Fluorotelomer ethoxylates cause developmental toxicity in mice
Received
2nd October 2023
, Accepted 1st March 2024
First published on 7th March 2024
Abstract
Poly- and perfluoroalkyl substances are a ubiquitous class of compounds which are considered persistent organic pollutants. Many of these compounds are unregulated and understudied but are still widely used. One group of these compounds are fluorotelomer ethoxylates, which recently emerged as compounds of interest following their detection in the environment. To determine the health impacts of these persistent compounds, healthy pregnant CD-1 mice were exposed to 0 ng L−1 (n = 8), 5 ng L−1 (n = 8), or 100 ng L−1 (n = 7) fluorotelomer ethoxylates in drinking water throughout gestation. At gestational day 17.5 (term is 18.5 days), high-frequency ultrasound was performed to investigate the placental and fetal hemodynamic responses following exposure. Maternal exposure to fluorotelomer ethoxylates showed evidence of placental insufficiency, with a significant increase in placental weights (p < 0.05), a decrease in the umbilical artery blood flow (p < 0.01) and vasodilation of the cerebral circulation (p < 0.01), consistent with brain sparing to preserve oxygen delivery to the brain. These results demonstrate that fluorotelomer ethoxylates cause developmental toxicity and motivate further work to evaluate the risk to human pregnancies and other vulnerable populations.
Environmental significance
Canadians spend >90% of their time indoors and inhalation of indoor dust particles is a significant source of exposure to poly- and perfluoroalkyl substances (PFAS). Our group recently discovered fluorotelomer ethoxylates (FTEOs), an unregulated group of PFAS, in dust samples and in industrial wastewater. Knowing that these compounds are persistent in the indoor environment, we aimed to study their health impact during the vulnerable period of pregnancy. This work used experimental laboratory mice and high-frequency ultrasound to determine how maternal exposure to FTEOs impact pregnancy, fetal growth and placental function. We found FTEO exposure at environmentally relevant concentrations resulted in placental insufficiency and fetal distress. These results motivate further studies to evaluate the risk to human pregnancies and inform environmental regulations.
|
1. Introduction
Poly- and perfluoroalkyl substances (PFAS) are a group of compounds that have a fluorinated carbon chain and a wide variety of functional groups. These compounds are considered to be persistent organic pollutants due to the slow rate at which they degrade and their ability to bioaccumulate. The unique properties of PFAS have led to widespread use across many different sectors including textiles, cosmetics and personal care products, and electroplating.1 It is thus unsurprising that many PFAS and their precursors have been detected in biological and environmental media.2
PFAS are often separated into two classes: legacy PFAS and replacement PFAS. Legacy PFAS consist of compounds such as perfluorooctanoic acid (PFOA) and perfluorooctane sulfonate (PFOS) which were at one time widely used, but have since been phased out following investigations into their impacts on human health and subsequent regulation. PFOA in particular has previously been linked to thyroid disease,3 testicular and kidney cancers,4 and ulcerative colitis5 in humans. Although legacy PFAS are now rarely used, their persistence has allowed them to remain in the environment, thus exposure to legacy PFAS is still a cause for concern. Replacement PFAS are new compounds being introduced to the market to replace the use of legacy PFAS. Legacy and replacement PFAS often share structural and compositional similarities.6 Yet, replacement PFAS are largely unregulated and often understudied leading to uncertainty around their environmental and health impacts. There are currently over 12
000 PFAS of interest,7 demonstrating how challenging it would be to characterize the effects of each compound. It is therefore important to perform environmental monitoring to identify key PFAS for further study.8
Recently, our group conducted nontargeted screening9 in industrial wastewater and indoor dust and found fluorotelomer ethoxylates (FTEOs) at concentrations similar to toxic PFAS such as PFOA and PFOS.10 FTEOs were detected in 9/15 indoor dust samples and 14/37 industrial wastewater samples, with higher concentrations associated with industries such as healthcare, electroplating, cosmetics and personal care products, and linen cleaning. Due to the variety of locations in which FTEOs were found, they likely have many different applications. The prevalence of these unregulated compounds and their unknown toxicity provided the motivation to study in vivo exposure effects of FTEOs in mice, specifically during the vulnerable period of pregnancy and fetal development.
The effects of legacy PFAS on pregnancy and development in mice has attracted significant interest and concern.11 Maternal exposure to PFOA and PFOS in mice has shown that these compounds cross the placental barrier12–14 and result in significant changes in placental weight and differences in placental morphology.15–17 These changes have been associated with negative pregnancy outcomes, such as fetal growth restriction and prenatal mortality, raising concerns about gestational effects of PFAS in humans. While FTEOs have not been studied in vivo during pregnancy before, FTEOs have been shown to cause significant in vitro cytotoxicity and adipogenic activity in murine cells.18 Moreover, while studies of the biodegradation of FTEOs present mixed results, they may degrade into perfluorinated carboxylic acids, including PFOA.19,20 As such, we hypothesize that FTEOs will also have a detrimental effect on pregnancy and fetal development.
Doppler ultrasound is the standard tool for evaluating placental health and fetal well being in both human and mouse pregnancy.21 In the present study, we investigated the effect of maternal exposure to FTEOs on placental and fetal growth using experimental mice. High-frequency Doppler ultrasound was used to characterize the impact on placental function and the placental and fetal hemodynamic responses following exposure to FTEOs.
