DOI:
10.1039/D4TC02135E
(Paper)
J. Mater. Chem. C, 2024,
12, 11752-11762
Asymmetric side-chain engineering of conjugated polymers with improved performance and stability in organic electrochemical transistors†
Received
24th May 2024
, Accepted 10th July 2024
First published on 11th July 2024
Abstract
This study rigorously investigated the impact of asymmetric side chain design on the performance of organic electrochemical transistors (OECTs). Hydrophilic and hydrophobic side chains were systematically introduced into poly(isoindigo-bithiophene)-based polymers, and the resulting symmetric and asymmetric side chain combinations were carefully compared. The objective was to enhance coplanarity, mobility, hydrophilicity, and stability to improve OECT device performance. While P(C,C) and P(O,O), with symmetric branched alkyl and oligoether side chains, exhibit effective OECT device functions, the asymmetric side-chain designed P(C,O), with branched alkyl and oligoether side chains, outperforms both symmetric counterparts. P(C,O) demonstrates OECT performance with a pronounced product of mobility and capacitance (μhC*) of 65.2 F cm−1 V−1 s−1, excellent hole mobility (μh) of 0.78 cm2 V−1 s−1, and fast response speed. Specifically, P(C,O) demonstrates superior stability while maintaining good capacitance. The performance retention of P(C,O) after consecutive ON/OFF cycles is 77.5%, which is much higher than 35.2% and 47.0% of P(C,C) and P(O,O). The asymmetric structure contributes to enhancing coplanarity and structural stability, resulting in improved crystalline properties after electrolyte swelling. This study presents a balanced and rigorous approach to balancing the performance and stability in OECTs through asymmetric side chain design.
1. Introduction
In recent years, conjugated polymers (CPs) have demonstrated their high potential for application in semiconducting devices. CPs can be synthesized using low-cost and low-temperature fabrication methods, and can be readily processed with ordinary solvents.1–3 The widespread applications of CPs are attributed to their remarkable electrical and optical properties, and various organic optoelectronic devices are designed using them.1,4 Devices have been broadly applied and categorized as follows: light-emitting diodes (OLEDs),5 field-effect transistors (OFETs),6–8 organic thermoelectrics (OTEs),9 solar cells (OPVs),10,11 optical memristors,12 electro-chemical transistors (OECTs),13,14etc. Among these devices, the mechanism of OECTs is particularly fascinating in bioelectronics. OECTs are fabricated using a three-electrode system, with CPs as the electron/hole channel in contact with an electrolyte layer. Electric field driven ions transport through the aqueous layer into the electrolyte interface, doping the polymer layer. In this process, doping with ions causes an increase in the charge carrier concentration in CPs, making charge transport occur easily in the CP channel. Therefore, a p-type accumulation mode OECT can transport holes with anion doping.13
With the development of OECTs, exploring new strategies to improve transistor performance has become a common goal. Although the research is progressing rapidly, the fundamental characteristics of OECTs remain a significant challenge. The CP layer must adapt to the electrolyte phase to facilitate the transfer of ionic-to-electronic signals, allowing ions to permeate and dope the polymer layer effectively. As a result, the hydrophilicity of the polymer becomes a critical factor in improving performance. Contemporary researchers are diligently striving to design CPs that can achieve high electron/hole mobility (μ), high volumetric capacitance (C*), short response time (τ), and good electrochemical stability. To compare the superiority of polymer materials, μC* is considered an indicator of OECT performance. Traditional organic semiconductor devices use poly(3,4-ethylenedioxythiophene)polystyrene sulfonate (PEDOT:PSS) as the channel material in n-type depletion mode devices. PEDOT:PSS has a good μC* value, which may be attributed to its hydrophilic structure and high conductivity, leading to excellent ionic penetration at the electrolyte interface.15 Precisely for this reason, an appropriate design of hydrophilic side chains is essential to OECTs.
In the operation of OECTs, some dopant ions are accommodated in the polymer, and additional water enters the polymer with hydrated ions. However, a degraded polymer film morphology induced by the hydrophilic properties also reduces device performance. The polymer layer's stability affects OECT device performance. Excessive doping and electrolyte swelling induced by the hydrated ions may force the polymer films to swell and destroy the semiconducting channels.16,17 Nevertheless, designing hydrophilic side chains can efficiently enhance ion permeation and result in high μC*.18–20 Using CP blends and ionic backbones are also good strategies for hydrophilic CP design.21 Based on the above, designing CPs with sufficient hydrophilicity and morphological stability is effective in striking a balance between the device performance and operational stability.
Due to the diversified and modified ability of CP structures, recently, a nascent approach of asymmetric side-chain engineering has been applied to improve the OFET performance of CPs. To enhance the charge-transport performance of CPs, the design of high-coplanarity backbones is required. Asymmetric side chains enhance the electrical properties of CPs by mitigating steric effects and facilitating a bimodal orientation.6,9,21,22 Additionally, the asymmetric design of linear/branched alkyl side chains can improve the mechanical properties while preserving the majority of the electrical properties even after stretching.23,24 While the abovementioned methods can be effective in OFETs, there has been no study on applying asymmetric side-chain engineering in OECTs.
This study aims to balance mobility and stability using an asymmetric side chain design with a pair of hydrophilic and hydrophobic side chains. Appropriate hydrophilicity of the polymer layer can easily facilitate ionic doping, a fast response time, and high charge carrier concentration. Poly(isindigo-alt-bithiophene) (PII2T) was chosen as the polymer backbone; it is a common donor–accepter CP material with good charge-transport performance. The side chains were categorized into two types: hydrophobic octyl-dodecane (C) and hydrophilic oligoether (O). CPs with symmetric hydrophobic/hydrophilic side chain combinations were designated as P(C,C) and P(O,O), while the CP with an asymmetric side chain combination was named P(C,O). The chemical structures of the polymers are shown in Fig. 1a. The increase in polymer hydrophilicity accompanies the reduction of film stability. Therefore, the asymmetric combination of hydrophilic/hydrophobic side chains has the potential to strike a balance between the device performance and operational stability. To gain insight into the side-chain effect on the properties of CPs, the optical/electrochemical properties of the CPs were first investigated. Subsequently, the morphology and device characteristics were investigated to corroborate their structure–morphology–performance relationship.
