Surfactant-enhanced coagulation and flocculation improves the removal of perfluoroalkyl substances from surface water†
Received
25th March 2024
, Accepted 10th August 2024
First published on 13th August 2024
Abstract
Coagulation/flocculation is a widely used water and wastewater treatment process due to its low cost, simplicity, and effectiveness. However, the process is not effective in the treatment of per- and polyfluoroalkyl substances (PFAS), the presence and treatment of which is an ongoing challenge for water providers. Here, we explore cationic surfactant-enhanced coagulation as a process modification to target the removal of PFAS in existing coagulation/flocculation systems. Batch experiments, in jar testing apparatus, were performed to assess the removal of two short-chain and two long-chain PFAS at an initial concentration of 10 μg L−1 with the addition of cetyltrimethylammonium chloride (CTAC) as the coagulant-aid. Our findings suggest that elevated coagulant dose (60 mg L−1 of alum or 100 mg L−1 of FeCl3) coupled with the addition of a cationic surfactant (1 mg L−1 of CTAC) significantly enhanced the removal of both short-chain (perfluorobutane sulfonate: PFBS removal to >40%) and long-chain PFAS (perfluorooctanoic acid: PFOA and perfluorooctane sulfonate: PFOS removal to >80%), with FeCl3 showing better performance than alum. Sulfonates (PFBS, PFOS) were shown to be removed more efficiently compared to carboxylates (PFBA, PFOA), presumably due to their higher hydrophobicity leading to better interactions with the flocs. Furthermore, CTAC in combination with traditionally used additives such as powdered activated carbon (PAC), served as a better aid for PFAS treatment and improved the removal of PFBS, PFOA, and PFOS to >98%. This study highlights that introducing a cost-effective pre-treatment with a cationic surfactant to existing conventional treatment systems can improve the performance efficiency in treating PFAS-contaminated waters.
Environmental significance
Per- and polyfluoroalkyl substances (PFAS) are a class of highly persistent and toxic anthropogenic contaminants. Conventional coagulation process is not effective in removing PFAS from contaminated source water. In this study, we have shown that we can improve existing coagulation process with the use of readily available chemicals (cationic surfactants and activated carbon) to accomplish the removal of both suspended solids and regulated PFAS. This study therefore shows how we can achieve PFAS remediation at environmentally relevant levels without the need for extensive capital expenditure and modifications to existing treatment regimes.
|
1. Introduction
Coagulation is a popular water treatment technique for removing suspended solids, natural organic matter (NOM), and various particulates.1 Coagulation is generally carried out by adding hydrolysable metal salts, such as alum or ferric salts, into contaminated waters to form flocs, which are allowed to settle and then filtered out. The coagulation treatment efficiency is largely affected by the water source and the nature of the chemical constituents present. In theory, coagulation process mainly involves: (i) destabilization and/or charge neutralization of suspended and colloidal particulates; (ii) formation of hydrolysable metal flocculent particles, (iii) adsorption of the formed particles onto the flocs; and (iv) trapping the larger “colloidal-flocs aggregates” during the settlement process.2 In practice, coagulation processes are typically composed of three stages: (i) rapid mixing stage, where the dosed coagulant is dispersed into the water, (ii) slow mixing stage, where the hydrolysed coagulant species and contaminants aggregate together to form larger insoluble flocs, and (iii) sedimentation stage, where the flocs are allowed to settle under gravity, which can then be removed by filtration. Introduction of the coagulants into the water stream triggers a series of physicochemical reactions, which induce chemical destabilization of the charged particles, and ultimately agglomerate the large particles through perikinetic flocculation.3
Despite the merits of low-cost and wide application, the coagulation treatment efficiency for perfluoroalkyl substances (PFAS) in drinking water is limited yet contradictory. PFAS are ubiquitous contaminants of concern4–7 and select PFAS compounds were recently regulated by U.S. Environmental Protection Agency (US EPA) at levels as low as 4 ng L−1.8 Several studies have shown that conventional treatment processes, such as coagulation, ozonation, chlorination etc., are ineffective for PFAS removal.9–12 However, other studies have also reported impressive PFAS removal efficiencies by means of coagulation. A study by Deng et al. (2011) showed a high perfluorooctanoate (PFOA) removal (up to 90%) from surface water by polyaluminium chloride coagulation (PACl, Al2O3 = 29%).13 Their findings suggested a potential sorption of hydrophobic PFAS on the particles during the coagulation process, concluding that both electrostatic and hydrophobic interactions are involved in PFOA sorption. Other studies have also looked at the mechanisms for perfluorooctane sulfonate (PFOS) and PFOA removal during the coagulation process by examining different types of coagulants, the effects of different water-specific matrices (i.e., pH, NOM, turbidity, etc.) and coagulant specific parameters (i.e., type, dose, stirring time etc.).14–17 Studies have also looked at the impact of cationic polymers such as polydiallyldimethyl ammonium chloride (polyDADMAC) and polyamine, in improving PFOS/PFOA removal from contaminated groundwaters.18–20
To the author's knowledge, almost all the existing studies have focused on removing long-chain PFAS by coagulation. Studies on short-chain PFAS, such as perfluorobutanoate (PFBA) and perfluorobutane sulfonate (PFBS), removal by coagulation is very limited.21,22 To that end, the current study investigated parameters that affect short-chain PFAS removal by coagulation. As discussed previously, the primary/dominant mechanism of PFAS removal in coagulation is the hydrophobic interaction with flocs. In the present study, we hypothesized that the addition of a hydrophobic cationic surfactant can enhance the electrostatic interaction of short chain PFAS with the counter ion, and the overall hydrophobic and neutral ion-pair can be removed via sorption/entrapment within flocs. A similar process, called adsorptive micellar flocculation (AMF), has been successfully applied for the removal of other chemicals of concern (e.g., benzene, tetracycline).23–25 Similar to coagulation, AMF also works on the principles of micellization behavior of surfactants and their ability to form larger micellar-flocs. The physico-chemical processes in both coagulation and AMF are similar, including colloidal/micelle formation, charge neutralization, adsorption of flocculants, complexation of pollutant(s), and aggregation of the surfactant micelles/floccs.26 AMF, however, is targeted for the removal of contaminants from highly concentrated waters. In the present study, we propose to use a cationic surfactant as a coagulant-aid to improve the removal of PFAS at environmentally relevant levels in surface water.