2. Materials and methods
2.1 Chemicals
Capstone FS-30 (CAS Number 1640092-35-8), a commercial mixture of 6
:
2 FTEOs, was purchased from Apollo Scientific (Dredbury, Stockport, UK) (Fig. 1). 1H NMR and pulsed field gradient NMR were used to confirm the purity of the Capstone FS-30 mixture. Measurements were acquired on a Bruker Avance II 600 MHz spectrometer (1H Larmor frequency 600.29 MHz) and a Bruker diffusion Diff30 probe with a 1H radiofrequency coil insert with an inner diameter of 5 mm.
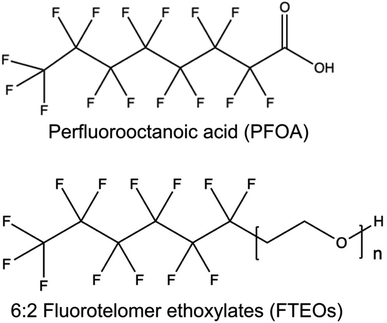 |
| Fig. 1 Chemical structure of perfluorooctanoic acid (PFOA) and 6 : 2 fluorotelomer ethoxylates (FTEOs). | |
2.2 Animals
Twenty three female CD-1 mice were purchased from Charles River Laboratories and bred in-house between 6 and 15 weeks of age. Detection of a vaginal plug the morning after mating was designated as gestational day 0.5 (GD0.5), at which time mice were singly housed to monitor water consumption. From GD0.5 to GD17.5, mice were provided with filtered drinking water containing either 5 ng L−1 6
:
2 FTEOs, 100 ng L−1 6
:
2 FTEOs, or control filtered water (three-phase micrometer filtration system at 5, 1 and 0.2 μm). 6
:
2 FTEOs were used as they were the most prevalent in indoor dust and industrial wastewater.10 The concentrations were selected based on reported concentrations of other PFAS in drinking water22,23 and the upper end of concentrations of individual FTEOs found in industrial wastewater.10 At GD17.5, high-frequency ultrasound was conducted to measure blood flow in the umbilical artery (UA) and middle cerebral artery (MCA). Dams were euthanized following ultrasound imaging using cervical dislocation. Dissections were performed to measure umbilical cord lengths, fetal weights, and placental weights. Tail or skin samples were taken for use in genotyping and stored in a −18 °C freezer. Genotyping to determine fetal sex was conducted using polymerase chain reaction (PCR) and primers designed for the sex-determining region Y (Sry) gene. These primers had forward primer sequence (CTCATCGGAGGGCTAAAGTG) and reverse primer sequence (AAGCTTTGCTGGTTTTTGGA) and a total segment length of 166 base pairs. Primers for the Cyp24a1 gene were used as a positive control and had forward sequence (CCAAGTGTGCCATTCACAAC) and reverse sequence (TCTCTCGCTGAACCTGG ATT). All experiments were conducted in accordance with guidelines established by the Canadian Council on Animal Care and were approved by the Institutional Care Committee at Memorial University of Newfoundland (Animal Use Protocol 20-02-LC).
2.3 High-frequency ultrasound biomicroscopy
A high-frequency ultrasound system (F2, VisualSonics, Toronto, Canada) was used with a UHFx57 probe at a center frequency of 40 MHz and an axial resolution of 40 μm. Two fetuses in the lower abdomen of each dam were chosen for ultrasound. This ultrasound protocol was described previously.21 Briefly, dams were anesthetized using isoflurane at 4% for induction and 2% for maintenance in 100% oxygen. The maternal heart rate, respiration rate and temperature were monitored throughout the experiment and the maternal temperature was maintained at 35–37 °C using a temperature-regulated platform. These parameters are best practices for measurements of physiology in experimental mice.24 At a constant level of 2% isoflurane, the maternal heart rate is within physiological range (>400 bpm) and there was no significant difference between experimental groups (450 (CI: 430–470) bpm). The blood velocity was measured using pulsed wave Doppler recordings and accounting for the angle of insonation (<60°). The diameter measurements of the UA were determined using M-mode recordings with the ultrasound beam at the same location as the Doppler measurement. M-Mode datasets from three of the fetuses in the 5 ng L−1 FTEO group had to be excluded because of failure to find a well-defined M-mode trace in the correct location.