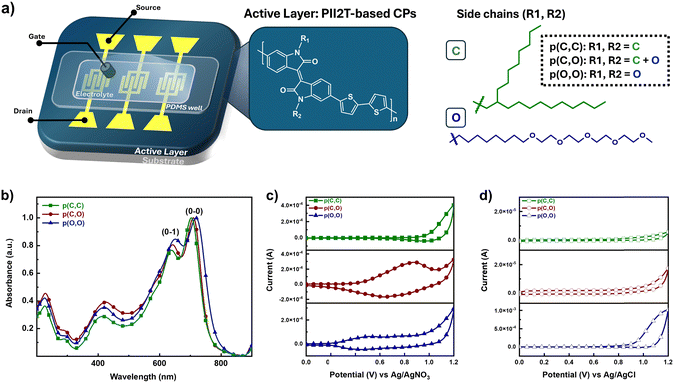 |
| Fig. 1 (a) Device architecture of a top-gate/bottom-contact OECT with PII2T-based polymers as an active layer. The chemical structures of the constituent polymers are indicated on the right side. (b) UV-vis absorption spectra of the polymer films. CV profiles of the polymer films in (c) an organic electrolyte of 0.1 M TBAP in acetonitrile or (d) an aqueous electrolyte of 0.1 M KCl(aq). Note that the CV measurements were conducted at a sweeping rate of 0.1 V s−1. | |
2. Experimental section
2.1 Materials
2-(Tributylstannyl)thiophene, 2-bromothiophene, 6-bromooxindole, 6-bromoisatin, tosylate chloride (TsCl), N-bromosuccinimide (NBS), and tetrabutylammonium perchlorate (TBAP) were purchased from Tokyo Chemical Industry Co., Ltd. 2,5,8,11-Tetraoxatridecan-13-ol, 8-bromo-1-octanol, 2-octylundecan-1-ol, and acetonitrile were purchased from Thermo Fisher Scientific Inc. 5,5′-Bis(trimethylstannyl)-2,2′-bithiophene (2T-tin) was purchased from Luminescence Technology Corp. Potassium carbonate, tris(dibenzylideneacetone)dipalladium(0) (Pd2(dba)3), acetic acid, sodium hydride, tetrahydrofuran (THF), dimethylformamide (DMF), ethyl acetate (EtOAc), anhydrous chlorobenzene (≥99%), anhydrous chloroform, pyridine, and tri(o-tolyl)phosphine (P(o-tolyl)3) were ordered from Sigma-Aldrich. These chemicals were used as received without further purification unless otherwise stated. Polymer P(C,C) was prepared according to a previous report25 (molecular weights evaluated by GPC: Mn = 84 kg mol−1, as shown in Fig. S1a, ESI†). P(O,O) was prepared according to previous reports12,26 (molecular weights evaluated by GPC: Mn = 66 kg mol−1, as shown in Fig. S1c, ESI†). The synthetic procedure for P(C,O) is described in the Experimental section, and the synthetic route is summarized in Scheme S1 (ESI†).
2.2 Synthesis of (E)-6,6′-dibromo-[3,3′-biindolinylidene]-2,2′-dione (Br-isoindogo)
6-Bromooxindole (1.09 g, 5.10 mmol) and 6-bromoisatin (1.13 g, 5.02 mmol) were placed into a 100 mL two-necked flask in which nitrogen was purged with a condenser in a 100 °C oil bath. Then, acetic acid (40 mL) and hydrochloric acid (0.25 mL) were added to the flask, and the mixture was stirred for 24 hours. After the reaction, the crude products were preliminarily purified by precipitation using deionized water (DI water). The solid mixture was then filtered and washed with ethanol. The red solid of Br-isoindogo (2.052 g, 97.3%) was collected from the filter cake after drying by evaporation overnight. 1H NMR (400 MHz, DMSO-d6, δ ppm, 25 °C, Fig. S2, ESI†): 9.00 (d, J = 8.64 Hz, 2H), 7.18 (dd, J = 6.68, 10.6 Hz, 2H), 6.99 (d, J = 1.84 Hz, 2H), 11.08 (br, 1H).
2.3 Synthesis of 2,5,8,11-tetraoxatridecan-13-yl 4-methylbenzenesulfonate (TEG-OTs)
2,5,8,11-Tetraoxatridecan-13-ol (1.95 g, 9.37 mmol) and anhydrous chloroform (10 mL) were placed into a 100 mL two-necked flask, and the system was cooled down to −20 °C. TsCl (1.77 g, 9.30 mmol, in 3.12 mL anhydrous pyridine) was added dropwise through a syringe into the mixture. The mixture was kept at −20 °C, and reacted for 5 h. After the reaction, rotary evaporation removed the crude products from the solvent. Subsequently, water (30 mL) was added, and the mixture was extracted with EtOAc (3 × 50 mL). The organic layer was washed with 1 N HClaq and sodium bicarbonate, and the organic layers were combined, dried over magnesium sulfate, filtered, and rotary-evaporated. The product was monitored with TLC and purified using column chromatography with EtOAc as the eluent. The product was then rotary-evaporated to obtain TEG-OTs as a colorless oil (1.772 g, 52.2%). 1H NMR (500 MHz, CDCl3, δ ppm, 25 °C, Fig. S3, ESI†): 7.77 (d, J = 8.25 Hz, 2H), 7.32 (d, J = 8.15 Hz, 2H), 4.13 (t, J = 9.70 Hz, 2H), 3.66 (t, J = 9.7 Hz, 2H), 3.61 (t, J = 9.35 Hz, 6H), 3.56 (s, 4H), 3.52 (q, J = 2, 9.25 Hz, 2H), 3.35 (s, 3H), 2.42 (s, 3H).
2.4 Synthesis of the side chain 22-bromo-2,5,8,11,14-pentaoxadocosane (TEG-C8-Br)
Sodium hydride (60% in mineral oil) (0.17 g, 7 mmol) was first placed into a 100 mL two-necked flask and washed with hexanes (70 mL × 3) to remove the outer mineral oil. Anhydrous THF (2.5 mL) was injected into the washed sodium hydride under nitrogen protection. 8-Bromo-1-octanol (1.62 g, 7.34 mmol) in anhydrous THF (2.5 mL) was added dropwise through a syringe into the mixture and stirred at 0 °C for 1 h until it mixed well. TEG-OTs (1.77 g, 4.89 mmol) in THF (2.5 mL) were added through a syringe and reacted at 0 °C under a nitrogen atmosphere. The mixture was then warmed to room temperature and stirred overnight. The solution was quenched by methanol and was then rotary-evaporated to remove the solvent. The mixture was subsequently extracted with EtOAc (3 × 50 mL). Then, the organic layer was washed with 1 N HClaq and sodium bicarbonate. The organic layers were combined, dried over magnesium sulfate, filtered, and rotary-evaporated sequentially. Finally, the crude products were purified using chromatography using hexane and EtOAc (1
:
3) as an eluent to obtain TEG-C8-Br as the white solid (1.08 g, 55.1%). 1H NMR (500 MHz, CDCl3, δ ppm, 25 °C, Fig. S4, ESI†): 3.72 (t, J = 11.15 Hz, 1H), 3.69–3.57 (m, 18H), 3.57–3.48 (m, 5H), 3.46–3.27 (m, 10H), 1.82 (t, J = 14.45 Hz, 2H), 1.53 (d, J = 6 Hz, 4H), 1.46–1.35 (br, 2H), 1.35–1.20 (br, 10H).
2.5 Synthesis of the side chain 9-(bromomethyl)octadecane (OD-Br)
2-Octylundecan-1-ol (2.99 g, 10 mmol), triphenylphosphine (3.93 g, 15 mmol), and dichloromethane (DCM) (50 mL) were placed into a 100 mL flask. The mixture was stirred under nitrogen protection at 0 °C. NBS (2.67 g, 15 mmol) was added in portions to the mixture. The reaction was conducted for 16 h. Subsequently, the solvent was removed by rotary evaporation. Then, the crude products were purified using column chromatography with hexane as an eluent. The mixture was then rotary-evaporated to remove hexane, and OD-Br was obtained as a colorless oil (2.96 g, 82.1%). 1H NMR (500 MHz, CDCl3, δ ppm, 25 °C, Fig. S5, ESI†): 3.47 (d, J = 5.95 Hz, 1H), 1.59 (br, 2H), 1.40–1.23 (br, 19H), 0.90 (t, 17 Hz, 4H).
