DOI:
10.1039/D4TC00428K
(Paper)
J. Mater. Chem. C, 2024,
12, 6098-6105
Homochirality to design high-Tc lead-free ferroelastic semiconductors†
Received
30th January 2024
, Accepted 1st April 2024
First published on 2nd April 2024
Abstract
Ferroelastic semiconductor materials have garnered significant research interest due to their promising applications in the fields of shape memory, superelasticity, templated electronic nanostructures, mechanical switching, and optoelectronic transmission. However, the toxicity of lead-based structures and low phase-transition temperature (Tc) greatly constrain the application scenarios of ferroelastic semiconductors. Here, using an H/OH-substitution-induced homochiral strategy, we synthesize a pair of lead-free ferroelastic semiconductors (R/S-CTA)2SbCl5 (CTA = 3-chloro-2-hydroxypropyltrimethyllammonium) having semiconductor properties with an indirect bandgap of 3.41 eV. They crystallized in the chiral space group P212121 at room temperature, and both undergo 422F222 type ferroelastic phase transitions with Tc up to 410 K, accompanied by a large entropy change of 68.75 and 66.09 J mol−1 K−1, respectively. Owing to the introduction of chirality, they exhibited temperature-dependent nonlinear second-harmonic generation (SHG) properties. Relatively, the achiral TMCP (TMCP = N,N,N-trimethylchloropropylamine) makes the phase transition properties of centrosymmetric TMCP2SbCl5 ordinary compared to chiral R/S-pair. This is precisely the main starting point of homochiral strategies in phase transition and optical structure research, while arousing research interest. This work, which provides a new avenue for the design of high-Tc lead-free ferroelastic semiconductor compounds, is a powerful motivation for the realization of multifunctional materials related to chirality.
Introduction
Organic–inorganic hybrid perovskites have been the focus of research in the fields of chips, photovoltaics, and batteries due to their good mechanical reversibility, long carrier transport distance, and high structural flexibility.1–18 Recently, the ferroelasticity of the popular hybrid semiconductor CH3NH3PbI3 has been demonstrated to be beneficial for the improvement of photovoltaic efficiency,19 while the ferroelastic domain wall facilitates benign carrier transport, thus giving rise to extensive research on ferroelastic semiconductors.20–22 However, most of the reported ferroelastic semiconductors are lead halide-based structures, where heavy metals are toxic and can cause great harm to organisms and the environment.23–27 Organic–inorganic hybrid perovskite semiconductor structures have structural diversity and tunability, and metallic lead can usually be replaced by non-toxic metallic elements such as Ge(II), Sn(II), Bi(III), and Sb(III).28–30 Therefore, some lead-free semiconductors have been constructed by this method, such as dimethylamineGeI3,31 [R-3-hydroxylpiperidinium]2SbCl5,32 and (3-bromopropylaminium)4AgBiBr8.33 In addition, the low phase transition temperatures in these hybrid perovskites ferroelastic semiconductors limit their further applications at high temperatures and in special environments.34–39 Hence, it is imperative to advance the development of lead-free ferroelastic semiconductors with high phase transition temperatures.
Halogen substitution,25,26,39 mixed cation engineering,40 and the introduction of homochirality23,26,32,41,42 are commonly used to regulate ferroelastic materials in organic–inorganic hybrid systems. The incorporation of homochirality is advantageous for achieving a multi-channel bistable response in ferroelastic materials, garnering widespread research and attention. Luo and his coworkers introduced homochirality into (Rac-3-OHpyl)PbBr3 (OHpyl = hydroxypyrrolium), achieving a pair of eight channel bistable response of chiral ferroelastic compound (R/S-3-OHpyl)PbBr3 with dielectric, DSC, SHG, ferroelasticity, conductivity, photoluminescence, circular dichroism, and photocurrent response.43 Xiong's group reported a thermochromic ferroelastic compound (R/S-CTA)2CuCl4 with seven physical channels.44 The introduction of homochirality can be used to precisely design ferroelastic materials with multi-channel switches, but it does have certain limitations in obtaining ferroelastic materials with high-Tc. Therefore, we use the H/OH-substitution-induced homochiral strategy to achieve the construction of high-Tc ferroelastic semiconductors. On the one hand, the introduction of hydroxyl groups induces hydrogen bonding, which increases the potential energy barrier for cation motion to raise the Tc.36,45 On the other hand, the introduction of chiral cations alters the microstructure of the crystals, allowing the construction of ferroelasticity, and also enables the compounds to crystallize in non-centrosymmetric space groups, enabling the molecules to be SHG-responsive.41,42,44,46,47
Based on the homochiral strategy, we have successfully synthesized high-Tc lead-free ferroelastic semiconductor compounds (R-CTA)2SbCl5 and (S-CTA)2SbCl5 (CTA = 3-chloro-2-hydroxypropyltrimethyllammonium). (R-CTA)2SbCl5 and (S-CTA)2SbCl5 undergo a plastic phase transition at 410 K, having a large entropy change of 68.75 and 66.09 J mol−1 K−1, respectively. Compared to TMCP2SbCl5 (TMCP = N,N,N-trimethylchloropropylamine), the introduction of hydroxyl groups increases the Tc of (R/S-CTA)2SbCl5 by 20 K, and the introduction of chirality allows (R/S-CTA)2SbCl5 to crystallize in non-centrosymmetric space groups, accompanied by switchable second-harmonic generation (SHG) between SHG-active and SHG-inactive states. A comparison of variable-temperature PXRD and SHG results reveals that (R/S-CTA)2SbCl5 undergoes the 422F222 type ferroelastic phase transition and possesses excellent temperature dependence and reversibility. This work has successfully obtained high-Tc lead-free ferroelastic semiconductor compounds using the strategy of H/OH-substitution-induced homochirality (Scheme 1), while injecting fresh energy into the realization of chiral related multifunctional materials.
