DOI:
10.1039/D3TC04752K
(Paper)
J. Mater. Chem. C, 2024,
12, 5578-5586
Ferroelectricity of alkylamide-substituted triptycene derivatives†
Received
24th December 2023
, Accepted 14th March 2024
First published on 19th March 2024
Abstract
1,8,13-Tris(dodecyloxy)triptycene forms uniform two-dimensional (2D) thin films by self-assembly. We synthesized new 1,8,13- and 1,8-substituted triptycene derivatives in which the alkoxy chains were replaced with alkylamide chains capable of hydrogen-bonding and investigated their phase transition behaviors, molecular assembly structures, and dielectric properties. In the three-chain compounds, the formation of a tightly packed 2D structure dominated by one-dimensional (1D) intermolecular N–H⋯O
hydrogen bonds was observed. In the two-chain compounds, the molecular arrangement and hydrogen bonding patterns were different from those of the three-chain molecules due to the decrease in the occupied volume of the alkyl chains and molecular symmetry, and the disorder in the molecular arrangement increased in the hydrophobic moiety. Both compounds exhibit solid-to-solid and solid-to-liquid phase transitions without forming a liquid-crystalline phase. In the temperature- and frequency-dependent dielectric constants, thermally activated dynamics of the polar amide groups with increasing temperature was observed at low frequencies, accompanied by a hysteresis in the polarization–electric field (P–E) curve above 400 K, which is a characteristic of ferroelectrics.
Introduction
Since the structures and physical properties of molecular crystals are governed by intermolecular interactions on various energy scales, controlling the arrangement of the components by considering the manner of intermolecular interactions is essential in developing functions for molecular assemblies. For example, organic π-molecules tend to adopt one-dimensional (1D) π-stacked structures, resulting in electrically conducting crystals, and exhibit metal–insulator transitions, due to the instability of their 1D electronic systems and carrier trapping by impurities.1–4 In order to suppress the phase transition to the insulator at low temperatures, the formation of a two-dimensional (2D) molecular arrangement has attracted attention from the viewpoint of stabilizing the metallic state, realizing superconducting and high carrier transport properties.5–10 On the other hand, the 2D lamellar phases formed by amphiphilic organic molecules have been known to form Langmuir–Blodgett films.11,12 In addition, a variety of functional 2D organic–inorganic hybrid perovskites have been developed by designing organic cations to achieve physical properties such as ferroelectricity, opto-electric responses, magnetism, and thermal conductivity.13–16
An interesting π-skeleton capable of forming 2D molecular arrays by self-assembly has been developed using triptycene.17–19 For instance, 1,8,13-tris(dodecyloxy)triptycene, in which three dodecyl ether chains are introduced at the 1, 8, and 13-positions of triptycene, can form 2D thin films on a variety of substrate surfaces.17 The 2D arrangement of the triptycene moieties with three-fold symmetry provides the molecular thin films with excellent stability and flexibility via the aid of multipoint van der Waals interactions between the alkyl chains. Applications of a 2D molecular layered structure to organic transistors and thermoplastic materials have already been reported.20–25 Thus, 1,8,(13)-substituted triptycene represents an interesting molecular motif for the design of organic functional materials featuring 2D structural elements.