2. Materials and methods
2.1 Chemical materials
Perfluorobutanoic acid (PFBA), perfluorobutane sulfonate (PFBS), perfluorooctanoic acid (PFOA), and perfluorooctane sulfonate (PFOS) were purchased from Sigma–Aldrich Chemical Co. Sodium chloride (NaCl), alum (Al2(SO4)3·18H2O, ferric chloride (FeCl3·6H2O) and polyDADMAC were purchased from Fisher Scientific. Powdered activated carbon (PAC) was sourced from Calgon Carbon. All solutions used in the experiments were prepared with Milli-Q water supplied by a Milli-Q Gradient-A 10 Millipore (resistance of 18.25 MΩ cm@25 °C). All chemicals used in the experiments were of reagent grade or higher.
2.2 Jar test experiments
Coagulation of PFAS was performed in a series of jar tests at different experimental parameters (coagulant, dosage, PAC, additives). The surface water used for the experiments was collected from Roth Pond (RP), located within the Stony Brook University campus. Bulk water samples were prepared by diluting the RP water with tap water at a 1
:
1 ratio. Prior to conducting the experiments, this water was analyzed for PFAS to determine background levels. All the analytes monitored (PFBA, PFBS, PFOA, PFOS) were below detection limits. Coagulation experiments were performed in 1 L polypropylene (PP) bottles using a conventional jar test apparatus. Experimental samples were prepared by sequentially adding 1.0 mmol per L NaCl (to provide ionic strength) followed by spiking with 10 ppb of each PFAS (equating to a total concentration of 40 ppb). Control experiments were performed without coagulants to assess the loss of PFAS due to adsorption onto the plastic bottles, paddles, and baffles. The PFAS spiked surface water was used in the coagulation experiments to study the effects of coagulant dose (1–100 mg L−1), and additives (polyDADMAC, CTAC and PAC) on the removal of PFAS. The jar test procedures consisted of a 60 s rapid mix (100 rpm), a 20 min slow mix (at 50 rpm), and a 30 min settling period. Following the coagulation process, PFAS concentration, pH values, and the turbidity were measured from the collected supernatants in each batch experiment. An aliquot of 20 mL of the undisturbed solution was collected from each jar. Of this, 15 mL was used for measuring the pH and turbidity and the remaining 5 mL was diluted with 5 mL methanol and filtered through a 0.45 μm PP filter.
2.3 PFAS analysis by LC-MS/MS
PFAS concentrations were measured using a high-performance liquid chromatography coupled to a triple-stage quadrupole mass spectrometer (Agilent 6495 HPLC-MS/MS, Agilent, USA). Chromatographic separation was performed using a ZORBAX Eclipse Plus C18 column (3 × 50 mm; 1.8 μm; Agilent) at 50 °C. The mobile phases comprised of MilliQ water fortified with 5 mM CH3COONH4 (solvent A) and methanol (solvent B). The flow rate was set at 0.4 mL min−1, giving a total run time of 15 min. HPLC gradient information is listed in Table S1.† The mass spectrometer was operated in negative mode with the scan type set to dynamic multiple reaction monitoring (dMRM). The MRM ions selected for PFAS identifications are listed in Table S2.† PFAS quantification was performed on Agilent Mass Hunter Quantitative Analysis (Agilent) based on a calibration curve corrected using labeled internal standard response. The limit of quantification (LOQ) was 0.01 ppb, and a valid peak was defined as the peak of analyte with signal-to-noise (S/N) ≥ 10. Internal standards (IS) were composed of four mass labeled compounds as 13C3-PFBA, 13C3-PFBS, 13C8-PFOA, 13C8-PFOS, and 1 ng IS was spiked in both the calibration standards and samples.