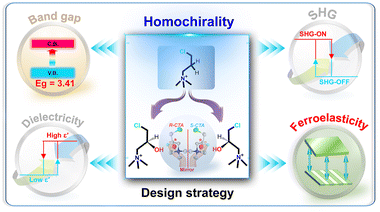 |
| Scheme 1 Schematic diagram of the molecular design strategy for constructing high-Tc lead-free ferroelastic semiconductors. | |
Results and discussion
Crystal structure analysis
In order to explore the details and microscopic mechanisms of the crystal structure, we used X-ray diffraction to measure the crystallographic data of TMCP2SbCl5 and (R/S-CTA)2SbCl5. At 293 K, TMCP2SbCl5 crystallizes in the monoclinic central space group P21/c, with unit cell parameters of a = 8.5967(4) Å, b = 31.8060(15) Å, c = 9.6375(5) Å, β = 115.487°, V = 2378.7(2) Å3, and Z = 4. The inorganic skeleton and the cations exhibit an ordered state (Fig. S4a, ESI†). The inorganic skeleton is connected to 5 Cl atoms with Sb atoms as the center, presenting a pyramid configuration. The Sb–Cl bond length and Cl–Sb–Cl bond angle range from 2.3680(14) to 2.6774(12) and from 87.83(7) to 177.62(5), respectively (Table S3, ESI†). The inorganic skeleton and organic cations alternate to form a zero-dimensional packing structure (Fig. 1a). The introduction of chirality often brings about structural changes, thereby inducing some novel properties. Here we use hydroxyl group substitution to induce homochirality, allowing (R/S-CTA)2SbCl5 to crystallize in the chiral space group, which not only induces SHG signals but also enables ferroelastic phase transitions. (R/S-CTA)2SbCl5 crystallizes in the space group P212121, with unit cell parameters of a = 8.9032(3) Å, b = 9.5971(3) Å, c = 28.2922(8) Å, V = 2417.42(12) Å3, Z = 4 and a = 8.9021(2) Å, b = 9.5969(2) Å, c = 28.2865(7) Å, V = 2416.59(10) Å3, Z = 4 at 293 K, respectively. The smallest independent unit consists of two CTA cations and one [SbCl5]2− anion skeleton (Fig. S4b and c, ESI†), with the organic part exhibiting an ordered state. The inorganic skeleton in (S-CTA)2SbCl5 is similar to that in TMCP2SbCl5, with the Sb–Cl bond length and Cl–Sb–Cl bond angle ranging from 2.3606(10) to 2.6993(12) and 87.46(4) to 176.62(4), respectively (Table S1, ESI†). (R/S-CTA)2SbCl5 exhibits a zero-dimensional packing form, and possesses a mirror symmetry relationship (Fig. 1b). In Fig. 1d, there is an O–H⋯Cl interaction force between the cation and the anion in (R-CTA)2SbCl5, and the stretching leads to a longer Sb–Cl4 bond. In contrast, there is no hydrogen bonding interaction in TMCP2SbCl5, so the bond length of Sb–Cl4 in TMCP2SbCl5 is smaller than that of Sb–Cl4 in (R-CTA)2SbCl5 (Fig. 1c and d).
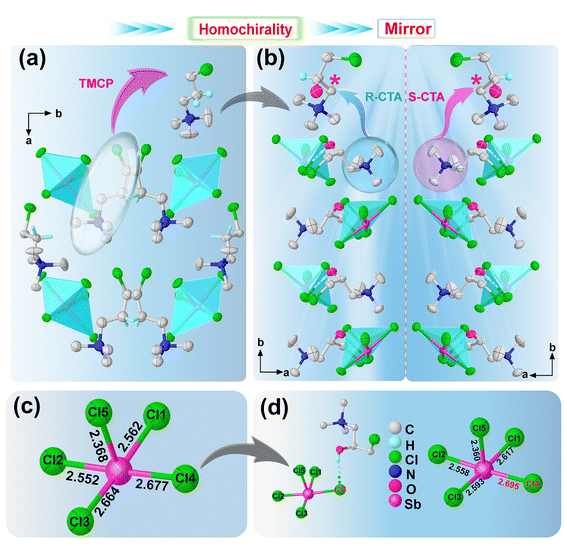 |
| Fig. 1 Packing view along the c-axis of the crystal structure of TMCP2SbCl5 (a) and (R/S-CTA)2SbCl5 (b). Geometry of the [SbCl5]2− pyramid of TMCP2SbCl5 (c). Hydrogen-bonding interactions between the inorganic skeleton and R-CAT cations, and geometry of the [SbCl5]2− pyramid of (R-CTA)2SbCl5 (d). | |
Intermolecular interaction analysis
To further illustrate that (S/R-CTA)2SbCl5 has relatively stronger intermolecular internal forces compared to TMCP2SbCl5, the CrystalExplorer software was used to calculate and evaluate the Hirshfeld dnorm surfaces, and two-dimensional fingerprints of cations were plotted. The results show that the short contact (red concave area on the surface) between R/S-CTA cations is more compact than that of TMCP cations (Fig. 2a–c). At the same time, from Fig. 2d–f, it can be seen that the minimum (di, de) values of (R/S-CTA)2SbCl5 are (0.8103, 1.6105) and (0.8095, 1.6096), respectively, which are shorter than the minimum (di, de) values of (0.9562, 1.7954) in TMCP2SbCl5. It is obvious that the contact between R/S-CTA cations is stronger than that of TMCP cations, which greatly increases the motion barrier of cations. The results indicate that there is an O–H⋯Cl hydrogen bonds interaction force between organic cations and [SbCl5]2− inorganic anions, and it play an important role in increasing the Tc.