2.6 Synthesis of (E)-6,6′-dibromo-1-(2,5,8,11,14-pentaoxadocosan-22-yl)-1′-(2-octylundecyl)-[3,3′-biindolinylidene]-2,2′-dione, M(C,O)
Br-isoindogo (0.70 g, 1.67 mmol), potassium carbonate (0.69 g, 4.99 mmol), and DMF (10 mL) were placed into a 100 mL two-necked flask under nitrogen protection. The mixture was stirred at 60 °C for 1 h until it mixed well. The mixture of TEG-C8-Br (0.83 g, 2.08 mmol) and OD-Br (0.75 g, 2.08 mmol) was added dropwise through a syringe into the Br-isoindogo mixture. Subsequently, the mixture was stirred overnight at 100 °C under nitrogen protection. After the reaction, the crude products were removed from the solvent by rotary evaporation and washed with DCM. Then, water was added (50 mL), and the mixture was extracted with DCM (3 × 50 mL). The combined organic layer was washed with 1 N HClaq, dried over magnesium sulfate, filtered, and rotary-evaporated. The product was monitored with TLC and purified by chromatography using hexane and DCM (1
:
1) as an eluent. The product was then rotary-evaporated to obtain M(C,O) as a red solid (0.42 g, 19.8%). 1H NMR (500 MHz, CDCl3, δ ppm, 25 °C, Fig. S6, ESI†): 4.11 (q, J = 7.0, 21.35 Hz, 3H), 4.06 (t, J = 13.4 Hz, 4H), 3.75 (t, J = 11.9 Hz, 2H), 3.69–3.60 (m, 35H), 3.58–3.51 (m, 12H), 3.46–3.35 (m, 20H), 2.09 (s, 2H), 2.04 (s, 10H), 1.84 (qn, J = 15, 28.7 Hz, 3H), 1.79–1.47 (m, 16H), 1.45–1.08 (m, 44H), 0.93 (t, J = 14.75 Hz, 6H).
2.7 Polymerization of P(C,O)
The monomer M(C,O) (180.3 mg, 0.177 mmol), 5,5′-bis(trimethylstannyl)-2,2′-bithiophene (2T-tin) (87 mg, 0.177 mmol), and chlorobenzene (0.885 mL) were placed in a 50 mL two-necked flask with a stir bar and a condenser. The mixture was bubbled with nitrogen for 1 h. Pd2(dba)3 (9.72 mg, 0.106 mmol) and P(o-tolyl)3 (10.77 mg, 0.035 mmol) were subsequently added to the mixture and the polymerization was conducted at 130 °C for 24 h under nitrogen protection. After polymerization, the end-capping agents 2-bromothiophene (9 μL) and 2-tributylstannylthiophene (29 μL) were sequentially added to the mixture at 110 °C for 2 h for each end-capping reaction. After cooling to room temperature, the solution was precipitated by pouring it into methanol. The crude polymer was then purified by Soxhlet extraction using methanol, acetone, hexane, and chloroform (24 h for each solvent). The crude polymer was evaporated and poured into methanol to precipitate the polymer product. After filtration and drying under vacuum, the target polymer P(C,O) was obtained. 1H NMR (500 MHz, CDCl3, δ ppm, 25 °C, Fig. S7, ESI†): 8.74 (br, 8H), 8.09 (s, 1H), 7.69 (s, 2H), 7.52 (s, 2H), 7.47–5.16 (br, 32H), 4.32–4.14 (m, 4H), 3.95–3.42 (br, 207 H), 3.42–3.22 (br, 35H), 2.23–1.50 (br, 138H), 1.49–1.14 (br, 159H), 1.13–0.43 (br, 76 H). 13C NMR (100 MHz, CDCl3, δ ppm, 25 °C, Fig. S8, ESI†): 11.06, 14.08, 14.35, 19.82, 22.60, 26.07, 26.35, 29.34, 29.63, 31.17, 31.90, 32.15, 33.50, 37.09, 58.99, 68.54, 70.56, 71.90, 76.74, 76.99, 77.25, 128.77, 129.46, 130.85, 132.44, 167.72. Anal. Calcd for C63H92N2O7S2 (%): C, 41.61; H, 4.07; N, 8.09; S, 18.50. Found (%): C, 41.65; H, 3.98; N, 8.06; S, 18.60. Molecular weight evaluated by GPC: Mn = 68 kg mol−1, Mw = 174 kg mol−1, PDI = 2.56, as shown in Fig. S1b (ESI†).
2.8 Characterization
1H and 13C NMR spectra were recorded using a Bruker AVNEO500 at 25 °C with working frequencies of 500 MHz for 1H and 100 MHz for 13C. Gel permeation chromatography (GPC) was performed in tetrahydrofuran (THF) at 40 °C with a LC-20AT (Shodex GPC LF-604, inter diameter: 6 mm, length: 150 mm, packing material: styrene-divinylbenzene) using THF as an eluent at a flowing rate of 0.3 mL min−1 at 40 °C. The results were obtained based on the calibration performed using styrene-divinylbenzene standards. UV-vis absorption spectra were recorded on a Jasco V-770 (200–900 nm). Cyclic voltammetry (CV) was carried out using a CHI 6273E to characterize the electrochemical properties in a three-electrode system, with polymer-coated indium tin oxide (ITO) glass, a Pt rod, Ag/AgCl as a working electrode, a counter electrode, and a reference electrode, respectively. Organic phase measurement was carried out in an electrolyte of 0.1 M tetrabutylammonium perchlorate (TBAP) in anhydrous ACN, and aqueous phase measurement was carried out in an electrolyte of 0.1 M KCl(aq). To obtain the in situ electrochemical-optical spectra, CHI 6273E was employed in an aqueous phase apparatus to establish a three-electrode system, and UV-vis absorption spectra were recorded using Jasco V-770 (300–1100 nm), simultaneously. Grazing-incidence X-ray diffraction (GIXD) measurements were carried out on the beamline 13A1 in the National Synchrotron Radiation Research Center (NSRRC), Taiwan. The applied wavelength of X-ray was 1.023 Å with an incident angle of 0.12°. For all the thin film spectral characterization, including UV-vis absorption spectroscopy, CV measurement, electrochemical-optical spectroscopy, and GIXD, polymer solution (6.3 mg mL−1 in chloroform) was spin-coated onto the substrate to prepare the thin film.
2.9 Fabrication and measurement of OECT devices
A top-gate/bottom-contact (TG/BC) architecture was applied to fabricate an OECT device. The polymers P(C,C), P(C,O), and P(O,O) served as the channel layer of the device. First, 25-nm-thick Cr and 40-nm-thick Au were deposited on the glass substrate through a shadow mask, which served as the source/drain electrodes. The width (W) and length (L) of the interdigitated electrode pattern were 9000 (consisting of 9 parallel channels, each with 1000 μm) and 30 μm, respectively. Second, polymer solutions (6.3 mg mL−1 in chloroform), preheated at 60 °C, were spin-coated at 1200 rpm for 60 s (acceleration: 500 rpm) to deposit onto the substrate with patterned Au/Cr electrodes. Third, poly(dimethylsiloxane) (PDMS) synthesized using a 3D printer was adhered to the polymer film. Finally, 0.1 M KCl(aq) was injected into the PDMS well, in which an Ag/AgCl electrode was in contact with the electrolyte, and a pair of Ag wires were in contact with the source/drain electrodes. All the characteristics were measured and analyzed using a Keithley 4200-SCS. The dual-sweep transfer curves of the OECT were measured in the gate voltage (VG) sweep range from 0.1 to −1.0 V and with a fixed drain voltage (VD) of 0.3 V. Note that all the mobilities (μh) of the polymer films were calculated from their transfer curves. Output curves were obtained by ranging VD from 0.1 to −0.35 V and keeping VG fixed from 0 to 0.9 V, respectively. Finally, the transient curves using VG = −0.85 V as the gate bias were obtained every 20 s to analyze the rise time (tr), fall time (tf), and stability properties. The volumetric capacitance (C*) of the film was measured using a three-electrode system with CHI 6273E from 0.1 Hz to 10 kHz. The film thickness of P(C,C), P(C,O), and P(O,O) was controlled at 39 ± 2 nm, 42 ± 6 nm, and 45 ± 4 nm, respectively. The thickness was determined using a surface profilometer of Alpha-Step D300.