The development of functional organic materials based on dynamic molecular assemblies has attracted much attention in recent years.26–32 For example, proton and ion dynamics in molecular assemblies facilitate ferroelectricity and ionic conductivity,33,34 and molecular rotational dynamics causes dielectric phase transitions and ferroelectricity.35,36 Among the structural units that can be used to realize diverse dynamics in solids, alkylamide chains (–CONHCnH2n+1) are the most promising unit, because of their hydrophobic interactions and intermolecular N–H⋯O
hydrogen bonding, both of which coexist in the molecular assemblies.37–39 The formation of discotic hexagonal columnar (Colh) liquid-crystalline phases has been reported for N,N′,N′′-tri(tetradecyl)-1,3,5-benzenetricrboxamide with three alkylamide chains at the 1, 3, and 5-positions of benzene, and ferroelectricity with reversal of the polarization direction has been reported in the Colh phase when an external electric field is applied.40–44 The inversion of polar intermolecular N–H⋯O
hydrogen bonds along the 1D chains can result in a macroscopic dipole inversion, which is the origin of ferroelectricity. The partial melting of alkyl chains in the molecular assembly plays an important role in the inversion of intermolecular amide hydrogen bonds even in the solid state.45 The π-electron cores at the molecular center have been utilized for pyrene,46,47 tetrabenzoporphyrin,48 and azobenzene,49 in addition to benzene rings, and offer a lot of design freedom. 1D ferroelectrics can be formed by the formation of π-stacks and intermolecular N–H⋯O
hydrogen bonds. The introduction of alkylamide chains into nonplanar π-electron compounds such as helicenes tends to form 2D hydrogen-bonding networks, increasing the values of the physical properties in ferroelectrics, remanent polarization (Pr), and coercive electric field (Ec), compared to materials featuring 1D hydrogen-bonding chains.50 Furthermore, the chiral design of intermolecular amide hydrogen-bonding chains plays an important role in controlling the physical properties of ferroelectrics by utilizing the dynamics of the molecules.51,52
Triptycene is an interesting research target as a π-core to introduce multiple alkylamide chains. In previous studies, introduction of alkylamide chains into disc-shaped π-molecules such as benzene and pyrene resulted in the formation of 1D columnar structures and 1D ferroelectrics. The introduction of alkylamide chains into triptycene has the potential to form molecular assemblies that differ from 1D structures. Since triptycene easily adopts a 2D π-interdigitated layered structure and forms a stable 2D arrangement by self-assembly of alkyl chains through van der Waals interactions, the formation of intermolecular hydrogen bonding through additional amide units is expected to affect the 2D layered structure. In this study, we synthesized N,N′,N′′-tritetradecyl-1,8,13-triptycenetricarboxamide (C14TATP) and N,N′-ditetradecyl-1,8-triptycenedicarboxamide (C14DATP) and investigated their phase transition behaviors, assembly structures, and dielectric properties and succeeded in revealing C14TATP shows a tightly packed 2D structure dominated by one-dimensional (1D) intermolecular N–H⋯O
hydrogen bonds and ferroelectricity by the inversion of the amide group in the solid phase (Scheme 1). The reduction in the number of alkyl chains in C14DATP produced randomness in the packing structure, which affected the dielectric response and ferroelectricity.
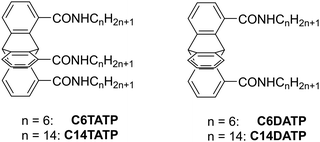 |
| Scheme 1 Molecular structures of CnTATP and CnDATP derivatives with n = 6 and 14 in this study. | |
Results and discussion
Phase transition behaviors
C14TATP and C14DATP were synthesized from 1,8,13-tricarboxytriptycene53 and 1,8-dichlorotriptycene,54 respectively (Experimental section in the ESI†). The thermal stabilities of C14TATP and C14DATP were expected to depend on the number of tetradecylamide chains that provide the strongest intermolecular interaction in the assemblies. TG measurements showed that C14TATP and C14DATP start to decompose at 600 and 500 K, respectively, and the thermal stability of the former is 100 K higher than that of the latter (Fig. S1, ESI†), due to the larger number of structural units that can contribute to intermolecular hydrogen bonding. Fig. 1a shows the DSC charts of C14TATP and C14DATP. Both compounds exhibit a reversible phase-transition behavior in multiple heating/cooling cycles. For C14DATP, a solid-to-solid phase transition from a lower temperature solid phase (S1) to another solid phase (S2) was observed at 292 K, and upon heating, S2 underwent a solid-to-isotropic liquid (IL) phase transition at 448 K. The transition enthalpy change (ΔH) for the S1–S2 phase transition was 22.7 kJ mol−1, which was 65% smaller than that for the S2-IL phase transition (ΔH = 34.9 kJ mol−1).