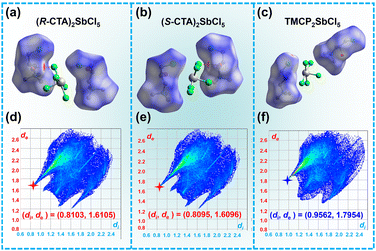 |
| Fig. 2 Hirshfeld dnorm surfaces of (R-CTA)2SbCl5 (a), (S-CTA)2SbCl5 (b), and (TMCP)2SbCl5 (c). 2D fingerprint plots of R/S-CTA cations (d and e) and the TMCP cation (f). | |
Plastic phase transition analysis
Structural changes are often accompanied by changes in thermal properties, and DSC measurements of TMCP2SbCl5 and (R/S-CTA)2SbCl5 are carried out at a rate of 20 K min−1 in the temperature range of 320 K to 430 K. Fig. 3a and b show that TMCP2SbCl5 and (R/S-CTA)2SbCl5 exhibit a pair of endothermic/exothermic peaks during heating and cooling, indicating that TMCP2SbCl5 and (R/S-CTA)2SbCl5 undergo reversible phase transitions at 390 K and 410 K, respectively. There is a significant difference in the phase transition between compounds TMCP2SbCl5 and (R/S-CTA)2SbCl5, which is due to the introduction of hydroxyl groups that generate hydrogen bonds, increasing the energy barrier of (R/S-CTA)2SbCl5 and the Tc of (R/S-CTA)2SbCl5 by 20 K. Through the homochiral strategy, the Tc of (R/S-CTA)2SbCl5 is at a higher level in the lead-free ferroelastic semiconductor32,33,40,48–58 (Fig. 3c). In addition, the thermal hysteresis of 20 K and 45 K reveal the first-order phase transition type of TMCP2SbCl5 and (R/S-CTA)2SbCl5. The entropy changes (ΔS) of TMCP2SbCl5 and (R/S-CTA)2SbCl5 are 1.83, 68.75, and 66.09 J mol−1 K−1, respectively. At the same time, the entropy change during phase transition is greater than that during melting, indicating that (R/S-CTA)2SbCl5 exhibits the characteristic of plastic phase transition (Fig. 3g and Fig. S3 ESI†). The larger ΔS indicates that (R/S-CTA)2SbCl5 shows a high degree of disorder in the plastic phase, and their phase transition type should be ordered–disordered ferroelastic phase transition. The dielectric constant, as a temperature dependent function, usually exhibits significant abrupt changes near the Tc. During the heating process, the real part (ε′) of the dielectric constant of TMCP2SbCl5 and (R/S-CTA)2SbCl5 exhibits a stepped mutation near 390 K and 410 K, respectively. During cooling operation, the ε′ exhibits the same trend of change at 340 K and 365 K, which is consistent with the results of DSC (Fig. 3c–e). It is worth noting that near the phase transition point, the ε′ value undergoes nearly twice the change, which is consistent with the larger entropy change of (R/S-CTA)2SbCl5. TMCP2SbCl5 exhibits insignificant dielectric anomalies near the phase transition temperature, indicating slight structural changes in the vicinity of the phase transition.