All values were calculated using an average over three cardiac cycles. The velocity time integral was calculated by tracing the intensity-weighted mean velocity of the Doppler waveform as a function of time. The vessel diameter was measured at systole and diastole using the inner boundaries of the vessel walls. The flow was calculated by multiplying the velocity time integral by the vessel cross-sectional area and the fetal heart rate. The pulsatility index (PI) was calculated using the peak systolic and diastolic velocities, subtracting the two and dividing by the mean velocity over the cardiac cycle. The cerebroplacental ratio (CPR) was calculated as the MCA PI divided by the UA PI.25
2.4 Statistical analysis
All statistical analyses were carried out using R software (http://www.r-project.org/) where statistical significance was defined as p < 0.05. Data from each group of animals are reported as the means and 95% confidence intervals (CI). The maternal weights, litter sizes, male
:
female ratio, and number of fetal resorptions were analyzed using a one-way analysis of variance (ANOVA) to evaluate the effect of concentration (0, 5, 100 ng L−1 FTEOs). Litter was a significant factor in the fetal and placental weights, umbilical cord lengths, and feto-placental weight ratios and therefore litter means were used for statistical analysis using a two-way ANOVA to evaluate the effects of concentration (0, 5, 100 ng L−1 FTEOs) and biological fetal sex (female, male). If the ANOVA was significant, Tukey post hoc tests were performed. The UA blood flow, UA diameter, fetal heart rate, UA PI, MCA mean velocity, MCA PI, and CPR were analyzed using linear mixed-effects models with concentration (0, 5, 100 ng L−1 FTEOs) as the fixed effect and litter as the random effect to account for similarity amongst littermates.26 If the linear mixed-effect model was significant, post-hoc tests were performed by relevelling the model with different experimental groups chosen as the reference group.
3. Results and discussion
There was no difference in maternal weight prior to mice being randomly assigned to the 5 ng L−1 FTEOs, 100 ng L−1 FTEOs or control group and there was no difference between groups in the maternal weight gain at GD17.5. The dams all consumed the same amount of water, an average of 8 (CI: 7–9) mL per day. None of the dams showed any visible signs of illness (e.g. lethargy, hunched posture, unkempt fur). There was no effect of concentration on the litter size, male
:
female ratio, or number of resorptions. This demonstrates that maternal health is not significantly affected by exposure to the concentrations of FTEOs used in this study, and thus any fetal effects are not likely attributed to maternal illness.
At GD17.5, there was no effect of fetal sex or concentration on the umbilical cord length. For fetal and placental weights, we observed a trend towards an increase in males compared to females (p = 0.06 and p = 0.07, two-way ANOVA). While there was no effect of concentration on the fetal weights (Fig. 2a), there was a significant effect of concentration on placental weights (p < 0.01), with the placentas from the 100 ng L−1 FTEOs group weighing 14% more than controls (p < 0.05, by Tukey post hoc test following two-way ANOVA) (Fig. 2b). There was also a significant effect of concentration on the feto-placental weight ratio (FPWR) (p < 0.05), with a decrease of 9 and 10% in the 5 ng L−1 and 100 ng L−1 FTEO groups compared to controls (p < 0.05, by Tukey post hoc test following two-way ANOVA) (Fig. 2c). A decreased FPWR is associated with adverse pregnancy outcomes27 and suggests a decrease in placental efficiency, requiring a larger placenta to support the same fetal growth trajectory as during normal gestation. In human pregnancy, several fetal morbidities have been associated with increased placental weight including low Apgar scores, neonatal seizures, and the requirement of neonatal ventilation.28 Additionally, it has been proposed that placental weights may be increased as a compensatory mechanism due to decreased blood flow to the fetus.29 This indicates that, despite the lack of changes in fetal weights, FTEO exposure may be causing stress on the fetoplacental unit requiring additional oxygen and nutrients for the fetus to reach its full growth potential. To further explore the impact of FTEO exposure on placental and fetal well being, we used high-frequency ultrasound to quantify changes in UA and MCA blood flow.
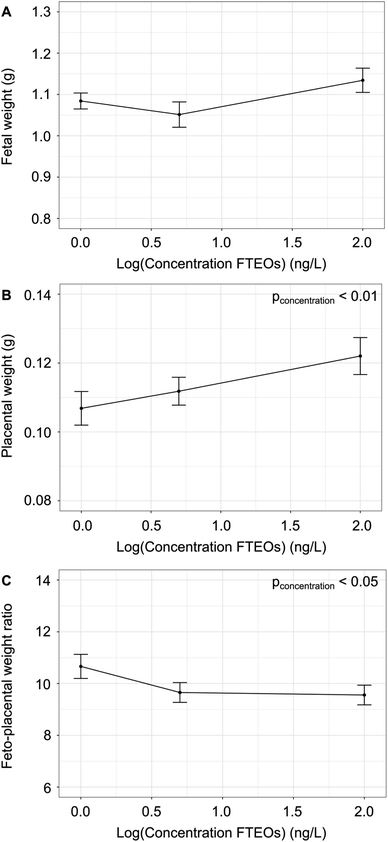 |
| Fig. 2 Developmental changes following maternal exposure to fluorotelomer ethoxylates. (A) There was no significant effect of the concentration of fluorotelomer ethoxylates (FTEOs) on the fetal weights. (B) Placental weights increased significantly with increasing concentration of FTEOs (p < 0.01, two-way ANOVA). (C) Feto-placental weight ratio decreased significantly with increasing concentration of FTEOs (p < 0.05, two-way ANOVA). n = 7–8 dams per group. Data are shown as means and 95% confidence intervals. | |
Ultrasound demonstrated further evidence of stress on the fetoplacental unit following exposure to FTEOs. The physiological and hemodynamic parameters are summarized in Table 1. There was a significant effect of concentration on the UA blood flow (p < 0.05) (Fig. 3a), with post hoc analysis showing a 31% decrease in the 5 ng L−1 FTEO group (p < 0.01, by Tukey post hoc test following linear mixed-effects model) and a 28% decrease in the 100 ng L−1 FTEO group compared to controls (p < 0.01, by Tukey post hoc test following linear mixed-effects model). There was no effect of concentration on the UA diameter, fetal heart rate, and UA PI. The decrease in placental blood flow following maternal exposure to FTEOs is consistent with placental insufficiency, deterioration of placental function that limits the amount of oxygen and nutrients being delivered to the fetus. Pregnancies complicated by placental insufficiency are at risk of hypoxic brain injury and fetal demise from asphyxia.30,31
Table 1 Physiological and hemodynamic parametersa
Parameter |
Control (0 ng L−1), n = 16 |
FTEO-exposed (5 ng L−1), n = 13 |
FTEO-exposed (100 ng L−1), n = 14 |
Data are presented as means and 95% confidence interval (CI) values. MCA, middle cerebral artery; PI, pulsatility index; UA, umbilical artery.