3. Results and discussion
3.1 Effects of coagulant dosage
As evident from Fig. 1a, PFAS removal by both alum and FeCl3 is minimal during conventional coagulation. At an alum dose of 50 mg L−1, the removal of PFOA was about 10% while that of PFOS was roughly 25%. Similarly, no removal of either PFOA or PFOS was observed with FeCl3 coagulation at the conventional dose of 5–15 mg L−1. This finding agrees with other studies that have also reported negligible removal of PFAS with conventional coagulation compared to other treatment techniques.10,14,15,27,28 Both types of coagulants failed to remove either of the short-chain PFAS (PFBA and PFBS). However, as the coagulant dose was increased (enhanced coagulation), the PFAS removal percentage also increased. At an alum dose of 60–75 mg L−1, the removal percentage of PFOA increased to about 44% while that of PFOS improved to 56%. Similarly, for FeCl3, at a dose of 100 mg L−1, the PFOA and PFOS removal was 28% and 36%, respectively (Fig. 1b). The higher removal of PFOS compared to that of PFOA could be attributed to the differences in their physical properties. PFOS being a sulfonate with an additional –CF2 group in its chain tends to be more hydrophobic than PFOA. PFOS has a higher Koc (log
Koc = 2.4–3.7) value than PFOA (log
Koc = 1.89–2.63), and hence would likely adsorb more on to the flocs. Further, PFOS, having a hard base sulfonic acid group, compared to PFOA, which has a soft base carboxylic acid group, is more likely to be readily adsorbed on oxide surfaces, which are hard acids.29,30 Cumulatively, the synergistic effects of the physico-chemical properties, including, functionality of the head group, the chemistry of the functional group and the molecular size can lead to a higher adsorption of PFOS to therefore a higher removal of PFOS by coagulation as seen in this work. However, higher alum dose (100 mg L−1) negatively affected the removal of PFAS (Fig. 1a), indicating that the optimal dose of FeCl3 was 100 mgL−1 and that of alum was 60 mg L−1 for long-chain PFAS removal from the tested surface water. The drop in the removal of PFAS was expected because overdosing of coagulants can lead to charge reversal and re-stabilization of particles, resulting in poor removal of colloids/turbidity.31–33 Hence, we suspect that electrostatic repulsion of anionic PFAS caused by the charge reversal led to the drop in performance observed in Fig. 1a. Even at the higher doses, neither alum (60 mg L−1) nor FeCl3 (60 mg L−1) was effective in short-chain PFBA and PFBS removal (Fig. 1). Our result indicates that conventional coagulation is ineffective for short-chain PFAS removal, while effective for long-chain PFAS removal when an optimal high dose is provided.
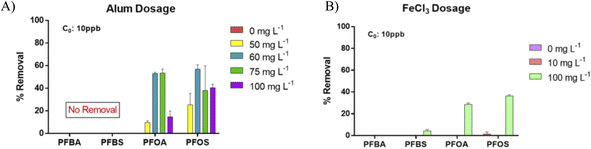 |
| Fig. 1 PFAS removal by conventional and enhanced coagulation. Panel A depicts PFAS removal by various doses of alum coagulant and Panel B depicts PFAS removal by various doses of ferric chloride (FeCl3) coagulant. Error bars represent mean ± SE (n = 2). | |
3.2 Additive-enhanced coagulation: effect of cationic additives
3.2.1 Cationic surfactant.
The most common environmentally relevant PFAS are anionic surfactants with varying –CF2 alkyl chain lengths and anionic functional groups. Quaternary ammonium compounds, like cetyltrimethylammonium bromide (CTAB), cetyltrimethylammonium chloride (CTAC) etc., are cationic salts with a hydrophobic linear alkyl group. As the hydrophilic head groups of PFOS and CTAC are inversely charged, the head groups of two surfactants are expected to associate with each other via electrostatic attraction. The resulting ion-pair complex will feature higher hydrophobicity and reduced critical micelle concentration (CMC), thus enhancing the formation of PFAS-CTAC ion-paired complexes.34,35 In the presence of iron or aluminum-based coagulants, these micelles can bind to the flocs formed and are subsequently removed by co-precipitation. Previous research proposed hydrophilic micelles formed between cationic surfactant cetyltrimethylammonium bromide (CTAB) and PFAS.36 Electrostatic interactions between the headgroups of oppositely charged surfactants is expected.37 When oppositely charged surfactants are mixed in aqueous solutions, bilayer or vesicles are formed spontaneously at concentrations far below their respective CMCs of either pure surfactant.38 Therefore, addition of cationic surfactants such as CTAC, will likely form ion pairs with PFOS or PFOA with reduced CMC, thereby enhancing their binding to the flocs to facilitate the coagulation process.39 As can be seen from Fig. 2, the enhanced coagulation of alum and FeCl3 with additive CTAC on PFAS removal. Fig. 2a compares coagulant FeCl3 removal of PFAS in the presence and absence of CTAC (1 mg L−1). Clearly, the presence of CTAC dramatically increased the PFAS removal irrespective of their fluoroalkyl chain length. PFBS removal increased from <1% to over 40% in the presence of the cationic surfactant during coagulation. Similarly, both PFOA and PFOS had over 80% removal. Yet, the presence of CTAC did not favor the removal of the short-chain carboxylic acid, PFBA. These results suggested that there may be competition within PFAS to electrostatically associate with CTAC, with long-chain PFAS preferably forming complexes with the cationic surfactant. This finding may likely be analogous to the observation that long chain PFAS are removed better in ion exchange resin systems compared to short chain PFAS.40Fig. 2b presents the impact of additive CTAC on PFAS removal with coagulant alum. In the presence of 1 mg L−1 CTAC surfactant concentration, the long-chain PFOA and PFOS removal rates were approximately 60% and ∼90% respectively at an alum dose of 60 mg L−1, similar to that of coagulant FeCl3. In contrast, the sorption was not promising with respect to both short-chain PFAS (Fig. 2b). At the alum dose of 60 mg L−1, the removal rates of PFBA and PFBS were <1% and <10%, respectively. Further, the addition of Suwannee River NOM (10 mg L−1) to the water matrix did not impact the removal of long-chain PFAS in the presence of CTAC (Fig. S1†). Increasing CTAC's dose to 10 ppm only minimally improved the removal of PFOA and PFOS (Fig. S1†), and hence we chose 1 ppm concentration for further experiments.