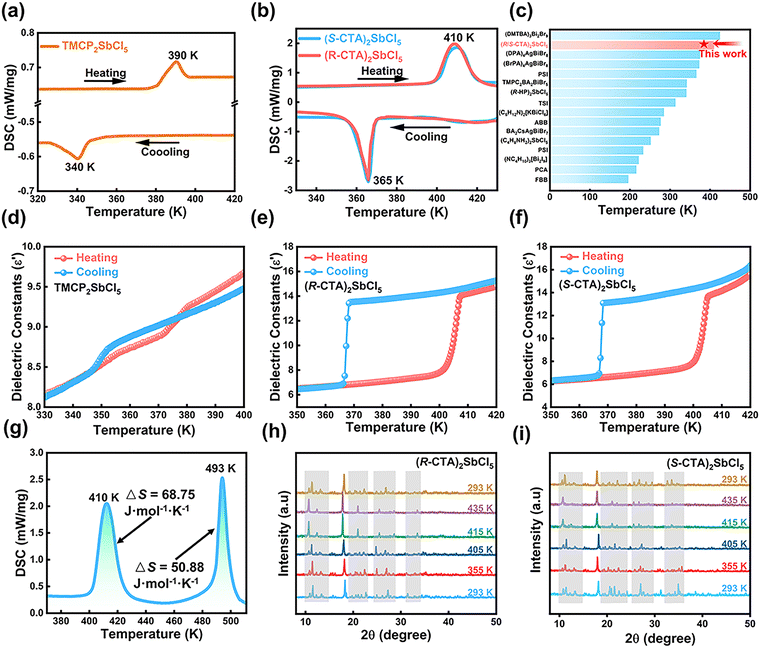 |
| Fig. 3 DSC curves of TMCP2SbCl5 (a) and (R/S-CTA)2SbCl5 (b). Reported Tc of lead-free ferroelastic semiconductor materials (c). FBB, PCA (NC4H10)3[Bi2I9], PSI, (C4H8NH2)SbCl5, BA2CsAgBiBr7, ABB, (C5H12N)2[KBiCl6], TSI, (R-HP)2SbCl5, (BrPA)4AgBiBr8, (DPA)4AgBiBr8, and (DMTB)3ABiBr9. Dielectric curves of TMCP2SbCl5 (d), (R-CTA)2SbCl5 (e), and (S-CTA)2SbCl5 (f). The entropy change of the plastic phase transition surpasses that of melting. (g). Variable-temperature PXRD patterns of (R-CTA)2SbCl5 (h) and (S-CTA)2SbCl5 (i). | |
Variable-temperature PXRD
Due to the fact that (R/S-CTA)2SbCl5 is a plastic crystal above Tc, its accurate high-temperature structure cannot be obtained. In order to further obtain high temperature structural data for (R/S-CTA)2SbCl5, variable-temperature powder diffraction was used to measure the PXRD data of (R/S-CTA)2SbCl5. When the temperature is 300 K, the PXRD data of (R/S-CTA)2SbCl5 well match the data obtained from crystal structure simulation, which proves that (R/S-CTA)2SbCl5 has good crystallinity and purity (Fig. S1, ESI†). As shown in Fig. 3h and i, the (R/S-CTA)2SbCl5 powder diffraction plot did not change below Tc, indicating that the sample has good thermal stability. After reaching the Tc, the PXRD pattern of (R/S-CTA)2SbCl5 shows significant changes. As (R/S-CTA)2SbCl5 is a pair of enantiomers with the same phase transition type, only (R-CTA)2SbCl5 will be analyzed in detail. In the range of 10–18°, a series of peaks disappear, but new diffraction peaks appear at 11°. From 20–30°, new diffraction peaks appear at 22° and 26°, but all other peaks in this range disappear. In the high angle region bigger than 30°, only a new diffraction peak appears at 34°, and the rest of the diffraction peaks remain unchanged. When cooling to room temperature, the PXRD pattern of (R-CTA)2SbCl5 corresponds to the original pattern, indicating good thermal reversibility of the sample. After the phase transition point, the number of diffraction peaks of (R-CTA)2SbCl5 significantly decreases, indicating that (R-CTA)2SbCl5 has high symmetry after the phase transition. Through Pawley refinement and simulation of the PXRD pattern at 435 K, it was found that the structure of (R-CTA)2SbCl5 above the Tc belongs to the 422 point group of the tetragonal crystal system (Fig. S5, ESI†).
Nonlinear optical properties
Nonlinear materials with the SHG effect are often used to detect non-centrosymmetric crystals, as the SHG effect occurs in non-centrosymmetric crystals. The introduction of chirality lead (R/S-CTA)2SbCl5 to crystallize in the non-centrosymmetric space group P212121. As shown in Fig. 4c and d, at 273 K, the SHG signal of (R/S-CTA)2SbCl5 is very active. During the heating process, (R/S-CTA)2SbCl5 still has SHG intensity and can maintain a stable SHG intensity at temperatures below Tc, which is defined as the SHG-active state. When the temperature reaches near the Tc, the SHG intensity rapidly decreases to zero, which can be regarded as an inactive state of SHG. During the cooling process, the SHG intensity can be restored to its original state. The SHG intensity of (R/S-CTA)2SbCl5 switches back and forth between the SHG-active and SHG-inactive states, indicating that (R/S-CTA)2SbCl5 is a potential nonlinear switching material. Although (R/S-CTA)2SbCl5 is still in the chiral space group at high temperatures, according to the Kleinman rule, the chiral space group 422 622 432 does not exhibit SHG-activity, which is very consistent with the simulation results of variable temperature PXRD (Fig. S5a and b, ESI†). At the same time, the SHG intensity of (R/S-CTA)2SbCl5 was measured in the low-temperature phase, which is approximately 0.6 and 0.5 times (Fig. S6, ESI†) that of the typical second-order nonlinear material KDP (KH2PO4). It is noteworthy that the SHG intensities of (R-CTA)2SbCl5 and (S-CTA)2SbCl5 are not equal, which may be due to the size-dependent SHG intensity. Therefore, the SHG intensities of (R/S-CTA)2SbCl5 at different particle sizes were measured for phase matching analysis, and Fig. 4c shows that the SHG intensity of the (R/S-CTA)2SbCl5 gradually increases with increasing particle size, which indicates that (R/S-CTA)2SbCl5 have excellent SHG phase matching characteristics.