3. Results and discussion
3.1 Polymer film characterization
To investigate the effects of asymmetric side-chain design on the performance of OECTs, oligoether and branched alkyl side chains were introduced into PII2T-based CPs. This experimental strategy aims to enhance the coplanarity and charge-transport performance of CPs. The polymer films were applied in top-gate/bottom-contact (TG/BC) OECT devices. The device architecture is displayed in Fig. 1a. Moreover, the oligoether side chain provides good wettability in aqueous electrolytes, meeting the hydrophilicity requirements for OECT fabrication and the hydrophilicity of PII2T-based CPs can be adjusted based on varying side chain content. For a better understanding of its hydrophilicity, CPs were coated onto glass. Fig. S9 (ESI†) displays the contact angles of 105.2°, 82.0°, and 65.4° for P(C,C), P(C,O), and P(O,O), respectively. The asymmetric side chains were uniformly introduced into the polymer in P(C,O), resulting in a contact angle that precisely falls between its symmetric counterparts of P(C,C) and P(O,O). This phenomenon suggests an enhanced hydrophilicity of the film due to the hydrophilic side chains, and it is anticipated that its ion-doping capability will also be enhanced.16,19,22 Additionally, the asymmetric side-chain design introduced uniform hydrophilic side chains into the polymer film, thereby mitigating localized heterogeneous or phase separation issues. In addition, the molecular weight of the polymer was controlled within the range of 66–84 kg mol−1. Therefore, the potential influence of CP's molecular weight on its morphology and device performance can be precluded.
In optical analysis, Fig. 1b presents the UV-vis absorption spectra of the polymer thin film. The absorption band between 350 and 450 nm is associated with the π–π* transition. In comparison, the two vibrational peaks (0–0 and 0–1) observed between 520 and 775 nm represented intramolecular charge transfer (ICT) between the constituent donor and acceptor moieties. Accordingly, the positions of absorption maxima (λmax) are 703, 710, and 718 nm for P(C,C), P(C,O), and P(O,O), respectively. The maximal absorption peaks of P(C,O) and P(O,O) are located at similar positions, and are more red-shifted than that of P(C,C). In addition, the values of the (0–1)/(0–0) ratio are 0.77, 0.81, and 0.85 for P(C,C), P(C,O), and P(O,O), respectively, as shown in Table S1 (ESI†). A low (0–1)/(0–0) ratio indicates an enhanced aggregation. The hydrophobic branched alkyl side chains contribute to polymer aggregation. In contrast, hydrophilic side chains cause polymer segregation.19 Note that the aggregation performance of P(C,O) falls between that of P(C,C) and P(O,O), and this trend indicates the good compatibility of asymmetric side-chain combinations. Besides the absorption profiles, the optical band gap (Eg) estimated from the spectral onset is similar around 1.64, 1.61, and 1.58 eV for P(C,C), P(C,O), and P(O,O), respectively.
Their cyclic voltammetry (CV) profiles in a thin-film state were obtained to investigate these polymers’ electrochemical behavior further. Fig. 1c and d display the measurements performed in organic- (0.1 M TBAP in acetonitrile) and aqueous-phase (0.1 M KCl(aq)) electrolytes, respectively. All of the optical and electrochemical parameters are summarized in Table S1 (ESI†). The oxidative onset potential was derived from the organic-phase measurement to calculate the highest occupied molecular orbital (HOMO) levels of the polymer films. The HOMO levels are −5.52, −5.31, and −5.08 eV for P(C,C), P(C,O), and P(O,O) respectively. Then, the lowest unoccupied molecular orbital (LUMO) level was calculated based on: LUMO = HOMO + Eg.19 The LUMO levels are −3.88, −3.70, and −3.50 eV for P(C,C), P(C,O), and P(O,O) respectively. The frontier energy levels of the polymers are summarized in Fig. S10 (ESI†). Even though the polymers share the same PII2T backbone, it can be observed that the HOMO/LUMO levels increase along with the contents of oligoether side chains.27,28
Moreover, the oxidative onset potential difference (ΔEonset) between the organic and aqueous phases indicates the underlying ion-doping resistance. P(C,C) with symmetric hydrophobic side chains exhibits a significantly higher oxidative onset potential (>1.0–1.2 V) in the aqueous phase, thereby hindering the prediction of ΔEonset. This behavior indicates the difficulty of ion doping in P(C,C). This relatively high oxidative onset potential in an aqueous system is also related to the fast scanning speed of 0.1 V s−1. In contrast, the ΔEonset values of P(C,O) and P(O,O) are 0.61 and 0.70 V. P(C,O) has a lower ΔEonset than P(O,O), representing its facile doping behavior with a lower energy barrier, thereby indicating that it is a more appropriate polymer in OECT operation.
3.2
In situ electrochemical-optical spectroscopy studies
After investigating the independent optical and electrochemical properties, in situ electrochemical-optical spectroscopy measurement was employed to understand the polymer's doping behavior further. The polymer film was scanned from 0 to 1.2 V and simultaneously measured the optical absorption spectra. Fig. 2a–c display the optical absorption spectra of P(C,C), P(C,O), and P(O,O), respectively. As a result, the absorption peak of ICT (600–800 nm) decreases, while the absorption peak of the polaron (800–1200 nm) increases. As can be seen, Fig. 2d–f illustrate the decrease in the (0–0) absorption peak and the increase in the polaron absorption peak as the applied potential increases. It can be observed that P(C,C) exhibits poor electrochemical doping behavior up to 1.1 V, with only slight doping at 1.2 V. In contrast, P(C,O) and P(O,O) exhibit strong electrochemical doping behaviors after 0.7 V, which can be attributed to the hydrophilic side chains enabling facile ion penetration and uptake. Notably, P(O,O) demonstrates poorer stability than P(C,O), and the optical properties of P(O,O) rapidly decreased after the potential sweeping. This behavior may indicate P(O,O)'s poor electrochemical stability during OECT device operation. Therefore, the asymmetric side-chain design contributes to the improved stability of P(C,O).
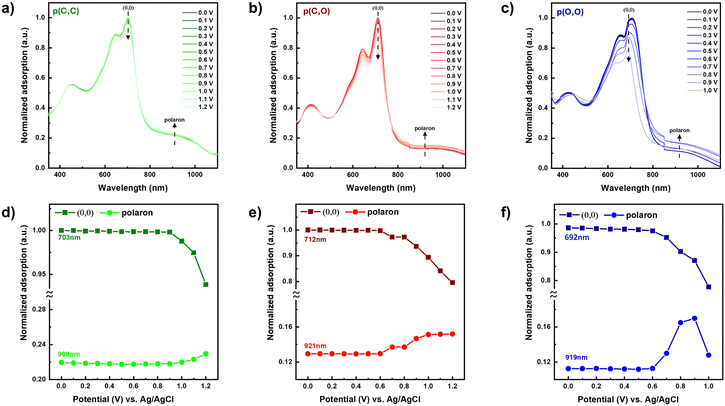 |
| Fig. 2 (a)–(c) In situ electrochemical-optical spectra and (d)–(f) the optical absorbance changes in the characteristic peaks of (a) and (d) P(C,C), (b) and (e) P(C,O), and (c) and (f) P(O,O) films deposited on an ITO glass. | |
3.3 Polymer film morphologies
After analyzing the effects of hydrophilic side chains on electrochemical doping, the morphology of the polymer films was investigated. To understand the impact of side-chain arrangement and electrolyte penetration on morphology changes, grazing-incidence X-ray diffraction (GIXD) analysis was carried out to characterize the polymer films. The 2D GIXD patterns of the as-cast polymer film are shown in Fig. 3a. Furthermore, the 2D GIXD patterns of the electrolyte-swelled polymer films soaked in KCl(aq) for 7 hours are shown in Fig. 3b. The corresponding 1D scanning profiles along the out-of-plane (OOP) and in-plane (IP) directions are presented in Fig. 3c and d, and the pole figure based on (100) diffractions is shown in Fig. S11 (ESI†). The crystallographic properties calculated from these data are displayed in Tables S2 and S3 (ESI†), including the lamella spacing (d), crystallite coherence length (Lc), paracrystalline disorder (g), and relative degree of crystallinity (rDOC). Originally, the polymers exhibited a typical edge-on orientation, indicated by their intense (n00) and (010) characteristics. First, the OOP lamellar spacing (d200) values calculated from the (200) diffraction for P(C,C), P(C,O), and P(O,O) are 12.04, 12.91, and 11.61 Å, respectively. The IP π–π spacing (d010) values for P(C,O) and P(O,O) are 3.55 and 3.65 Å. Notably, P(C,O) exhibits a better edge-on stacking with larger d200 and closer d010 than P(C,C) and P(O,O). This is a synergistic effect of asymmetric side-chain design in mitigating the steric hindrance of side chains imposed on the backbone.