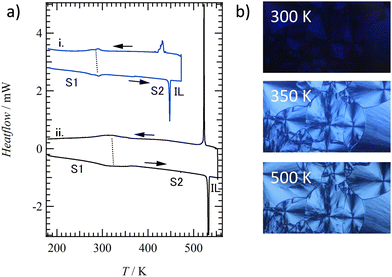 |
| Fig. 1 Phase-transition behaviors. (a) DSC charts of C14DATP (blue i) and C14TATP (black ii) in the second heating cycle. (b) POM images of C14TATP at 300, 350, and 500 K. | |
A similar phase transition behavior was observed for the triple-chain compound, C14TATP, displaying S1–S2 and S2-IL phase transitions at 320 (ΔH = 30.9 kJ mol−1) and 532 K (ΔH = 52.5 kJ mol−1), respectively. Compared to C14DATP, the S2-IL phase transition temperature for C14TATP increased by 92 K, which was due to the presence of an additional intermolecular hydrogen bonding site that allows for the stabilization of the ordered phase up to higher temperatures. As expected, ΔH accompanying the S2-IL phase transition for C14TATP is 1.5 times larger than that for C14DATP (ΔH = 34.9 kJ mol−1). Fig. 1b shows the change in the polarized optical microscopy (POM) image of C14TATP with increasing temperature. The S1 phase showed a dark field under a Cross-Nicol arrangement, whereas, upon the phase-transition into S2, the POM image turned bright with the emergence of a birefringent texture. This corresponds to a change in the domain orientation.
Crystal structure of C6TATP
Although, despite numerous trials, single crystals of C14TATP could not be obtained, those of C6TATP, suitable for X-ray crystallography, were successfully prepared by recrystallizing it from THF. However, the quality of the single crystals is not high enough to collect sufficient high-angle reflection data. Therefore, a detailed discussion of distances angles is not possible (Fig. S3, ESI†). Based on X-ray analysis, the crystal of C6TATP has a space group P21/c at 100 K, and the asymmetric unit in the unit cell consists of one C6TATP molecule (Experimental section in the ESI† and Fig. S4).55,56
Fig. 2a and b show the assembly structures of C6TATP viewed along the a- and c-axis, respectively. C6TATP molecules form a layered structure with a 2D arrangement in the bc plane, where the neighboring molecules are aligned in such a way that the longer molecular axis orientations are opposite to each other. Unlike the 2D lamellar structure observed for 1,8,13-tris(dodecyloxy)triptycene, an interdigitated molecular assembly between the triptycene moieties was not observed. On the other hand, intermolecular N–H⋯O
hydrogen bonding interactions were observed along the c-axis, where the intramolecular and intermolecular N–O distances (dN–O) were 2.847(2) Å, 2.802(8), and 2.749(7) Å, respectively. The intermolecular N–H⋯O
hydrogen bonds are stronger than intramolecular ones. Given that there are no effective intermolecular interactions along the a-axis that corresponds to the interlayer direction, the hydrogen-bonding interaction is considered to be the most effective intermolecular interaction in determining the packing structure of the molecular assembly.