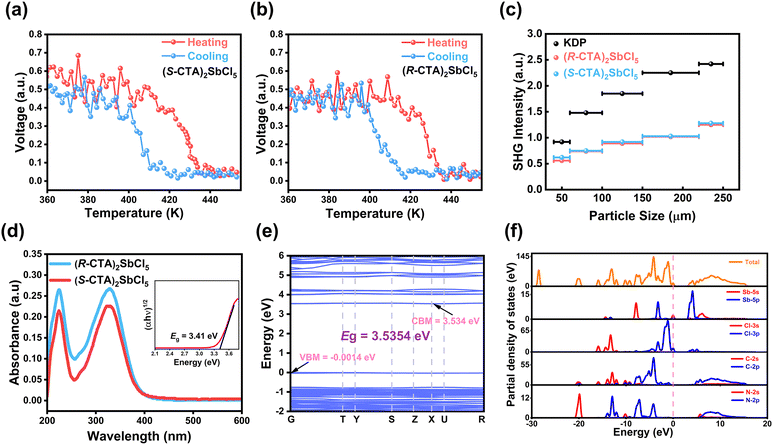 |
| Fig. 4 The changes of SHG intensity of (R-CTA)2SbCl5 (a) and (S-CTA)2SbCl5 (b) during heating and cooling. The SHG intensity of (R/S-CTA)2SbCl5 and KDP with different particle sizes at room temperature (c). UV-vis absorption spectra of (R/S-CTA)2SbCl5. The inset shows the Tauc plot for (R-CTA)2SbCl5 (d). The energy-band structure (e) and the partial density of states (PDOS) (f) of (R-CTA)2SbCl5. | |
Semiconductors
Due to the inherent semiconductor properties of stibium-based compounds, the band structure of (R/S-CTA)2SbCl5 crystals was studied at room temperature through solid-state UV-visible spectroscopy and theoretical calculations. The UV visible absorption spectrum of (R/S-CTA)2SbCl5 shows strong absorption at the beginning of the band edge at 400 nm (Fig. 4c). Since (R-CTA)2SbCl5 and (S-CTA)2SbCl5 are a pair of enantiomers, (R-CTA)2SbCl5 is chosen for analysis. Using the Tauc plot (αhν)1/n = A(hν − Eg) (where α, h, ν, and A represent the absorption coefficient, Planck constant, light frequency, and proportionality constant, respectively, and n = 2 represents the indirect bandgap and n = 1/2 represents the direct bandgap), the characteristics of the indirect bandgap semiconductor can be obtained (Fig. 4d, illustration). In order to gain a deeper understanding of the electronic structure of (R-CTA)2SbCl5 (Fig. 4e), we also calculated the band structure, with the conduction band minimum (CBM) and valence band maximum (VBM) located at the vectors of different Brillouin regions. From this, we can conclude that (R-CTA)2SbCl5 is an indirect bandgap semiconductor, which is very consistent with the experimental phenomenon. It is worth noting that the energy distribution of the VBM is quite flat, indicating that the electronic states of (R-CTA)2SbCl5 are highly localized due to the limited charge carriers.25 In addition, energy bands can be allocated based on partial density of states (PDOS) (Fig. 4f). From the PDOS perspective, the energy band at the top of the VBM originates from the unbonded state of Cl-3p, while the energy band at the bottom of the CBM mainly comes from the unoccupied Sb-5p orbital. Obviously, the bandgap of the material is determined by the inorganic [SbCl5]2− skeleton.
Ferroelasticity
Based on the simulation results of variable-temperature PXRD and the measurement results of variable-temperature SHG, it can be inferred that (R/S-CTA)2SbCl5 belongs to the 422 point in the high-temperature phase, therefore (R/S-CTA)2SbCl5 undergoes the 422F222 type phase transition. According to the Auzi rule,59 it undergoes one of the 94 types of ferroelastic phase transitions. As shown in Fig. 5a, films were prepared by dropwise addition of a mixed solution of DMSO (2 mL) and (R-CTA)2SbCl5 (30 mg) onto ITO glass and then heated at 323 K for 50 min. (R-CTA)2SbCl5 was used as a representative to observe its ferroelastic domain transition using a polarizing microscope (Fig. 5b). In the initial state (300 K), (R-CTA)2SbCl5 belongs to the ferroelastic phase, and the thin film crystal of (R-CTA)2SbCl5 has a single domain state. When the temperature rises to the Tc (430 K), (R-CTA)2SbCl5 transitions to the paraelastic phase, and the sample transforms into a highly symmetric tetragonal phase. The single domain state disappears when viewed under a polarizing microscope. When the temperature cools to room temperature, the sample exhibits striped domain structures again. The significant changes in ferroelastic domains with temperature evolution demonstrate the ferroelasticity of (R-CTA)2SbCl5. At the same time, the samples are measured in two heating and cooling cycles, and the results showed that the domain structure of the sample switched between appearance and disappearance, indicating that the domain structure of (R-CTA)2SbCl5 has outstanding reversibility and stability.