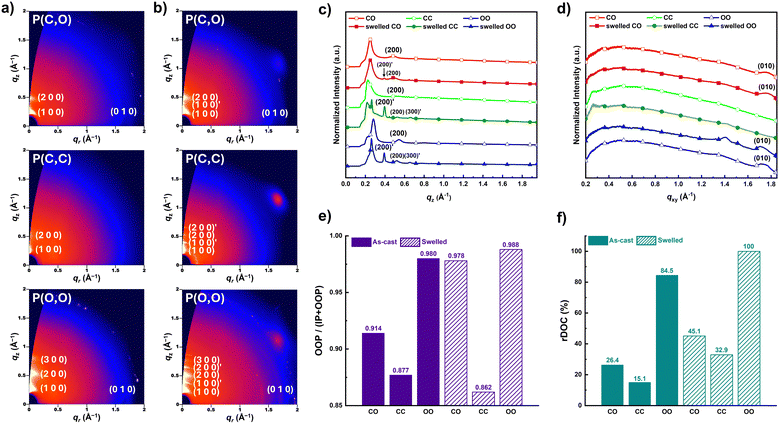 |
| Fig. 3 2D GIXD patterns of the (a) as-cast and (b) electrolyte-swelled polymer films. 1D GIXD line-cutting profiles along the (c) OOP and (d) IP directions. Note that the open/solid symbols indicate the as-cast and electrolyte-swelled polymer films. Summary of the (e) orientational populations and (f) rDOC of the as-cast and electrolyte-swelled polymer films. | |
After swelling by the electrolyte, the d200 values for P(C,C), P(C,O), and P(O,O) became 12.09, 12.91, and 12.09 Å, respectively. The d010 values of the swelled films for P(C,O) and P(O,O) are 3.55 Å and 3.67 Å. Interestingly, P(C,C) has no π–π spacing signal in the as-cast and swelled films, showing its irregular lamellar spacing induced by the steric hindrance of the branched alkyl side chains. As can be seen, P(C,O) and P(C,C) showed less changed spacings than P(O,O), indicating their stable crystalline structures. It is worth noting that P(C,C) and P(O,O) exhibit another couple of lamellar diffraction signal, designated as (200)′ in Fig. 3b, after soaking in the electrolyte, suggesting that the surface structural arrangement becomes more compact after swelling.16,18 However, the (200)′ signal of swelled P(C,O) was subtle, suggesting that asymmetric side chains are more stable under film swelling. In addition, P(C,O) demonstrates the largest d200 due to its ordered structural arrangement and orientation in the OOP direction. Asymmetric side-chain design helps increase the polymer coplanarity and improve the polymer's resistance to electrolyte swelling.
With regard to the structural order and orientations in the crystalline domains before/after electrolyte swelling, the changes in polymer orientational populations (face-on vs. edge-on) between the as-cast and swelled state were calculated and are shown in Fig. 3e. The changes in rDOC are shown in Fig. 3f. In the OOP direction, the values of Lc,200 (Å) and g200 (%) for the as-cast P(C,C), P(C,O), and P(O,O) are (63, 18.3), (90, 15.9), and (105, 14.0), respectively. The Lc,200 (Å) and g200 (%) values for the swelled P(C,C), P(C,O), and P(O,O) are (62, 18.6), (84, 16.5), and (140, 12.3), respectively. A larger Lc indicates a more uniform crystalline arrangement, while a larger g suggests a more disordered arrangement; these two properties typically exhibit opposite trends. After swelling, P(C,C) becomes more segregated, whereas P(C,O) and P(O,O) exhibit improved crystallinity. Fig. S12a (ESI†) clearly shows that P(O,O) exhibits an ordered structure, and further film swelling enhances the P(O,O)'s ordered structure even better. Due to the strong swelling caused by the hydrophilic side chains, the larger film volume provides sufficient space for rearranging structures to improve the structural order and promote the edge-on population. In the IP direction, the Lc,010 (Å) and g010 (%) values for P(C,O) and P(O,O) are (64, 9.9) and (54, 10.9), and they became (62, 10.0) and (58, 10.6) after electrolyte swelling. As shown in Fig. S12b (ESI†), P(C,O) and P(O,O) exhibit a similar trend in the OOP direction. Introducing hydrophilic side chains can appropriately and significantly promote the edge-on orientation and structural order through swelling. The pole figure can support this point, as shown in Fig. S11 (ESI†).
With regard to the crystallinity variations of the polymer films before/after electrolyte swelling, Table S4 (ESI†) presents the rDOC values calculated from azimuthal integrations of P(C,C), P(C,O), and P(O,O). The rDOC calculation was normalized based on the electrolyte-swelled P(O,O). The rDOC values are 15.1%, 26.4%, and 84.5% for P(C,C), P(C,O), and P(O,O), respectively. After swelling, the rDOC values increase to 32.9%, 45.1%, and 100%. P(C,C) showed low rDOC values before/after electrolyte swelling. In contrast, P(O,O) exhibits the highest crystallinity among the polymers studied, followed by P(C,O). To maintain the crystalline morphology and stability of the film, asymmetric side chain designs are necessary. The P(C,O) film exhibits the most significantly improved crystalline properties after swelling. While it has been previously mentioned that stronger swelling can provide more space for polymer rearrangement, the P(C,O) film shows the greatest increase in rDOC. This disparity is because excessive swelling can degrade the crystalline structure of the film. However, the asymmetric structure of P(C,O) contributes to a superior structural arrangement, making the film less susceptible to electrolyte swelling and thereby enhancing its ability to rearrange. As a result, P(C,O) outperforms P(O,O) regarding rearrangement capability. The analysis of OECT device stability further supports this perspective.