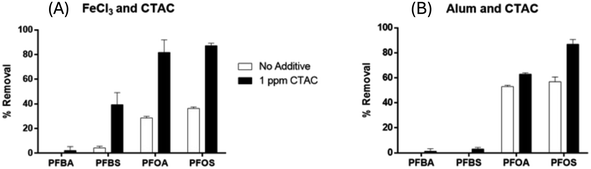 |
| Fig. 2 PFAS removal (in %) by surfactant-enhanced coagulation (A) 100 mg L−1 of ferric chloride (FeCl3) coagulant in the presence and absence of CTAC (1 mg L−1); and (B) 60 mg L−1 of alum coagulant in the presence and absence of CTAC (1 mg L−1). Error bars represent mean ± SE (n = 2). | |
3.2.2 Cationic polymer.
One of the common additives generally added to aid coagulation processes are cationic polymers. A popular choice is the cationic polymer, polydiallyldimethylammonium chloride (polyDADMAC), because of its high charge density and ability to operate over a wide range of pH.31,41,42 To examine if a synergistic enhancement of PFAS removal can be achieved with the addition of a cationic polymer along with a cationic surfactant, coagulation was performed with addition of CTAC followed by polyDADMAC. This sequential addition is necessary to facilitate the ionic interactions between the PFAS and CTAC, and then the subsequent interaction of the PFAS–CTAC complex with polyDADMAC, which can then be removed by the enhanced doses of the coagulants. As expected, in the presence of only polyDADMAC, only long chain PFAS removal was achieved to a certain degree with both alum and FeCl3 (Fig. 3). Interestingly, with the combination of both cationic surfactant and cationic polymer, improved PFAS removal was seen with iron-based coagulation compared with alum as the coagulant (Fig. 3a). Moreover, increasing the dose of CTAC improved the removal of short-chain sulfonic acids and achieved near complete removal of both the long-chain carboxylic and sulfonic acids (Fig. 3a). This further confirms that PFAS sorption on the flocs can be enhanced by increasing their size by ion pairing with cationic surfactants during the coagulation process. Polyelectrolytes such as polyDADMAC have been reported as precursors for N-nitrosodimethylamine (NDMA) formation, a known carcinogen.43–46 Hence, it can be argued that addition of cationic surfactants would be a relatively safer option than with the addition of cationic polymers like polyDADMAC alone or as a combination.
 |
| Fig. 3 Additive impact on PFAS removal (in %). Panel A depicts the removal of PFAS by FeCl3 coagulation in the presence of cationic surfactant CTAC and cationic polymer polyDADMAC. Panel B depicts the removal of PFAS by alum coagulation in the presence of cationic surfactant CTAC and cationic polymer polyDADMAC. | |
3.3 Enhanced removal of PFAS by PAC–CTAC-coagulation
The combination of coagulation and powder-activated carbon (PAC) has been previously used to improve organic pollutant removal in drinking water treatment plants.47,48 Previous studies have reported that the use of PAC for adsorption before coagulation has improved the removal efficiency of long-chain PFAS, particularly PFOA and PFOS.13,15 However, to the best of our knowledge, no studies have reported the removal of short-chain PFAS by means of coagulation. In this study, PAC–CTAC-coagulation samples were prepared by spiking the additive CTAC (1 mg L−1) followed by the addition of varying amounts (8 mg L−1 or 16 mg L−1) of PAC just before the coagulation process. In Fig. 4, the PFAS sample treated with PAC only (Fig. 4, blue bar) was ineffective in both short-chain and long-chain PFAS removal (<50%). Increasing the PAC dose from 8 mg L−1 to 16 mg L−1 did not significantly improve the PFAS removal irrespective of their chain length (Fig. 4, a: 8 mg L; b: 16 mg L−1, blue bar), indicating PFAS sorption was not majorly affected by the PAC dose at a low spiked level (8–16 mg L−1). However, when low-level PAC combined with coagulant FeCl3 (100 ppm, yellow bar), the long-chain PFOA and PFOS removal was improved from 14–45% to 90–96%, and short-chain PFBA and PFBS removal increased from 1–7% to 18–46%. The turbidity of the PAC-only samples (ESI Table S3,† 21.51–23.4) was much higher than that of PAC–FeCl3 integrated samples (ESI Table S3,† 0.81–2.05). The PAC–FeCl3 synergetic coagulation is more effective in long-chain PFAS removal than short-chain PFAS removal. This result is consistent with the previous observation that the coagulation process is more effective in long-chain PFAS than short-chain PFAS.
