed.
- J. R. H. Black and D. W. Kingery, Segregation of Aliovalent Solutes Adjacent Surfaces in MgO, J. Am. Ceram. Soc., 1979, 62, 176–184 CrossRef CAS.
Footnote |
† Center for Defect Engineering of Energy Materials, University of Florida, P.O. Box 116300, Gainesville, FL 32611-6300, USA. |
|
This journal is © The Royal Society of Chemistry 2024 |
Click here to see how this site uses Cookies. View our privacy policy here.