p < 0.01 compared to controls.
p < 0.01 compared to FTEO-exposed (5 ng L−1).
n = 11 controls (0 ng L−1), 8 FTEO-exposed (5 ng L−1), 14 FTEO-exposed (100 ng L−1).
|
UA diameter (mm) |
0.36 (CI: 0.34–0.38) |
0.36 (CI: 0.33–0.39) |
0.33 (CI: 0.31–0.35) |
Fetal heart rate (bpm) |
172 (CI: 149–195) |
155 (CI: 132–178) |
166 (CI:150–182) |
UA blood flow (mL min−1) |
0.13 (CI: 0.11–0.15) |
0.09 (CI: 0.07–0.11)b |
0.10 (CI: 0.08–0.12)b |
UA PI |
1.97 (CI: 1.89–2.05) |
2.0 (CI: 1.8–2.2) |
2.0 (CI: 1.9–2.1) |
MCA mean velocityd (mm s−1) |
13 (CI: 11–15) |
11 (CI: 4–18) |
6 (CI: 5–7)b |
MCA PId |
1.42 (CI: 1.33–1.51) |
1.6 (CI: 1.4–1.8) |
1.23 (CI: 1.14–1.32)b,c |
Cerebroplacental ratiod |
0.73 (CI: 0.68–0.78) |
0.74 (CI: 0.64–0.84) |
0.63 (CI: 0.58–0.68)b,c |
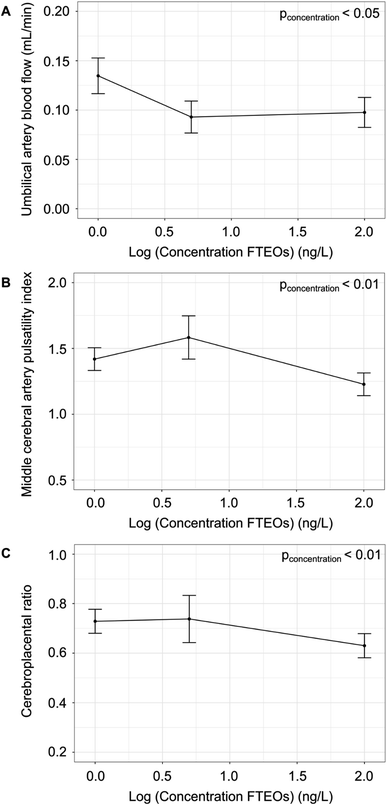 |
| Fig. 3 Impact of fluorotelomer ethoxylate exposure on placental and fetal hemodynamic responses at 17.5 days of gestation. (A) Umbilical artery blood flow was significantly decreased following exposure to fluorotelomer ethoxylates (FTEOs) (p < 0.05). (B) Middle cerebral artery pulsatility index was significantly decreased in the 100 ng L−1 FTEO exposure group compared to controls and 5 ng L−1 FTEOs (p < 0.01). (C) Cerebroplacental ratio was significantly decreased in the 100 ng L−1 FTEO exposure group compared to controls and 5 ng L−1 FTEOs (p < 0.01). n = 8–16 fetuses per group. Data are shown as means and 95% confidence intervals. | |
In response to hypoxia, there is physiological redistribution of the fetal circulation to preserve oxygenation of the fetal brain at the expense of other fetal organs. This phenomenon, the brain sparing effect, occurs in both humans32 and mice33,34 and is detected using the fetal MCA PI and the CPR.35 In this study, there was a significant effect of concentration on the MCA mean velocity (p < 0.05), MCA PI (p < 0.01) (Fig. 3b) and the CPR (p < 0.01) (Fig. 3c). Post hoc analysis showed a trend towards a higher MCA PI (p = 0.06) and no difference in the CPR in the 5 ng L−1 FTEOs group compared to controls. In contrast, the MCA PI was 15% lower in the 100 ng L−1 FTEOs group compared to controls (p < 0.01, by Tukey post hoc test following linear mixed-effects model) and the CPR was 14% lower in the 100 ng L−1 FTEOs group compared to controls (p < 0.01, by Tukey post hoc test following linear mixed-effects model). The MCA PI and CPR were significantly different between the two FTEO exposure groups (p < 0.05, by Tukey post hoc test following linear mixed-effects model). The significant decrease in the MCA PI indicates vasodilation of the cerebral circulation at the highest concentration of FTEOs, a fetal adaptation that is part of the brain sparing response to preserve oxygen delivery to the brain. Brain sparing is a hallmark of accelerating placental dysfunction,35 a known cause of adverse long-term neurodevelopmental outcomes.36
Taken together, maternal exposure to FTEOs causes reduced placental blood flow that initiates compensatory mechanisms to increase placental growth and to redistribute blood flow to the fetal brain to protect the fetus from hypoxic injury. The exposure does not significantly impact fetal growth in late gestation, suggesting the placental insufficiency is late onset. To confirm this hypothesis, future investigations will involve longitudinal studies of placental and fetal blood flow throughout gestation in mice. Moreover, it will be valuable to study the long-term neurological outcomes of FTEO exposure, since by term the placenta may have evoked all of its compensatory mechanisms and further fetal growth may result in detrimental outcomes.