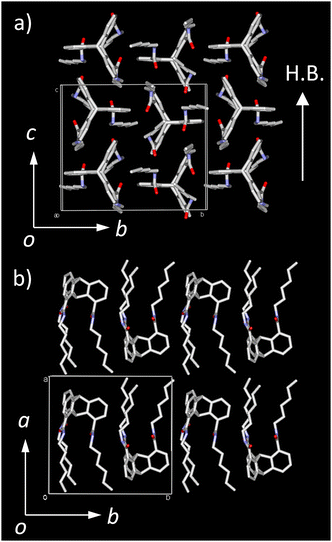 |
| Fig. 2 Crystal structure of C6TATP at 100 K. Unit cell viewed (a) along the a-axis and (b) along the c-axis. | |
Packing structures of C14TATP and C14DATP
To evaluate the molecular assembly structures of C14TATP and C14DATP, we carried out temperature-dependent PXRD experiments. Fig. 3a shows the temperature-dependent PXRD patterns of C14TATP and a simulated pattern obtained using the single crystal X-ray crystal data of C6TATP at 100 K. The diffraction peak of C6TATP at 2θ = 5.68° with a d-spacing of 15.56 Å arises from the stacking periodicity of the 2D layers, which corresponds to the a-axis length [15.8016(13) Å at 100 K] in the single crystal. For C14TATP, no significant differences in the PXRD patterns were observed between the S1 and S2 phases. At 300 K, diffraction patterns corresponding to a layer spacing of d = 25.5 Å appear at 2θ = 6.94 and 10.7°, which are assignable to 200 and 300 reflections of the a-axis for d200 = 12.73 and d300 = 8.261 Å. These are due to the stacking periodicity of the 2D layers. As the temperature was increased, the peak at 2θ = 21.7° with a d-spacing of 4.10 Å slightly shifts to become 2θ = 21.5° and 21.2° at 350 K and 400 K, respectively, and then broadens at 450 K. This broadening is the result of partial melting and thermal motion of the alkyl chains with increasing temperature.
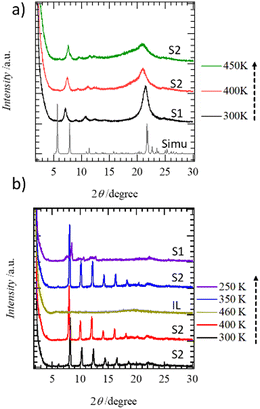 |
| Fig. 3 Temperature-dependent PXRD patterns of (a) C14TATP and (b) C14DATP. | |
Fig. 3b shows the temperature-dependent PXRD pattern of C14DATP with regular diffraction peaks appearing at 2θ = 4.09, 6.07, 8.11, 10.2, 12.2, 14.2. 16.3, and 18.4°. These can be well-explained by considering a layer periodicity with a d-spacing of 43.1 Å and correspond to correlation lengths of 1/2, 1/3, 1/4, 1/5, 1/6 1/8, and 1/9. Therefore, the molecular assembly of C14DATP has a 2D layered structure. We can now conclude that the dark-field POM images observed for the S1 and S2 phases are due to the formation of a homeotropic alignment perpendicular to the substrate surface, rather than randomly oriented domains. Notably, both C14TATP and C14DATP form 2D molecular assemblies with a layered lamellar structure, whereas the values of the layer periodicity (d = 25.5 and 43.1 Å for C14TATP and C14DATP, respectively) are very different from each other.
The molecular arrangements of C14TATP and C14DATP can be discussed in terms of 2D layered structures with different interlayer spacings. Assuming that the alkyl chains adopt an all-trans conformation, the maximum molecular lengths of C14TATP and C14DATP are estimated to be about 25 Å (Scheme 2a), with a thickness of about 7 Å for the triptycene moiety and an alkylamide chain length of about 18 Å. The molecular arrangement in C14TATP is expected to be dominated by 1D intermolecular N–H⋯O
hydrogen bonds, as observed for single-crystalline C6TATP. The neighboring C14TATP molecules are arranged in upper and lower orientations with respect to each other, allowing the formation of a closest-packed structure even when the alkyl chain length is elongated from hexyl to tetradecyl chains (Scheme 2b). The amide N–H⋯O
hydrogen bonds are formed in a direction parallel to the 2D layer. The molecular length of about 25 Å is in good agreement with an interlayer spacing of d = 25.5 Å found in the PXRD pattern.