3.4 OECT device characteristics
After investigating the electrochemical-optical spectra and thin-film morphologies, these CP films were applied to OECT devices (Fig. 1a). Fig. 4a–c present the transfer characteristics of the polymers studied. Table 1 summarizes the device parameters. The OECT device parameters were calculated based on the TG/BC device configuration, and the PII2T-based OECTs exhibit typical p-type behavior. The transfer characteristics are typical for Type II doping processes that occur when the polymer film is doped beyond the initial stage, which needs to be refilled by the transport carrier. It is characterized by filling higher energy states in the less ordered regions of the polymers, leading to more efficient conduction. Due to the doping process initially filling the transport carrier-deficient sites in the polymer before efficient doping occurs, two slopes were observed in the turn-on part of the transfer curves.29 An obvious turn-on current occurred while measuring the transfer curve with a forward sweep of VG around −0.6 V, and a clockwise hysteresis occurred during the backward sweeping. The clockwise hysteresis is attributed to the limitation of ion penetration.13 In addition, doping must penetrate the film deeply, making it slower than de-doping. The higher the content of hydrophilic oligoether side chains, the more easily water-phase ions can penetrate into the polymer film, making doping and dedoping easier. Therefore, P(C,C), P(C,O) and P(O,O) exhibit a decreasing relationship in hysteresis. As shown in Fig. S13 (ESI†), the threshold voltage (Vth) values for P(C,C), P(C,O), and P(O,O) are −0.92, −0.81, and −0.52 V, respectively. Notably, the Vth values support the trend of the HOMO/LUMO levels and ΔEonset values. Since the CPs share the same conjugated backbone, the HOMO/LUMO levels are less affected by the side chain combinations. However, it can still be observed that the CP with more oligoether content in the side chains has increased HOMO/LUMO levels. Fig. S14 (ESI†) shows the product of hole mobility (μh) and volumetric capacitance (C*) obtained using
, where the transconductance (gm) was calculated from
.14 The average μhC* values for P(C,C), P(C,O), and P(O,O) are 0.13, 65, and 94 F cm−1 V−1 s−1, respectively. In addition, the OECT output characteristics were measured and summarized in Fig. S15 (ESI†). As VG exceeds Vth, the channel turns on, resulting in typical p-type output curves observed from the polymers studied.
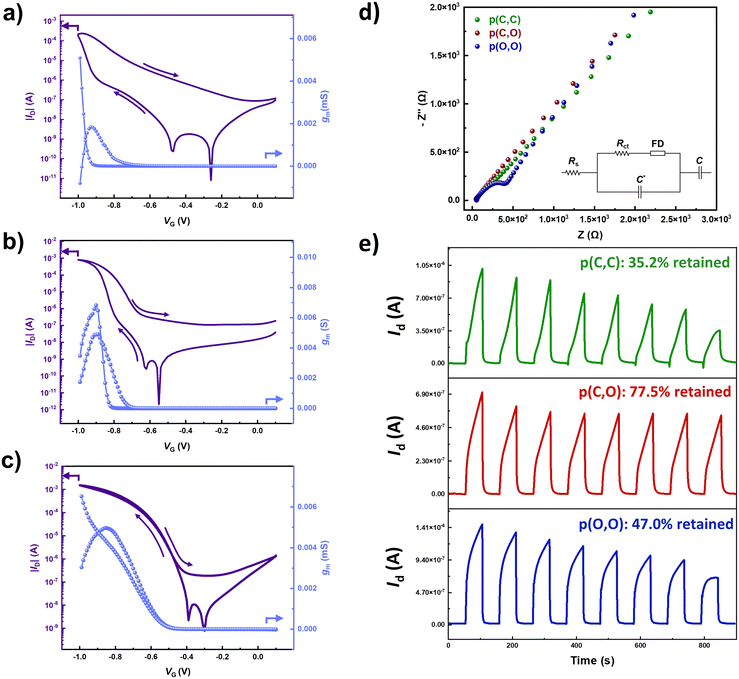 |
| Fig. 4 OECT transfer characteristics of (a) P(C,C), (b) P(C,O), and (c) P(O,O) with a forward VG sweeping from 0.1 to −1.0 V at VD = −0.3 V. (d) Nyquist plots derived from the EIS characteristics of the polymer films. (e) Transient characteristics of the polymer films with a VG pulse of −0.85 V at VD = −0.3 V for 8 cycles. | |
Table 1 Summary of the OECT device parameters based on the polymers studied
Parameters |
P(C,C)
|
P(C,O)
|
P(O,O)
|
V
th (V) |
−0.92 |
−0.81 |
−0.52 |
μ
h
C* (F cm−1 V−1 s−1) |
0.13 ± 0.01 |
65.2 ± 6.4 |
93.6 ± 6.0 |
C* (F cm−3) |
8.72 ± 0.02 |
74.2 ± 1.5 |
261 ± 16 |
μ
h (cm2 V−1 s−1) |
0.015 ± 0.002 |
0.78 ± 0.09 |
0.35 ± 0.02 |
p (×1012 cm−2) |
9.03 ± 3.92 |
9.47 ± 1.15 |
21.4 ± 5.4 |
t
r (s) |
14.2 ± 3.2 |
5.41 ± 1.58 |
5.87 ± 2.92 |
t
f (s) |
0.088 ± 0.01 |
0.035 ± 0.01 |
0.997 ± 0.57 |
I
D retention (%) |
35.2 |
77.5 |
47.0 |
Next, the C* values of polymer films were determined using electrochemical impedance spectroscopy (EIS). A three-electrode system comprising CP-coated ITO glass as a working electrode, platinum as a counter electrode, and an Ag/AgCl reference electrode was used. All electrodes were immersed in KCl(aq) to emulate the doping behavior in OECTs. Nyquist plots of polymers and the equivalent circuit are shown in Fig. 4d, and Bode plot fittings are shown in Fig. S16 (ESI†). The equivalent circuit involves a simulated RC parallel combination, hole transfer resistance (Rct), and C* with antecedent series solution resistance (Rs) and finite length diffusion (FD). The fitted C* values are summarized in Table 1. The average C* values for P(C,C), P(C,O), and P(O,O) are 8.72, 74.2, and 261 F cm−3, respectively. As a result, the hole mobility (μh) of the CP channel can be evaluated from the preceding data; the μh values for polymers are 0.015, 0.78, and 0.35 cm2 V−1 s−1 for P(C,C), P(C,O), and P(O,O), respectively. From the values of C*, it can be observed that the introduction of hydrophilic side chains does indeed enhance electrochemical doping. Both P(C,O) and P(O,O) exhibit better hydrophilicity, while μhC* values are higher. In the case of P(C,O), which combines both hydrophilic and hydrophobic side chains, it not only has appropriate capacitance and ion uptake but also maintains higher mobility levels. This is because the hydrophobic side chains help to maintain a better alignment that improves charge transport, while the hydrophilic side enhances capacitance. Therefore, P(C,O) achieves a favorable balance in charge transport and ion accommodation. Although P(O,O) exhibits the highest μhC* value, it possesses a low μh value because of its symmetric hydrophilic side chains, resulting in relatively poor packing. In addition, the high C* of P(O,O) indicates its strong uptake and this behavior may deteriorate the film stability.
To verify the relationship between polymer's hydrophilicity and ion uptake, their carrier density (p) was calculated using the equation:
, where
is the integral of the gate curve (IG) versus VG, rv is the scan rate of VG (10 mV s−1), e is the elementary charge, A is the effective gate surface area, and d is the channel thickness of each polymer.26 Fig. S17 (ESI†) shows the integration area of the IG–VG plot to derive the carrier density, and the results are summarized in Table 1. The value of p can indicate the magnitude of ion injection. P(C,O) and P(O,O) exhibit higher carrier density, as their good ion uptake and C* indicate. The lower carrier density of P(C,C) results in relatively low ion uptake and C*.