In addition to the experimental approaches used here, several computational strategies have been developed to predict developmental toxicology.37–39 TIRESIA (Toxicology Intelligence and Regulatory Evaluations for Scientific and Industry Applications)40 and TISBE (TIRESIA Improved on Structure-Based Explainability)41 are eXplainable Artificial Intelligence platforms that uses state-of-the-art machine learning to predict developmental toxicity based on molecular features from existing toxicity data. TISBE predicted 6
:
2 FTEOs to be a developmental toxicant (classification score = 0.88), consistent with the experimental results presented in this study. As more experimental toxicity data from PFAS are incorporated into the training set, these non-animal-based approaches will have an important role to play in screening the over 12
000 replacement PFAS of interest.
The finding that maternal exposure to FTEOs during gestation causes adverse effects during pregnancy is consistent with several studies of legacy and replacement PFAS exposure. PFAS are endocrine disruptors and, while not measured in this study, maternal PFAS exposure in mice has been shown to alter fetal anogenital distance,42 a biomarker of reproductivity toxicity.43 Exposure to PFOA and hexafluoropropylene oxide dimer acid (a replacement PFAS known as GenX) in CD-1 mice resulted in an increase in placental weights and a reduction in the FPWR at GD17.5.16 Histopathological lesions were also found in the placenta including necrosis and atrophy of the placental labyrinth (the interface for gas and nutrient exchange), suggesting impaired transfer of nutrients and oxygen. Future investigations should use histopathology to determine the impact of FTEO exposure on placental structure. While the study by Blake et al. also reported a significant decrease in fetal weights,16 we did not observe any significant differences following FTEO exposure. The difference may be explained by the administered dose of the PFAS. Most of the studies exploring the impact of PFAS on pregnancy in mice used much higher doses than those in the present study (assuming a 50 g dam that drinks 8 mL of water per day, 0.25 mg day−1 PFOA or 0.5 mg day−1 GenX in Blake et al. vs. 8 × 10−7 mg day−1 in the present study). This is supported by a recent meta-analysis that reported PFOA and PFOS were only associated with lower fetal weights when the maternal dose was greater than 5 mg kg−1 day−1 and 10 mg kg−1 day−1 respectively.44 Similar high doses of PFOA and PFOS in CD-1 mice also resulted in a decrease in the number of live fetuses, reduced maternal weight gain, and birth defects including cleft palate, skeletal malformations, anasarca, and congenital heart defects.17,45–47 Although the concentrations in the present study are relatively low by comparison, they are similar to the newly proposed regulations put forth by the US EPA which recommend establishing legally enforceable limits for PFOA and PFOS at individual concentrations of 4 ng L−1 (4 parts per trillion) in drinking water.48 FTEOs have not yet been detected in finished drinking water, but they have been detected in wastewater as well as indoor dust, which suggests inhalation may be a route to exposure.
4. Conclusions
In summary, FTEOs, a new class of PFAS, were found to have a significant impact on placental and fetal health at environmentally relevant concentrations in mice. The observed decrease in umbilical artery blood flow and fetal hemodynamic distress may have serious long-term effects into adulthood. While the ability of FTEOs to bioaccumulate remains unknown, their toxicity demonstrated in this study raises significant concerns about their impact on human health. These findings have important implications for policy makers to inform new environmental regulations and guidelines on replacement PFAS such as FTEOs.
Abbreviations
CPR | Cerebroplacental ratio |
FPWR | Feto-placental weight ratio |
FTEOs | Fluorotelomer ethoxylates |
GD | Gestational day |
MCA | Middle cerebral artery |
PFAS | Poly- and perfluoroalkyl substances |
PFOA | Perfluorooctanoic acid |
PFOS | Perfluorooctane sulfonate |
PI | Pulsatility index |
UA | Umbilical artery |
Author contributions
Katherine L. Steeves: data curation, formal analysis, investigation, project administration, writing – original draft. Jenna Hanrahan: investigation, writing – review & editing. Nikita E. Harvey: investigation, writing – review & editing. Karl J. Jobst: conceptualization, project administration, writing – review & editing. Lindsay S. Cahill: conceptualization, funding acquisition, project administration, writing – review & editing.
Conflicts of interest
There are no conflicts to declare.