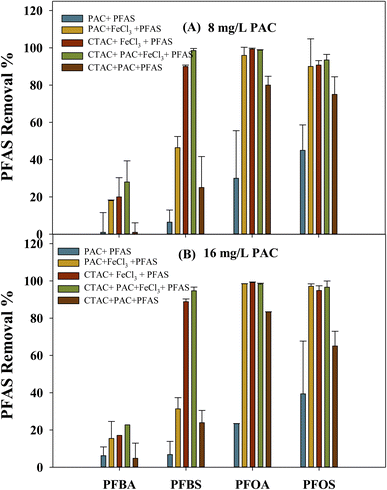 |
| Fig. 4 PFAS removal in Roth Pond water with (A) 8 mg L−1 and (B) 16 mg L−1 PAC (blue bar), PAC and coagulant FeCl3100 mg L−1 (yellow bar), CTAC (1 ppm) spiked with FeCl3 (red bar), CTAC (1 ppm) spiked with both PAC and FeCl3 (green bar), and CTAC spiked with PAC (brown bar). Error bars were derived from variants from duplicate experiments. | |
The synergetic impact of CTAC and PAC on the coagulation process of short-chain and long-chain PFAS was also examined. The CTAC–FeCl3 system (Fig. 4, 1 ppm, red bar) showed high removal for long-chain PFOA and PFOS (>90%), similar to the PAC–FeCl3 system (Fig. 4, a: 8 mg or b: 16 mg, yellow bar). Clearly, FeCl3 coagulation is effective in removing long-chain PFAS in the presence of either CTAC or PAC. For short-chain PFBS, the CTAC–FeCl3 showed much higher removal efficiency (96–98%) compared to the PAC–FeCl3 system (31–46%). Attached to a cationic functional group, the long chain (C16) CTAC is likely to form ion pairs with anionic PFBS via electrostatic interactions, thus increasing the formed ion pair complex's chain length and hydrophobicity.36 The improved PFBS sorption was likely caused by the increased chain length on the PFBS–CTAC ion pairs. Instead, PAC, without high surface cationic charges, was less effective in promoting PFBS sorption during the FeCl3 coagulation. Short-chain PFBA sorption was slightly improved under the CTAC–FeCl3 system compared to PAC–FeCl3. Less electronegativity from PFBA carboxylate functional group compared to the sulfonate group on PFBS is expected to limit the formation of CTAC–PFBA ion pairs, thus their removal in the coagulation system.36 Our data indicated that the addition of CTAC could better facilitate the removal of short-chain PFAS, especially for sulfonate compounds removal, compared to conventional PAC addition in the coagulation system.
The synergetic impact was also investigated by examining the coagulation process in the presence (Fig. 4, green bar) and absence of both CTAC and PAC (Fig. 4, brown bar). Without coagulant FeCl3, CTAC and PAC showed removal for long-chain PFOA and PFOS ranging from 78–82%, followed by PFBS removal at 23–25%, and lowest for PFBA removal of 4–6% (Fig. 4, brown bar). This sorption trend follows the PFAS chain length dependence that long-chain PFAS competed over short-chain PFAS on conventional adsorbents such as GAC, PAC, and resins.49 In the presence of coagulant FeCl3 (Fig. 4, green bar) high removal was achieved for long-chain PFOA and PFOS (>98%). Again, this result is consistent with previous observations that coagulation is effective in removing long-chain PFAS in the presence of PAC or CTAC. In the presence of FeCl3, short-chain PFBA and PFBS sorption were improved to 25% and >98%, respectively. The synergetic impact of PAC and CTAC with coagulant FeCl3 (Fig. 4, green bar) provided the best sorption for the short-chain PFBS and to an extent for PFBA.
4. Conclusions
Conventional coagulation and flocculation, though an economical and widespread water treatment process, is not an efficient technique to remove PFAS from surface water. With PFAS being considered for regulation in the parts-per-trillion levels by the US EPA and coagulation/flocculation systems being a very common treatment system across the nation, water providers are under stress to identify treatment alternatives. The present study has shown that by elevating the coagulant dose and by adding a cationic surfactant to the treatment setup, it is effective in removing regulated PFAS including both short and long-chain PFAS. The current study highlights that the application of low-cost modifications to existing conventional coagulation systems can improve its performance efficiency to treat PFAS-contaminated waters. We also reported that removal efficiency is dependent on chain-length as well as functional groups, with sulfonates showing better removal than carboxylates. The removal efficiency can be summarized as long chain sulfonates > long chain carboxylates > short chain sulfonates > short chain carboxylates. The removal of PFAS by cationic surfactant-enhanced coagulation appears to be influenced by both electrostatic and hydrophobic interactions. Further, our results suggested that CTAC served as a better coagulant-aid for PFAS treatment compared to the traditionally used polyDADMAC and PAC. A combination of CTAC and PAC further improved the treatment of PFAS in such systems. However, it needs to be pointed out that even though removal technologies, like the assisted coagulation technique described above, have shown to be effective in removing PFAS from contaminated water, they do not necessarily destroy PFAS. These techniques primarily involve physical mass transfer and only temporarily remove PFAS from the specific medium. It is to be noted that, almost all commercially implemented PFAS removal technologies such as ion exchange and granular activated carbon (GAC) follows the same principle of mass transfer. However, the pressing issue with these sequestration approaches is that the PFAS is not destroyed but continue to remain in the environment and can cause health risks. The water treatment plant residuals, spent GAC/resins and brine regenerant contains high concentrations of PFAS (>ppm), salt, and residual organic compounds. A potential remedial measure for this dilemma is combining such technologies with PFAS destruction techniques. Even though destructive technologies are still in the development stage, they have shown great promise to destroy PFAS compounds at the bench scale and small pilot-scale studies.50–52 Some of the promising destruction techniques include electron beam, electrochemical oxidation, photocatalysis, sonolysis etc.50,53 Also, the current work did not analyze for CTAC after treatment and hence future work should focus on the removal and fate of CTAC after treatment. Consequently, ongoing studies on destructive based techniques are being carried out in parallel to examine destruction of PFAS and QACs because QACs are also considered as emerging contaminants,54 and hence the risks associated with the use of CTAC should be further explored. The impact of surfactant addition on the overall treatment process and other co-contaminants (such as inorganic/organic ions etc.), should also be further assessed.
Data availability
The data supporting this article have been included as part of the ESI.†
Conflicts of interest
There are no conflicts to declare.