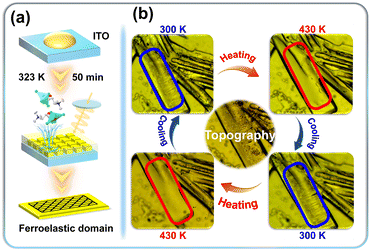 |
| Fig. 5 Preparation process of (R-CTA)2SbCl5 thin films (a). Evolution of ferroelastic domains for (R-CTA)2SbCl5 (b). | |
Conclusions
In summary, the high-Tc lead-free ferroelastic semiconductor (R/S-CTA)2SbCl5 with a direct bandgap of 3.41 eV has been successfully synthesized using the H/OH-substitution-induced homochiral strategy. They undergo plastic phase transition with a large entropy gain at 410 K (ΔS = 68.75 J mol−1 K−1 much higher than its melting enthalpy). Due to the introduction of chirality, they crystallize in the non-centrosymmetric space group P212121 exhibiting good SHG signals, while also increasing the Tc of (R/S-CTA)2SbCl5 by 20 K. Variable-temperature PXRD and SHG data indicate that (R/S-CTA) 2SbCl5 undergoes a 422F222 type ferroelastic phase transition at 410 K. With the change of external temperature, the ferroelastic domain structure of (R/S-CTA)2SbCl5 undergoes significant changes. This work, which provides an approach for obtaining high-Tc lead-free ferroelastic semiconductors, is a powerful motivation for the realization of multifunctional materials related to chirality.
Author contributions
B.-W. Deng performed the synthesis and analysis of the compounds; Z.-P. Rao and M.-J. Shen checked the writing; K.-W. Liang designed part of the experimental figures; Z.-X. Zhang, Y. Zhang and D.-W. Fu guided the synthesis and writing; Zhu and other authors analyzed the experimental data and discussed the conclusions.
Conflicts of interest
There are no conflicts to declare.
Acknowledgements
The authors thank the support of the Jiangsu Key Laboratory for Science and Applications of Molecular Ferroelectrics, and Southeast University.
References
- C. C. Fan, X. B. Han, B. D. Liang, C. Shi, L. P. Miao, C. Y. Chai, C. D. Liu, Q. Ye and W. Zhang, Adv. Mater., 2022, 34, e2204119 CrossRef PubMed
.
- J. Harada, M. Takehisa, Y. Kawamura, H. Takahashi and Y. Takahashi, Adv. Electron. Mater., 2022, 8, 2101415 CrossRef CAS
.
- M. M. Lun, H. F. Ni, Z. X. Zhang, J. Y. Li, Q. Q. Jia, Y. Zhang, Y. Zhang and D. W. Fu, Angew. Chem., Int. Ed., 2024, 63, e202313590 CrossRef CAS PubMed
.
- J. Y. Li, T. Zhang, M. M. Lun, Y. Zhang, L. Z. Chen and D. W. Fu, Small, 2023, 19, e2301364 CrossRef PubMed
.
- J. X. Gao, W. Y. Zhang, Z. G. Wu, Y. X. Zheng and D. W. Fu, J. Am. Chem. Soc., 2020, 142, 4756–4761 CrossRef CAS PubMed
.
- Y. Ai, P. F. Li, M. J. Yang, Y. Q. Xu, M. Z. Li and R. G. Xiong, Chem. Sci., 2022, 13, 748–753 RSC
.
- B. D. Liang, C. C. Fan, C. D. Liu, C. Y. Chai, X. B. Han and W. Zhang, Nat. Commun., 2022, 13, 6599 CrossRef CAS PubMed
.
- L. P. Miao, N. Ding, N. Wang, C. Shi, H. Y. Ye, L. Li, Y. F. Yao, S. Dong and Y. Zhang, Nat. Mater., 2022, 21, 1158–1164 CrossRef CAS PubMed
.
- Y. Zhang, X.-J. Song, Z.-X. Zhang, D.-W. Fu and R.-G. Xiong, Matter, 2020, 2, 697–710 CrossRef
.
- Y. Xie, Y. Ai, Y. L. Zeng, W. H. He, X. Q. Huang, D. W. Fu, J. X. Gao, X. G. Chen and Y. Y. Tang, J. Am. Chem. Soc., 2020, 142, 12486–12492 CrossRef CAS PubMed
.
- X. J. Song, T. Zhang, Z. X. Gu, Z. X. Zhang, D. W. Fu, X. G. Chen, H. Y. Zhang and R. G. Xiong, J. Am. Chem. Soc., 2021, 143, 5091–5098 CrossRef CAS PubMed
.
- H. Cho, S.-H. Jeong, M.-H. Park, Y.-H. Kim, C. Wolf, C.-L. Lee, J. H. Heo, A. Sadhanala, N. Myoung, S. Yoo, S. H. Im, R. H. Friend and T.-W. Lee, Science, 2015, 350, 1222–1225 CrossRef CAS PubMed
.
- D. Yang, G. Zhang, R. Lai, Y. Cheng, Y. Lian, M. Rao, D. Huo, D. Lan, B. Zhao and D. Di, Nat. Commun., 2021, 12, 4295 CrossRef CAS PubMed
.