Acknowledgements
This work was supported by a Natural Sciences and Engineering Research Council (NSERC) Discovery Grant, the Canadian Foundation for Innovation (CFI), and the Newfoundland and Labrador Department of Industry, Energy and Technology.
References
- J. Glüge, M. Scheringer, I. T. Cousins, J. C. DeWitt, G. Goldenman, D. Herzke, R. Lohmann, C. A. Ng, X. Trier and Z. Wang, An overview of the uses of per- and polyfluoroalkyl substances (PFAS), Environ. Sci.: Processes Impacts, 2020, 22, 2345 RSC
.
- M. Land, C. A. de Wit, A. Bignert, I. T. Cousins, D. Herzke, J. H. Johansson and J. W. Martin, What is the effect of phasing out long-chain per- and polyfluoroalkyl substances on the concentration of perfluoroalkyl acids and their precursors in the environment? A systematic review, Environmental Evidence, 2018, 7, 4 CrossRef
.
- D. Melzer, N. Rice, M. H. Depledge, W. E. Henley and T. S. Galloway, Association between serum perfluorooctanoic acid (PFOA) and thyroid disease in the U.S. National Health and Nutrition Examination Survey, Environ. Health Perspect., 2010, 118, 686 CrossRef CAS PubMed
.
- V. Barry, A. Winquist and K. Steenland, Perfluorooctanoic acid (PFOA) exposures and incident cancers among adults living near a chemical plant, Environ. Health Perspect., 2013, 121, 1313 CrossRef PubMed
.
- K. Steenland, L. Zhao, A. Winquist and C. Parks, Ulcerative colitis and perfluorooctanoic acid (PFOA) in a highly exposed population of community residents and workers in the mid-Ohio valley, Environ. Health Perspect., 2013, 121, 900 CrossRef
.
- X. Zhang, R. Di Lorenzo, P. A. Helm, E. J. Reiner, P. H. Howard, D. C. G. Muir, J. G. Sled and K. J. Jobst, Compositional space: a guide for environmental chemists on the identification of persistent and bioaccumulative organics using mass spectrometry, Environ. Int., 2019, 132, 104808 CrossRef CAS
.
- EPA, PFAS master list of PFAS substances, Comptox Chemicals Dashboard, https://comptox.epa.gov/dashboard/chemical-lists/pfasmaster, accessed on July 22, 2023.
-
E. J. Reiner, K. J. Jobst, D. Megson, F. L. Dorman and J.-F. Focant, Chapter 3: Analytical methodology of POPs, in Environmental Forensics of POPs, ed. C. Sandau and G. O'Sullivan, Elsevier, 2013 Search PubMed
.
- A. MacNeil, X. Li, R. Amiri, D. C. G. Muir, A. Simpson, M. J. Simpson, F. L. Dorman and K. J. Jobst, Gas chromatography-(cyclic) ion mobility mass spectrometry: a novel platform for the discovery of unknown per-/polyfluoroalkyl substances, Anal. Chem., 2022, 94, 11096 CrossRef CAS PubMed
.
- K. L. Steeves, M. J. Bissram, S. Kleywegt, D. Stevens, F. L. Dorman, A. J. Simpson, M. J. Simpson, L. S. Cahill and K. J. Jobst, Nontargeted screening reveals fluorotelomer ethoxylates in indoor dust and industrial wastewater, Environ. Int., 2023, 171, 107634 CrossRef CAS
.
- Z. Aghaei, K. L. Steeves, K. J. Jobst and L. S. Cahill, The impact of perfluoroalkyl substances on pregnancy, birth outcomes, and offspring development: a review of data from mouse models, Biol. Reprod., 2022, 106, 397 CrossRef PubMed
.
- J. L. Reiner, S. F. Nakayama, A. D. Delinsky, J. P. Sanko, S. E. Fenton, A. B. Lindstrom and A. M. J. Strynar, Analysis of PFOA in dosed CD1 mice. Part 1. Methods development for the analysis of tissues and fluids from pregnant and lactating mice and their pups, Reprod. Toxicol., 2009, 27, 360 CrossRef CAS PubMed
.
- S. E. Fenton, J. L. Reiner, S. F. Nakayama, A. D. Delinsky, J. P. Stanko, E. P. Hines, S. S. White, A. B. Lindstrom, M. J. Strynar and S.-S. E. Petropoulou, Analysis of PFOA in dosed CD-1 mice. Part 2. Disposition of PFOA in tissues and fluids from pregnant and lactating mice and their pups, Reprod. Toxicol., 2009, 27, 365 CrossRef CAS PubMed
.
- J. L. Bartels, S. R. Fernandez, T. A. Aweda, A. Alford, G. F. Peaslee, J. R. Garbow and S. E. Lapi, Comparative uptake and biological distribution of [18F]-labeled C6 and C8 perfluorinated alkyl substances on pregnant mice via different routes of administration, Environ. Sci. Technol. Lett., 2020, 7, 665 CrossRef CAS
.
- W. Jiang, Y. Deng, Z. Song, Y. Xie, L. Gong, Y. Chen and H. Kuang, Gestational perfluorooctanoic acid exposure inhibits placental development by dysregulation of labyrinth vessels and uNK cells and apoptosis in mice, Front. Physiol., 2020, 11, 51 CrossRef PubMed
.