Acknowledgements
The authors acknowledge the financial support of this work by the US Department of Defense's Strategic Environmental Research and Development Program (SERDP: ER22-3438) and the Division of Chemical, Bioengineering, Environmental and Transport Systems (CBET - 2401203) of the US National Science Foundation. The authors would like to acknowledge the contributions of Ms Isabella Sha to the coagulation experiments, which was facilitated through the Simons Summer Research Program at Stony Brook University. The content is solely the responsibility of the authors and does not necessarily represent the official views of the sponsors.
References
- C. Kan and C. Huang, Coagulation monitoring in surface water treatment facilities, Water Sci. Technol., 1998, 38, 237–244 CAS.
- A. Matilainen, M. Vepsäläinen and M. Sillanpää, Natural organic matter removal by coagulation during drinking water treatment: a review, Adv. Colloid Interface Sci., 2010, 159, 189–197 CrossRef CAS PubMed.
- G. M. Fair and R. S. Gemmell, A mathematical model of coagulation, J. Colloid Sci., 1964, 19, 360–372 CrossRef.
- E. M. Sunderland, X. C. Hu, C. Dassuncao, A. K. Tokranov, C. C. Wagner and J. G. Allen, A review of the pathways of human exposure to poly-and perfluoroalkyl substances (PFASs) and present understanding of health effects, J. Exposure Sci. Environ. Epidemiol., 2019, 29, 131–147 CrossRef CAS PubMed.
- M. Nian, K. Luo, F. Luo, R. Aimuzi, X. Huo, Q. Chen, Y. Tian and J. Zhang, Association between prenatal exposure to PFAS and fetal sex hormones: are the short-chain PFAS safer?, Environ. Sci. Technol., 2020, 54, 8291–8299 CrossRef CAS PubMed.
- A. K. Venkatesan and R. U. Halden, National inventory of perfluoroalkyl substances in archived US biosolids from the 2001 EPA National Sewage Sludge Survey, J. Hazard. Mater., 2013, 252, 413–418 CrossRef PubMed.
- A. K. Venkatesan, C.-S. Lee and C. J. Gobler, Hydroxyl-radical based advanced oxidation processes can increase perfluoroalkyl substances beyond drinking water standards: results from a pilot study, Sci. Total Environ., 2022, 847, 157577 CrossRef CAS PubMed.
- F. Xiao, B. Deng, D. Dionysiou, T. Karanfil, K. O'Shea, P. Roccaro, Z. J. Xiong and D. Zhao, Cross-national challenges and strategies for PFAS regulatory compliance in water infrastructure, Nature Water, 2023, 1, 1004–1015 CrossRef.
- S. Takagi, F. Adachi, K. Miyano, Y. Koizumi, H. Tanaka, I. Watanabe, S. Tanabe and K. Kannan, Fate of perfluorooctanesulfonate and perfluorooctanoate in drinking water treatment processes, Water Res., 2011, 45, 3925–3932 CrossRef CAS PubMed.
- C. Eschauzier, E. Beerendonk, P. Scholte-Veenendaal and P. De Voogt, Impact of treatment processes on the removal of perfluoroalkyl acids from the drinking water production Chain, Environ. Sci. Technol., 2012, 46, 1708–1715 CrossRef CAS PubMed.
- T. D. Appleman, C. P. Higgins, O. Quiñones, B. J. Vanderford, C. Kolstad, J. C. Zeigler-Holady and E. R. V. Dickenson, Treatment of poly- and perfluoroalkyl substances in U.S. full-scale water treatment systems, Water Res., 2014, 51, 246–255 CrossRef CAS PubMed.
- Y.-G. Park, W. H. Lee and K. Kim, Different adsorption behavior between perfluorohexane sulfonate (PFHxS) and perfluorooctanoic acid (PFOA) on granular activated carbon in full-scale drinking water treatment plants, Processes, 2021, 9, 571 CrossRef CAS.
- S. Deng, Q. Zhou, G. Yu, J. Huang and Q. Fan, Removal of perfluorooctanoate from surface water by polyaluminium chloride coagulation, Water Res., 2011, 45, 1774–1780 CrossRef CAS PubMed.
- F. Xiao, M. F. Simcik and J. S. Gulliver, Mechanisms for removal of perfluorooctane sulfonate (PFOS) and perfluorooctanoate (PFOA) from drinking water by conventional and enhanced coagulation, Water Res., 2013, 47, 49–56 CrossRef CAS PubMed.
- Y. Bao, J. Niu, Z. Xu, D. Gao, J. Shi, X. Sun and Q. Huang, Removal of perfluorooctane sulfonate (PFOS) and perfluorooctanoate (PFOA) from water by coagulation: mechanisms and influencing factors, J. Colloid Interface Sci., 2014, 434, 59–64 CrossRef CAS PubMed.
- B. K. Pramanik, S. K. Pramanik and F. Suja, A comparative study of coagulation, granular- and powdered-activated carbon for the removal of perfluorooctane sulfonate and perfluorooctanoate in drinking water treatment, Environ. Technol., 2015, 36, 2610–2617 CrossRef CAS PubMed.
- H.-Z. Zhao, L. Wang, Y.-Y. Chang and Y. Xu, High-efficiency removal of perfluorooctanoic acid from water by covalently
bound hybrid coagulants (CBHyC) bearing a hydrophobic quaternary ammonium group, Sep. Purif. Technol., 2016, 158, 9–15 CrossRef CAS.