- P. P. Shi, Y. Y. Tang, P. F. Li, W. Q. Liao, Z. X. Wang, Q. Ye and R. G. Xiong, Chem. Soc. Rev., 2016, 45, 3811–3827 RSC
.
- H. Y. Zhang, C. L. Hu, Z. B. Hu, J. G. Mao, Y. Song and R. G. Xiong, J. Am. Chem. Soc., 2020, 142, 3240–3245 CrossRef CAS PubMed
.
- Y.-Y. Zhang, J.-Q. Luo, Y. Han, W.-Y. Zhang, Y. Zhang, H.-F. Lu and D.-W. Fu, Chin. Chem. Lett., 2024 DOI:10.1016/j.cclet.2024.109530
.
- Y.-Y. Chen, C.-H. Gao, T. Yang, W.-J. Li, H.-J. Xu and Z.-H. Sun, Chin. J. Struct. Chem., 2022, 41, 2204001 CAS
.
- J. Q. Luo, M. M. Lun, Q. Q. Jia, Z. J. Wang, H. F. Lu, Y. Zhang and D. W. Fu, Chin. J. Chem., 2024, 42, 1706–1712 Search PubMed
.
- W. Li, Z. Wang, F. Deschler, S. Gao, R. H. Friend and A. K. Cheetham, Nat. Rev. Mater., 2017, 2, 16099 CrossRef
.
- X. Xiao, W. Li, Y. Fang, Y. Liu, Y. Shao, S. Yang, J. Zhao, X. Dai, R. Zia and J. Huang, Nat. Commun., 2020, 11, 2215 CrossRef CAS PubMed
.
- Y. Yalcinkaya, I. M. Hermes, T. Seewald, K. Amann-Winkel, L. Veith, L. Schmidt-Mende and S. A. L. Weber, Adv. Energy Mater., 2022, 12, 2202442 CrossRef CAS
.
- E. Strelcov, Q. F. Dong, T. Li, J. Chae, Y. C. Shao, Y. H. Deng, A. Gruverman, J. S. Huang and A. Centrone, Adv. Sci., 2017, 3, e1602165 CrossRef PubMed
.
- Y. L. Zeng, X. Q. Huang, C. R. Huang, H. Zhang, F. Wang and Z. X. Wang, Angew. Chem., Int. Ed., 2021, 60, 10730–10735 CrossRef CAS PubMed
.
- X. Q. Huang, H. Zhang, F. Wang, T. Gan, Z. K. Xu and Z. X. Wang, J. Phys. Chem. Lett., 2021, 12, 5221–5227 CrossRef CAS PubMed
.
- M. M. Lun, C. Y. Su, J. Li, Q. Q. Jia, H. F. Lu, D. W. Fu, Y. Zhang and Z. X. Zhang, Small, 2023, 19, e2303127 CrossRef PubMed
.
- Y. He, Z. Chen, X. Chen, X.-M. Zhang and D. Fu, Mater. Chem. Front., 2022, 6, 1292–1300 RSC
.
- G. Teri, Q.-Q. Jia, Q. Guo, Y. Zhang and D.-W. Fu, Sci. China Mater., 2023, 66, 3687–3695 CrossRef CAS
.
- C. Y. Su, Z. X. Zhang, J. Yao, M. Chen, P. Z. Huang, Y. Zhang, D. W. Fu and L. Y. Xie, Chin. Chem. Lett., 2023, 34, 107442 CrossRef CAS
.
- M. Rok, M. Moskwa, J. Hetmańczyk, Ł. Hetmańczyk and G. Bator, CrystEngComm, 2022, 24, 4932–4939 RSC
.
- H. Peng, Q. Liu, Y. H. Liu, Y. Z. Lu and W. Q. Liao, Chin. Chem. Lett., 2023, 34, 107980 CrossRef CAS
.
- K. Ding, H. Ye, C. Su, Y. A. Xiong, G. Du, Y. M. You, Z. X. Zhang, S. Dong, Y. Zhang and D. W. Fu, Nat. Commun., 2023, 14, 2863 CrossRef CAS PubMed
.
- Q. Liu, H. Peng, J. C. Qi, Y. Z. Lu, S. J. Yang and W. Q. Liao, Chem. Commun., 2023, 59, 1793–1796 RSC
.
- Z. Yue, F. Wu, X. Li, Y. Liu, J. Luo and X. Liu, Sci. China Mater., 2023, 66, 3977–3983 CrossRef CAS
.
- M.-M. Lun, T. Zhang, C.-Y. Su, J. Li, Z.-X. Zhang, D.-W. Fu and H.-F. Lu, Mater. Chem. Front., 2022, 6, 1929–1937 RSC
.
- M. Rok, B. Zarychta, J. Trojan-Piegza, A. Bil, A. Piecha-Bisiorek, J. K. Zaręba, W. Medycki and R. Jakubas, J. Mater. Chem. C, 2022, 10, 3036–3047 RSC
.
- M.-Z. Li, Z.-H. Chen, S.-Q. Hu, J.-S. Zhou, L.-Y. Ji and X.-G. Chen, J. Mater. Chem. C, 2023, 11, 15952–15958 RSC
.