After understanding the device parameters, the response speed of the OECT devices was subsequently investigated. The transient characteristics were determined using a single transient curve obtained for different devices, as shown in Fig. S18 (ESI†). Using the exponential decay function to fit the transient curve, we can obtain the rise time (tr) and fall time (tf) of the OECT devices. These values represent the time it takes for a 90% change in ID when turning on and off the polymer channel. The corresponding tr and tf values for P(C,C), P(C,O), and P(O,O) are (14.16, 0.088) s, (5.41, 0.035) s, and (5.87, 0.997) s, respectively. The abrupt rise in positive current is due to the ionic doping rate being faster than hole transport. The negative current exponentially grows as the channel turns on and exponentially decays as the channel turns off. Note that doping requires deeper penetration into the film, resulting in a slower rate than de-doping. Therefore, when VG is removed to turn off the channel, the de-doping rate is noticeably faster than the doping rate. Polymers with hydrophilic side chains, such as P(C,O) and P(O,O), exhibit much faster doping rates compared to the hydrophobic P(C,C). Consequently, P(C,O) and P(O,O) have shorter tr. Furthermore, the tf values of P(C,C) and P(C,O) are much faster than that of P(O,O). Hydrophilic side chains tend to retain ions within the film, slowing down the de-doping rate. Thus, combining hydrophilic and hydrophobic asymmetric side chains in P(C,O) allows it to achieve the fastest tr and tf.27
After understanding the response speed, the operational stability of the OECT devices was finally investigated. Accordingly, multiple cycles of continuous on/off operations were applied. The VG values were set to be −0.8 and 0 V for on/off operations and VD was set to be −0.3 V. Each on-time interval was controlled to be 25 s. After 8 cycles of continuous operation, the charge retention (ID
retention) values of P(C,C), P(C,O), and P(O,O) were 35.2%, 77.5%, and 47.0%, respectively. From these results, it is worth noting that P(C,O) not only exhibits a faster response speed but also demonstrates better stability. This stability is attributed to the contribution of the asymmetric side-chain design. Supported by the GIXD analysis, asymmetric side chain design promotes coplanarity and structural stability. Introducing hydrophilic side chains significantly enhances orientation and crystallinity through swelling. Particularly, the P(C,O) film shows the most substantial improvement in stabilizing the crystalline properties due to its asymmetric structure, which enhances its ability to rearrange and its structural stability under swelling conditions. This structural stability ensures superior device performance and the best current retention.
To corroborate the efficacy of asymmetric side-chain design, a reference device comprising the polymer blend of P(C,C) and P(O,O) with an equal amount was fabricated and characterized. The device characteristics are presented in Fig. S18 (ESI†). As shown, the μhC* value is 21 F cm−1 V−1 s−1, much lower than 65 and 94 F cm−1 V−1 s−1 obtained for P(C,O) and P(O,O). In addition, the ID
retention value of the blend device after 8 cycles is 22.1%, which is also inferior to those of P(C,O) and P(O,O). In addition, Chou et al. recently reported a series of statistical terpolymers comprising isoindigo monomers with symmetric branched alkyl or oligoether side chains. They found that the statistical terpolymers showed significantly decreased charge-transport performance when increasing the content of the oligoether monomer to 40–50 mol%.28 Therefore, we can confirm that asymmetric side-chain engineering best accommodates different side chain patterns among statistical terpolymers and polymer blends. Therefore, we finally summarized the experimental findings in Fig. 5 to illustrate the structure–property relationship of these polymers in OECTs. In other words, asymmetric side chains can stabilize the crystalline structure alignment while enhancing the ion doping rate compared to hydrophobic side chains. This effect also improves the ion accommodation and capacitance. Therefore, P(C,O) shows a moderately high μhC* value, the best response speed, and operational stability. These findings suggest that the asymmetric design with a combination of hydrophilic and hydrophobic side chains outperforms its analogs of P(C,C) and P(O,O) with symmetric side chains. This improvement underscores the potential of asymmetric side-chain design for OECT applications with a good balance between device performance and stability.
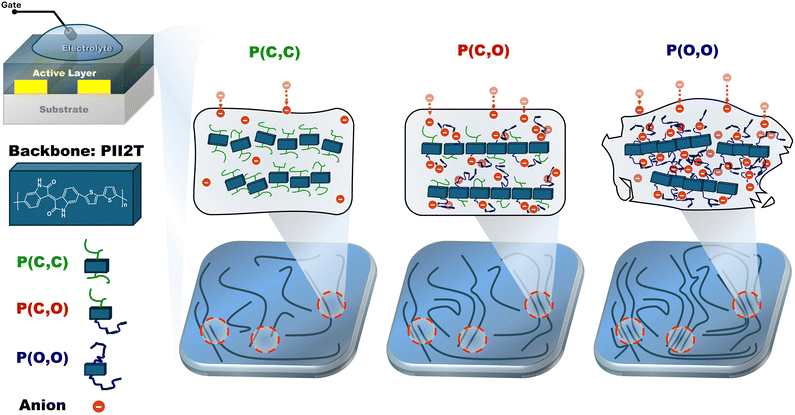 |
| Fig. 5 Schematic illustration of the structure–morphology–performance relationship of the conjugated polymers with symmetric hydrophilic/hydrophobic side chains and an asymmetric side-chain combination. | |
4. Conclusion
In conclusion, we developed a strategy to comparatively investigate the effects of asymmetric side chain design on the performance of OECTs. We introduced hydrophilic and hydrophobic side chains into PII2T-based polymers and examined the effects of symmetric and asymmetric side chain combinations. This allowed us to investigate the differences arising from various factors, including the backbone coplanarity, mobility, hydrophilicity, and stability in the OECT device. Introducing hydrophilic side chains enhances ion penetration, thereby enhancing the capacitance of P(O,O) films. To further improve charge-transport performance, we designed asymmetric side-chain engineered P(C,O). Introducing asymmetric side chains improves CP's coplanarity and charge-transport performance. In addition, the oligoether side chains enhance the film's hydrophilicity and ion uptake. Morphological analysis using GIXD analysis revealed film structure and crystallinity changes due to side chain arrangement and hydrated-ion penetration. Asymmetric side chain design promotes coplanarity and structure stability. We observed that introducing hydrophilic side chains appropriately and significantly promoted orientation and crystallinity through swelling. Particularly, the P(C,O) film exhibited the most substantial enhancement in crystalline properties after swelling. This improvement can be attributed to the asymmetric structure of P(C,O), which contributed to a superior structural arrangement, enhancing its ability to rearrange and maintain stability under swelling conditions. Consequently, P(C,C) and P(O,O) with symmetric side chains exhibited the device parameters: μh (cm2 V−1 s−1), C* (F cm−3), ID
retention (%) of (0.015, 8.72, 35.2) and (0.35, 260, 47.0), respectively. In stark contrast, P(C,O) showed satisfying μh (cm2 V−1 s−1), C* (F cm−3), and ID
retention (%) values of (0.78, 74.2, 77.5). Even while maintaining a capacitance similar to that of P(O,O), the stability of P(C,O) is far better than that of P(O,O). The device performance of P(C,O) does not fall between that of P(C,C) and P(O,O), but rather is superior to that of both. Overall, the combination of hydrophilic and hydrophobic side chains in P(C,O) resulted in improved mobility, response speed, and stability compared to those of symmetric counterparts. Our findings highlight the potential of asymmetric side chain designs to enhance the performance of CPs for OECT applications. The combination of hydrophilic and hydrophobic side chains offers a promising approach to optimize device performance.
Author contributions
Guo-Hao Jiang: polymer synthesis and characterization, device characterization, and writing – original draft; Chia-Ying Li: investigation, validation, and methodology; Shang-Wen Su: data curation and formal analysis; Yan-Cheng Lin: polymer design, conceptualization, supervision, resources, and manuscript reviewing and editing. All authors read and approved the final manuscript.
Data availability
The data that support the findings of this study are available from the corresponding author upon reasonable request.
Conflicts of interest
The authors declare that they have no known competing financial interests or personal relationships that could have appeared to influence the work reported in this article.