- Y. H. Aly, D. P. McInnis, S. M. Lombardo, W. A. Arnold, K. D. Pennell, J. Hatton and M. F. Simcik, Enhanced adsorption of perfluoro alkyl substances for in situ remediation, Environ. Sci.: Water Res. Technol., 2019, 5, 1867–1875 RSC.
- C. Liu, J. Hatton, W. A. Arnold, M. F. Simcik and K. D. Pennell,
In situ sequestration of perfluoroalkyl substances using polymer-stabilized powdered activated carbon, Environ. Sci. Technol., 2020, 54, 6929–6936 CrossRef CAS PubMed.
- P. Ramos, S. Singh Kalra, N. W. Johnson, C. M. Khor, A. Borthakur, B. Cranmer, G. Dooley, S. K. Mohanty, D. Jassby, J. Blotevogel and S. Mahendra, Enhanced removal of per- and polyfluoroalkyl substances in complex matrices by polyDADMAC-coated regenerable granular activated carbon, Environ. Pollut., 2022, 294, 118603 CrossRef CAS PubMed.
- J. W. Park, J. H. Noh, S. W. Yoon, S. Samiya, B. G. Choi, G.-B. Kim, H. Oh and S. K. Maeng, Removal of short- and long-chain perfluorinated compounds from surface water by coagulation, Membr. Water Treat., 2021, 12, 187–194 Search PubMed.
- Y.-F. Li, C.-Y. Ho, Y.-J. Liu, Y.-C. Lee, C.-Y. Hu and S.-L. Lo, Enhance electrocoagulation-flotation (ECF) removal efficiency perfluorohexanoic acid (PFHxA) by adding surfactants, J. Environ. Chem. Eng., 2024, 12, 111773 CrossRef CAS.
- H. Demissie, S. Lu, R. Jiao, L. Liu, Y. Xiang, T. Ritigala, F. O. Ajibade, H. K. M. Mihiranga, G. An and D. Wang, Advances in micro interfacial phenomena of adsorptive micellar flocculation: principles and application for water treatment, Water Res., 2021, 202, 117414 CrossRef CAS PubMed.
- H. Sultana, T. H. Bokhari and M. Usman, Adsorptive micellar flocculation (surfactant-based phase separation technique): theory and applications, J. Mol. Liq., 2021, 323, 115001 CrossRef CAS.
- L. A. Ratkievicius, F. J. V. da Cunha Filho, R. P. F. Melo, B. R. de Vasconcelos, L. d. J. N. Duarte, F. W. B. Lopes and E. L. de Barros Neto, Removal of hydrochlorothiazide micropollutant from synthetic pharmaceutical effluent by ionic flocculation, J. Dispersion Sci. Technol., 2023, 44, 1574–1583 CrossRef CAS.
- P. R. M. Cavalcante, R. P. F. Melo, T. N. Castro Dantas, A. A. Dantas Neto, E. L. Barros Neto and M. C. P. A. Moura, Removal of phenol from aqueous medium using micellar solubilization followed by ionic flocculation, J. Environ. Chem. Eng., 2018, 6, 2778–2784 CrossRef CAS.
- G. A. Zoumpouli, D. Herron, A. Thornton, B. Jefferson and P. Campo, The role of coagulation on the fate of PFAS, brominated flame retardants and other trace contaminants in tertiary wastewater treatment for phosphorus control, Sci. Total Environ., 2023, 887, 163982 CrossRef CAS PubMed.
- M. Hubert, T. Meyn, M. C. Hansen, S. E. Hale and H. P. H. Arp, Per- and polyfluoroalkyl substance (PFAS) removal from soil washing water by coagulation and flocculation, Water Res., 2024, 249, 120888 CrossRef CAS PubMed.
-
V. L. Snoeyink and D. Jenkins, Water Chemistry, John Wiley & Sons, 1991 Search PubMed.
- M. Wiśniewski and P. Gauden, Pearson's hard-soft acid-base principle as a means of interpreting the reactivity of carbon materials, Adsorpt. Sci. Technol., 2006, 24, 389–402 CrossRef.
- M. A. Yukselen and J. Gregory, Properties of flocs formed using different coagulants, Water Supply, 2002, 2, 95–101 CrossRef CAS.
- J. Duan and J. Gregory, Coagulation by hydrolysing metal salts, Adv. Colloid Interface Sci., 2003, 100, 475–502 CrossRef.
- Q. H. Malik, Performance of alum and assorted coagulants in turbidity removal of muddy water, Appl. Water Sci., 2018, 8, 40 CrossRef.
- M. Goldraich, J. R. Schwartz, J. L. Burns and Y. Talmon, Microstructures formed in a mixed system of a cationic polymer and an anionic surfactant, Colloids Surf., A, 1997, 125, 231–244 CrossRef CAS.
- G. Kume, M. Gallotti and G. Nunes, Review on anionic/cationic surfactant mixtures, J. Surfact. Deterg., 2008, 11, 1–11 CrossRef CAS.
- R. Li, O. F. Isowamwen, K. C. Ross, T. M. Holsen and S. M. Thagard, PFAS–CTAB complexation and its role on the removal of PFAS from a lab-prepared water and a reverse osmosis reject water using a plasma reactor, Environ. Sci. Technol., 2023, 57, 12901–12910 CrossRef CAS PubMed.
- N. Eleraky, D. F. Mohamed, M. Attia, G. Pauletti and J. Winkle, Hydrophobic ion-pairing of low molecular weight heparin with cetyltrimethylammonium bromide, Int. J. Pharm. Sci. Res., 2015, 6, 558–565 CAS.