- Y. Zeng, J. Liu, L. Zhou, X. Deng, W. Yang, X. Yan, Y. Luo, X. Zhu, X. Huang, X. Song and Y. Tang, Chin. Chem. Lett., 2023, 34, 108127 CrossRef CAS
.
- K. Xu, Z. Zhou, J. Men, Q. Zhou and Q. Ye, Inorg. Chem. Front., 2023, 10, 5064–5070 RSC
.
- Y. J. Cao, L. Zhou, P. P. Shi, Q. Ye and D. W. Fu, Chem. Commun., 2019, 55, 8418–8421 RSC
.
- J. Mu, K. Xu, L. He, Y. Xu, T.-J. Yin, J.-T. Men and Q. Ye, Chem. Commun., 2023, 59, 1209–1212 RSC
.
- H. Peng, Z. K. Xu, Y. Du, P. F. Li, Z. X. Wang, R. G. Xiong and W. Q. Liao, Angew. Chem., Int. Ed., 2023, 62, e202306732 CrossRef PubMed
.
- Y. L. Zeng, Y. Ai, S. Y. Tang, X. J. Song, X. G. Chen, Y. Y. Tang, Z. X. Zhang, Y. M. You, R. G. Xiong and H. Y. Zhang, J. Am. Chem. Soc., 2022, 144, 19559–19566 CrossRef CAS PubMed
.
- J.-Q. Luo, Q.-Q. Jia, G. Teri, M.-M. Lun, Z.-J. Wang, Z.-X. Zhang, Y. Zhang and D.-W. Fu, ACS Mater. Lett., 2023, 452–460, DOI:10.1021/acsmaterialslett.3c01458
.
- R. G. Xiong, S. Q. Lu, Z. X. Zhang, H. Cheng, P. F. Li and W. Q. Liao, Angew. Chem., Int. Ed., 2020, 59, 9574–9578 CrossRef CAS PubMed
.
- H. Ye, W.-H. Hu, W.-J. Xu, Y. Zeng, X.-X. Chen, R.-K. Huang, W.-X. Zhang and X.-M. Chen, APL Mater., 2021, 9, 031102 CrossRef CAS
.
- D. W. Fu, J. X. Gao, W. H. He, X. Q. Huang, Y. H. Liu and Y. Ai, Angew. Chem., Int. Ed., 2020, 59, 17477–17481 CrossRef CAS PubMed
.
- Z. B. Hu, C. F. Wang, T. T. Sha, C. Shi, L. Ye, H. Y. Ye, Y. Song, Y. M. You and Y. Zhang, Small Methods, 2022, 6, e2200421 CrossRef PubMed
.
- Q. R. Meng, W. J. Xu, W. H. Hu, H. Ye, X. X. Chen, W. Yuan, W. X. Zhang and X. M. Chen, Chem. Commun., 2021, 57, 6292–6295 RSC
.
- C. Y. Su, Y. F. Yao, Z. X. Zhang, Y. Wang, M. Chen, P. Z. Huang, Y. Zhang, W. C. Qiao and D. W. Fu, Chem. Sci., 2022, 13, 4794–4800 RSC
.
- X. Liu, Z. Xu, P. Long, Y. Yao, C. Ji, L. Li, Z. Sun, M. Hong and J. Luo, Chem. Mater., 2020, 32, 8965–8970 CrossRef CAS
.
- K. Mencel, V. Kinzhybalo, R. Jakubas, J. K. Zaręba, P. Szklarz, P. Durlak, M. Drozd and A. Piecha-Bisiorek, Chem. Mater., 2021, 33, 8591–8601 CrossRef CAS
.
- M. Wojciechowska, A. Gągor, A. Piecha-Bisiorek, R. Jakubas, A. Ciżman, J. K. Zaręba, M. Nyk, P. Zieliński, W. Medycki and A. Bil, Chem. Mater., 2018, 30, 4597–4608 CrossRef CAS
.
- Z.-X. Zhang, C.-Y. Su, J. Li, X.-J. Song, D.-W. Fu and Y. Zhang, Chem. Mater., 2021, 33, 5790–5799 CrossRef CAS
.
- K. Mencel, P. Starynowicz, M. Siczek, A. Piecha-Bisiorek, R. Jakubas and W. Medycki, Dalton Trans., 2019, 48, 14829–14838 RSC
.
- P. Szklarz, A. Gagor, R. Jakubas, W. Medycki and G. Bator, Dalton Trans., 2023, 52, 11981–11991 RSC
.
- P. Szklarz, R. Jakubas, W. Medycki, A. Gagor, J. Cichos, M. Karbowiak and G. Bator, Dalton Trans., 2022, 51, 1850–1860 RSC
.
- M. Ksiądzyna, A. Gągor, A. Piecha-Bisiorek, A. Ciżman, W. Medycki and R. Jakubas, J. Mater. Chem. C, 2019, 7, 10360–10370 RSC
.
- M. Rowinska, A. Piecha-Bisiorek, W. Medycki, P. Durlak, R. Jakubas and A. Gagor, Molecules, 2023, 28, 3894 CrossRef CAS PubMed
.
- K. Aizu, Phys. Rev. B: Solid State, 1970, 2, 754–772 CrossRef
.
|
This journal is © The Royal Society of Chemistry 2024 |
Click here to see how this site uses Cookies. View our privacy policy here.