- B. E. Blake, H. A. Cope, S. M. Hall, R. D. Keys, B. W. Mahler, J. McCord, B. Scott, H. M. Stapleton, M. J. Strynar, S. A. Elmore and S. E. Fenton, Effects in CD-1 mice following gestational exposure to perfluorooctanoic acid (PFOA) or hexafluoropropylene oxide dimer acid (HFPO-DA or GenX), Environ. Health Perspect., 2020, 128, 27006 CrossRef CAS
.
- C. H. Suh, N. K. Cho, C. K. Lee, C. H. Lee, D. H. Kim, J. H. Kim, B. C. Son and J. T. Lee, Perfluorooctanoic acid-induced inhibition of placental prolactin-family hormone and fetal growth retardation in mice, Mol. Cell. Endocrinol., 2011, 337, 7 CrossRef CAS PubMed
.
- N. J. Herkert, C. D. Kassotis, S. Zhang, Y. Han, V. F. Pulikkal, M. Sun, P. L. Ferguson and H. M. Stapleton, Characterization of per- and polyfluorinated alkyl substances present in commercial anti-fog products and their in vitro adipogenic activity, Environ. Sci. Technol., 2022, 56, 1162 CrossRef CAS
.
- T. Frömel and T. P. Knepper, Fluorotelomer ethoxylates: sources of highly fluorinated environmental contaminants part I: biotransformation, Chemosphere, 2010, 80, 1387 CrossRef
.
- X. Trier, K. Granby and J. H. Christensen, Polyfluorinated surfactants (PFS) in paper and board coatings for food packaging, Environ. Sci. Pollut. Res. Int., 2011, 18, 1108 CrossRef CAS PubMed
.
- Y.-Q. Zhou, L. S. Cahill, M. D. Wong, M. Seed, C. K. Macgowan and J. G. Sled, Assessment of flow distribution in the mouse fetal circulation at late gestation by high-frequency Doppler ultrasound, Physiol. Genomics, 2014, 46, 602 CrossRef PubMed
.
- H. A. Kaboré, S. V. Duy, G. Munoz, L. Méité, M. Desrosiers, J. Liu, T. K. Sory and S. Sauvé, Worldwide drinking water occurrence and levels of newly-identified perfluoroalkyl and polyfluoroalkyl substances, Sci. Total Environ., 2018, 616–617, 1089 CrossRef
.
- X. C. Hu, D. Q. Andrews, A. B. Lindstrom, T. A. Bruton, L. A. Schaider, P. Grandjean, R. Lohmann, C. C. Carignan, A. Blum, S. A. Balan, C. P. Higgins and E. M. Sunderland, Detection of poly- and perfluoroalkyl substances (PFASs) in U.S. drinking water linked to industrial sites, military fire training areas, and wastewater treatment plants, Environ. Sci. Technol. Lett., 2016, 3, 344 CrossRef CAS PubMed
.
- M. L. Lindsey, Z. Kassiri, J. A. I. Virag, L. E. de Castro Brás and M. Scherrer-Crosbie, Guidelines for measuring cardiac physiology in mice, Am. J. Physiol.: Heart Circ. Physiol., 2018, 314, H733 CrossRef PubMed
.
- C. Ebbing, S. Rasmussen and T. Kiserud, Middle cerebral artery blood flow velocities and pulsatility index and the cerebroplacental pulsatility ratio: a longitudinal reference ranges and terms for serial measurements, Ultrasound in Obstetrics & Gynecology, 2007, 30, 287 Search PubMed
.
- M. S. Golub and C. A. Sobin, Statistical modeling with litter as a random effect in mixed models to manage “interlitter likeness”, Neurotoxicol. Teratol., 2020, 77, 106841 CrossRef CAS
.
- C. E. Hayward, S. Lean, C. P. Sibley, R. L. Jones, M. Wareing, S. L. Greenwood and M. R. Dilworth, Placental adaptation: what can we learn from birthweight: placental weight ratio?, Front. Physiol., 2016, 7, 28 Search PubMed
.
- J. A. Hutcheon, H. McNamara, R. W. Platt, A. Benjamin and M. S. Kramer, Placental weight for gestational age and adverse perinatal outcomes, Obstet. Gynecol., 2012, 119, 1251 CrossRef
.
- C. Haavaldsen, S. O. Samuelsen and A. Eskild, Fetal death and placental weight/birthweight ratio: a population study, Acta Obstet. Gynecol. Scand., 2013, 92, 583 CrossRef
.
- E. C. Mallard, S. Rees, M. Stringer, M. L. Cock and R. Harding, Effects of chronic placental insufficiency on brain development in fetal sheep, Pediatr. Res., 1998, 43, 262 CrossRef CAS PubMed
.
- R. Gagnon, Placental insufficiency and its consequences, Eur. J. Obstet. Gynecol. Reprod. Biol., 2003, 110, S99 CrossRef PubMed
.
- W. Pearce, Hypoxic regulation of the fetal cerebral circulation, J. Appl. Physiol., 2006, 100, 731 CrossRef CAS PubMed
.