- M. T. Yatcilla, K. L. Herrington, L. L. Brasher, E. W. Kaler, S. Chiruvolu and J. A. Zasadzinski, Phase behavior of aqueous mixtures of cetyltrimethylammonium bromide (CTAB) and sodium octyl sulfate (SOS), J. Phys. Chem., 1996, 100, 5874–5879 CrossRef CAS.
- Y. F. Li, T. Fang, Y. C. Lee, Y. J. Liu, C. Y. Hu and S. L. Lo, Cationic surfactants influencing the enhancement of energy efficiency for perfluorooctanoic acid (PFOA) removal in the electrocoagulation-flotation (ECF) system, Chemosphere, 2023, 318, 137932 CrossRef CAS PubMed.
- T. H. Boyer, Y. Fang, A. Ellis, R. Dietz, Y. J. Choi, C. E. Schaefer, C. P. Higgins and T. J. Strathmann, Anion exchange resin removal of per-and polyfluoroalkyl substances (PFAS) from impacted water: a critical review, Water Res., 2021, 200, 117244 CrossRef CAS PubMed.
- W. Lee and P. Westerhoff, Dissolved organic nitrogen removal during water treatment by aluminum sulfate and cationic polymer coagulation, Water Res., 2006, 40, 3767–3774 CrossRef CAS PubMed.
- D. A. Cornwell and R. A. Brown, Replacement of alum and PolyDADMAC with natural polymers—turbidity removal and residuals reduction impacts, J. AWWA, 2017, 109, E252–E264 Search PubMed.
- S.-H. Park, P. Piyachaturawat, A. E. Taylor and C.-H. Huang, Potential N-nitrosodimethylamine (NDMA) formation from amine-based water treatment polymers in the reactions with chlorine-based oxidants and nitrosifying agents, Water Supply, 2009, 9, 279–288 CrossRef CAS.
- D. Hanigan, J. Zhang, P. Herckes, E. Zhu, S. Krasner and P. Westerhoff, Contribution and removal of watershed and cationic polymer N-nitrosodimethylamine Precursors, J. AWWA, 2015, 107, E152–E163 CrossRef.
- S. W. Krasner, W. A. Mitch, D. L. McCurry, D. Hanigan and P. Westerhoff, Formation, precursors, control, and occurrence of nitrosamines in drinking water: A review, Water Res., 2013, 47, 4433–4450 CrossRef CAS.
- D. An, Y. Chen, B. Gu, P. Westerhoff, D. Hanigan, P. Herckes, N. Fischer, S. Donovan, J. P. Croue and A. Atkinson, Lower molecular weight fractions of PolyDADMAC coagulants disproportionately contribute to N-nitrosodimethylamine formation during water treatment, Water Res., 2019, 150, 466–472 CrossRef CAS PubMed.
- M. B. Dixon, Y. Richard, L. Ho, C. W. K. Chow, B. K. O'Neill and G. Newcombe, A coagulation–powdered activated carbon–ultrafiltration – multiple barrier approach for removing toxins from two Australian cyanobacterial blooms, J. Hazard. Mater., 2011, 186, 1553–1559 CrossRef CAS PubMed.
- C. Sheng, A. G. A. Nnanna, Y. Liu and J. D. Vargo, Removal of Trace pharmaceuticals from water using coagulation and powdered activated carbon as pretreatment to ultrafiltration membrane system, Sci. Total Environ., 2016, 550, 1075–1083 CrossRef CAS PubMed.
- Y. Zhang, A. Thomas, O. Apul and A. K. Venkatesan, Coexisting ions and long-chain per- and polyfluoroalkyl substances (PFAS) inhibit the adsorption of short-chain PFAS by granular activated carbon, J. Hazard. Mater., 2023, 460, 132378 CrossRef CAS PubMed.
- K. Londhe, C.-S. Lee, Y. Zhang, S. Grdanovska, T. Kroc, C. A. Cooper and A. K. Venkatesan, Energy evaluation of electron beam treatment of perfluoroalkyl substances in water: a critical review, ACS ES&T Eng., 2021, 1, 827–841 Search PubMed.
- K. Londhe, C.-S. Lee, S. Grdanovska, R. Smolinski, N. Hamdan, C. McDonough, C. Cooper and A. K. Venkatesan, Application of electron beam technology to decompose per-and polyfluoroalkyl substances in water, Environ. Pollut., 2024, 348, 123770 CrossRef CAS PubMed.
- K. Londhe and A. K. Venkatesan, Effect of chain length, electrolyte composition and aerosolization on the removal of per-and polyfluoroalkyl substances during electrochemical oxidation, Environ. Sci. Water Res. Technol., 2024, 10, 1377–1388 RSC.
- J. N. Meegoda, B. Bezerra de Souza, M. M. Casarini and J. A. Kewalramani, A review of PFAS destruction technologies, Int. J. Environ. Res. Public Health, 2022, 19, 16397 CrossRef PubMed.
- W. A. Arnold, A. Blum, J. Branyan, T. A. Bruton, C. C. Carignan, G. Cortopassi, S. Datta, J. DeWitt, A.-C. Doherty and R. U. Halden, Quaternary ammonium compounds: a chemical class of emerging concern, Environ. Sci. Technol., 2023, 57, 7645–7665 CrossRef CAS PubMed.
|
This journal is © The Royal Society of Chemistry 2024 |
Click here to see how this site uses Cookies. View our privacy policy here.