Acknowledgements
The authors are grateful for the financial support from the National Science and Technology Council in Taiwan (NSTC 111-2222-E-006-020-MY2) and the Featured Area Research Center Program within the framework of the Higher Education Sprout Project by the Ministry of Education (113L9006). The authors also acknowledge the National Synchrotron Radiation Research Center (NSRRC) of Taiwan for the GIXD experiments in BL13A (TLS). The authors gratefully acknowledge the use of NMR (NMR005000), elemental analysis (EA000600), and the film thickness measurement (OTHER003600) of NSTC 112-2740-M-006-001 belonging to the Core Facility Center of National Cheng Kung University.
References
- L. Ding, Z. D. Yu, X. Y. Wang, Z. F. Yao, Y. Lu, C. Y. Yang, J. Y. Wang and J. Pei, Chem. Rev., 2023, 123, 7421–7497 CrossRef CAS PubMed.
- M. Ashizawa, Y. Zheng, H. Tran and Z. Bao, Prog. Polym. Sci., 2020, 100, 101181 CrossRef CAS.
- M. Kim, S. U. Ryu, S. A. Park, K. Choi, T. Kim, D. Chung and T. Park, Adv. Funct. Mater., 2019, 30, 1904545 CrossRef.
- T. Liu, J. Heimonen, Q. Zhang, C. Y. Yang, J. D. Huang, H. Y. Wu, M. A. Stoeckel, T. P. A. van der Pol, Y. Li, S. Y. Jeong, A. Marks, X. Y. Wang, Y. Puttisong, A. Y. Shimolo, X. Liu, S. Zhang, Q. Li, M. Massetti, W. M. Chen, H. Y. Woo, J. Pei, I. McCulloch, F. Gao, M. Fahlman, R. Kroon and S. Fabiano, Nat. Commun., 2023, 14, 8454 CrossRef CAS PubMed.
- W. Liu, C. Zhang, R. Alessandri, B. T. Diroll, Y. Li, H. Liang, X. Fan, K. Wang, H. Cho, Y. Liu, Y. Dai, Q. Su, N. Li, S. Li, S. Wai, Q. Li, S. Shao, L. Wang, J. Xu, X. Zhang, D. V. Talapin, J. J. de Pablo and S. Wang, Nat. Mater., 2023, 22, 737–745 CrossRef CAS.
- Y. C. Lin, F. H. Chen, Y. C. Chiang, C. C. Chueh and W. C. Chen, ACS Appl. Mater. Interfaces, 2019, 11, 34158–34170 CrossRef CAS.
- X. Yu, L. Chen, C. Li, C. Gao, X. Xue, X. Zhang, G. Zhang and D. Zhang, Adv. Mater., 2023, 35, e2209896 CrossRef PubMed.
- Y. Zheng, S. Zhang, J. B. Tok and Z. Bao, J. Am. Chem. Soc., 2022, 144, 4699–4715 CrossRef CAS PubMed.
- W. Yang, K. Feng, S. Ma, B. Liu, Y. Wang, R. Ding, S. Y. Jeong, H. Y. Woo, P. K. L. Chan and X. Guo, Adv. Mater., 2024, 36, e2305416 CrossRef PubMed.
- J. S. Park, G. U. Kim, S. Lee, J. W. Lee, S. Li, J. Y. Lee and B. J. Kim, Adv. Mater., 2022, 34, e2201623 CrossRef PubMed.
- Z. Li, L. Ying, P. Zhu, W. Zhong, N. Li, F. Liu, F. Huang and Y. Cao, Energy Environ. Sci., 2019, 12, 157–163 RSC.
- H.-C. Yen, Y.-C. Lin and W.-C. Chen, Macromolecules, 2021, 54, 1665–1676 CrossRef CAS.
- J. Rivnay, S. Inal, A. Salleo, R. M. Owens, M. Berggren and G. G. Malliaras, Nat. Rev. Mater., 2018, 3, 17086 CrossRef CAS.
- D. Ohayon, V. Druet and S. Inal, Chem. Soc. Rev., 2023, 52, 1001–1023 RSC.
- T. Sedghamiz, A. Y. Mehandzhiyski, M. Modarresi, M. Linares and I. Zozoulenko, Chem. Mater., 2023, 35, 5512–5523 CrossRef CAS.
- L. Q. Flagg, C. G. Bischak, J. W. Onorato, R. B. Rashid, C. K. Luscombe and D. S. Ginger, J. Am. Chem. Soc., 2019, 141, 4345–4354 CrossRef CAS PubMed.
- A. Savva, C. Cendra, A. Giugni, B. Torre, J. Surgailis, D. Ohayon, A. Giovannitti, I. McCulloch, E. Di Fabrizio, A. Salleo, J. Rivnay and S. Inal, Chem. Mater., 2019, 31, 927–937 CrossRef CAS.
- Z. Sun, B. Khau, H. Dong, C. J. Takacs, S. Yuan, M. Sun, B. Mosevitzky Lis, D. Nguyen and E. Reichmanis, Chem. Mater., 2023, 35, 9299–9312 CrossRef CAS PubMed.
- K. Feng, J. Wang, S. Y. Jeong, W. Yang, J. Li, H. Y. Woo and X. Guo, Adv. Sci., 2023, 10, e2302629 CrossRef.
- Y. Wang, A. Koklu, Y. Zhong, T. Chang, K. Guo, C. Zhao, T. C. H. Castillo, Z. Bu, C. Xiao, W. Yue, W. Ma and S. Inal, Adv. Funct. Mater., 2023, 34, 2304103 CrossRef.
- Y. Yu, G. Zhu, L. Lan, J. Chen, X. Zhu, J. Duan, S. Cong, Z. Li, Y. Wang, Z. Wang, I. McCulloch and W. Yue, Adv. Funct. Mater., 2023, 33, 2300012 CrossRef CAS.
- Y. Wang, E. Zeglio, L. Wang, S. Cong, G. Zhu, H. Liao, J. Duan, Y. Zhou, Z. Li, D. Mawad, A. Herland, W. Yue and I. McCulloch, Adv. Funct. Mater., 2022, 32, 2111439 CrossRef CAS.
- Y. Yuan, F. Zhao, Y. Ding, G. Zhang, X. Wang and L. Qiu, Macromol. Rapid Commun., 2022, 43, e2100636 CrossRef PubMed.
- G. Xue, X. Zhao, G. Qu, T. Xu, A. Gumyusenge, Z. Zhang, Y. Zhao, Y. Diao, H. Li and J. Mei, ACS Appl. Mater. Interfaces, 2017, 9, 25426–25433 CrossRef CAS PubMed.
- Y.-C. Lin, C.-C. Shih, Y.-C. Chiang, C.-K. Chen and W.-C. Chen, Polym. Chem., 2019, 10, 5172–5183 RSC.
- X. Teng, J. Sun, J. Jiang, S. Ke, J. Li, Z. Lou, Y. Hou, Y. Hu and F. Teng, Org. Electron., 2023, 120, 106859 CrossRef CAS.
- M. Mooney, A. Nyayachavadi, A. Awada, E. Iakovidis, Y. Wang, M.-N. Chen, Y. Liu, J. Xu, Y.-C. Chiu, X. Gu and S. Rondeau-Gagné, Polym. Chem., 2023, 14, 562–572 RSC.
- S. Chuo, Y.-C. Peng, T. Puangniyom, Q.-G. Chen, C.-C. Chueh and W.-Y. Lee, RSC Appl. Interfaces, 2024 10.1039/D4LF00093E.
- S. T. Keene, J. E. M. Laulainen, R. Pandya, M. Moser, C. Schnedermann, P. A. Midgley, I. McCulloch, A. Rao and G. G. Malliaras, Nat. Mater., 2023, 22, 1121–1127 CrossRef CAS PubMed.
|
This journal is © The Royal Society of Chemistry 2024 |