- L. S. Cahill, Y.-Q. Zhou, M. Seed, C. K. Macgowan and J. G. Sled, Brain sparing in fetal mice: BOLD MRI and Doppler ultrasound show redistribution during hypoxia, J. Cereb. Blood Flow Metab., 2014, 34, 1082 CrossRef CAS PubMed
.
- L. S. Cahill, J. Hoggarth, J. P. Lerch, M. Seed, C. K. Macgowan and J. G. Sled, Fetal brain sparing in a mouse model of chronic maternal hypoxia, J. Cereb. Blood Flow Metab., 2019, 39, 1172 CrossRef PubMed
.
- J. Miller, S. Turan and A. A. Baschat, Fetal growth restriction, Seminars in Perinatology, 2008, 32, 274 CrossRef PubMed
.
- Y. Leitner, A. Fattal-Valevski, R. Geva, R. Eshel, H. Toledano-Alhadef, M. Rotstein, H. Bassan, B. Radianu, I. Bitchonsky, A. J. Jaffa and S. Harel, Neurodevelopmental outcome of children with intrauterine growth retardation: a longitudinal, 10-year prospective study, Journal of Child Neurology, 2007, 22, 580 CrossRef PubMed
.
- M. Marzo, S. Kulkarni, A. Manganaro, A. Roncaglioni, S. Wu, T. S. Barton-Maclaren, C. Lester and E. Benfenati, Integrating in silico models to enhance predictivity for developmental toxicity, Toxicology, 2016, 370, 127 CrossRef CAS PubMed
.
- Z. Lin and W.-C. Chou, Machine learning and artificial intelligence in toxicology sciences, Toxicol. Sci., 2022, 189, 7 CrossRef CAS
.
- M. V. Togo, F. Mastrolorito, A. Orfino, E. A. Graps, A. R. Tondo, C. D. Altomare, F. Ciriaco, D. Trisciuzzi, O. Nicolotti and N. Amoroso, Where developmental toxicity meets explainable artificial intelligence: state-of-the-art and perspectives, Expert Opin. Drug Metab. Toxicol., 2023, 23, 1 Search PubMed
.
- M. V. Togo, F. Mastrolorito, F. Ciriaco, D. Trisciuzzi, A. R. Tondo, N. Gambacorta, L. Bellantuono, A. Monaco, F. Leonetti, R. Bellotti, C. D. Altomare, N. Amoroso and O. Nicolotti, TIRESIA: an eXplainable artificial intelligence platform for predicting developmental toxicity, J. Chem. Inf. Model., 2023, 63, 56 CrossRef CAS PubMed
.
- F. Mastrolorito, M. V. Togo, N. Gambacorta, D. Trisciuzzi, V. Giannuzzi, F. Bonifazi, A. Liantonio, P. Imbrici, A. De Luca, C. D. Altomare, F. Ciriaco, N. Amoroso and O. Nicolotti, TISBE: a public web platform for the consensus-based explainable prediction of developmental toxicity, Chem. Res. Toxicol., 2024, 37, 323 Search PubMed
.
- S. Chang, J. L. Butenhoff, G. A. Parker, P. S. Coder, J. D. Zitzow, R. M. Krisko, J. A. Bjork, K. B. Wallace and J. G. Seed, Reproductive and developmental toxicity of potassium perfluorohexanesulfonate in CD-1 mice, Reprod. Toxicol., 2018, 78, 150 CrossRef CAS PubMed
.
- U.S. EPA, Reproductive toxicity risk assessment guidelines, Federal Reserve, 1996, 61, 56274 Search PubMed
.
- E. Negri, F. Metruccio, V. Guercio, L. Tosti, E. Benfenati, R. Bonzi, C. La Vecchia and A. Moretto, Exposure to PFOA and PFOS and fetal growth: a critical merging of toxicological and epidemiological data, Crit. Rev. Toxicol., 2017, 47, 482 CrossRef PubMed
.
- J. R. Thibodeaux, R. G. Hanson, J. M. Rogers, B. E. Grey, B. D. Barbee, J. H. Richards, J. L. Butenhoff, L. A. Stevenson and C. Lau, Exposure to perfluorooctane sulfonate during pregnancy in rat and mouse. I: maternal and prenatal evaluations, Toxicol. Sci., 2003, 74, 369 CrossRef CAS PubMed
.
- D. Yahia, C. Tsukuba, M. Yoshida, I. Sato and S. Tsuda, Neonatal death of mice treated with perfluorooctane sulfonate, J. Toxicol. Sci., 2008, 33, 219 CrossRef CAS PubMed
.
- C. K. Lee, S. G. Kang, J. T. Lee, S.-W. Lee, J. H. Kim, D. H. Kim, B. C. Son, K. H. Kim, C. H. Suh, S. Y. Kim and Y. B. Park, Effects of perfluorooctane sulfuric acid on placental PRL-family hormone production and fetal growth retardation in mice, Mol. Cell. Endocrinol., 2015, 401, 165 CrossRef CAS PubMed
.
- EPA, Per- and Polyfluoroalkyl Substances (PFAS): Proposed PFAS National Primary Drinking Water Regulation, https://www.epa.gov/sdwa/and-polyfluoroalkyl-substances-pfas, accessed on August 4, 2023.
|
This journal is © The Royal Society of Chemistry 2024 |