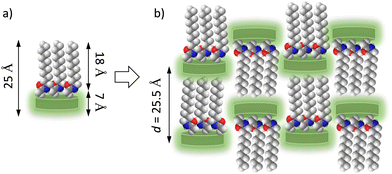 |
| Scheme 2 Possible packing structure of C14TATP. (a) Schematic molecule and (b) its assembly strucuture. | |
C14DATP having two tetradecylamide chains gives a longer lamellar periodicity of d = 43.1 Å than C14TATP, suggesting that its closest-packing structure is different from that of C14TATP. The size of the triptycene moiety is compatible in volume with the three alkyl chains, and the formation of an interdigitated structure of the triptycene moiety and the closest-packing of the alkyl chains has been reported for a tris(alkoxyl)-substituted triptycene derivative that realizes a 2D sheet-like structure. In the case of double-chain compounds such as C14DATP, a space to be occupied by the alkyl chains remains in contrast with that in C14TATP, and the molecular arrangement is different from that of triple-chain compounds such as C14TATP to maintain the closest-packing structure. Considering the lamellar periodicity of d = 43.1 Å observed in the PXRD pattern of C14DATP as well as the formation of intermolecular N–H⋯O
hydrogen bonds, a possible molecular arrangement can be proposed (Scheme 3). The lamellar periodicity (d = 43.1 Å) cannot be explained without assuming a dimer unit as a basic structure. It is reasonable to consider a dimer unit, in which the triptycene moieties interact in a face-to-face manner (left in Scheme 3a). The bilayer lamellar structure (d = 43.1 Å) can be explained if the alkyl chains are tilted about 54° with respect to the layer plane (Scheme 3b). Even in this case, intermolecular N–H⋯O
hydrogen bonds are possibly formed in the in-plane direction of the bilayer structure. However, when the packing density of the alkyl chains is reduced, it appears difficult to form a closest-packing structure as in the case of three alkyl chains with an all-trans conformation. Therefore, the conformational and packing patterns of the alkyl chains in the C14DATP assembly are expected to be different to the case of C14TATP.
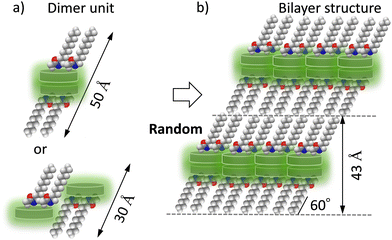 |
| Scheme 3 Possible packing structure of C14DATP. (a) Schematic dimer molecular units and (b) its assembly strucuture. | |
Temperature-dependent IR spectra
To evaluate the intermolecular N–H⋯O
hydrogen bonding interactions in the C14TATP and C14DATP assemblies, temperature-dependent IR spectra of KBr pellets were measured (Fig. 4). C14TATP and C14DATP exhibit S1–S2 phase transitions at 318 and 291 K, respectively. At 300 K, the asymmetrical stretching vibrational bands (νNH) of the amide unit of C14TATP appear at two different energies of 3258 and 3318 cm−1. The former νNH band is assigned to the intermolecular hydrogen-bonding amide groups, while the latter νNH band is assigned to the intramolecular hydrogen-bonding amide groups. The presence of amide groups in two different environments is consistent with the crystal structure of C6TATP. On the other hand, a broad νNH band is observed at 3299 cm−1 for the double-chain compound C14DATP at 300 K. The absence of νNH bands that are not involved in hydrogen bonding indicates that all the alkylamide chains in C14DATP contribute to intramolecular and intermolecular hydrogen-bonding interactions. The hydrogen-bonding νNH band for C14DATP is observed at higher energies than those for C14TATP, suggesting that a weaker hydrogen-bonding interaction is present in the former assembly.
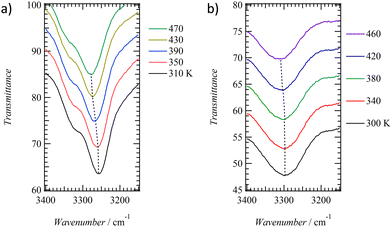 |
| Fig. 4 Temperature-dependent IR spectra (KBr) of (a) C14TATP and (b) C14DATP. | |
Upon increasing the temperature from the S1 phase at room temperature to the S2 phase, the energy of the νNH band in each compound shows a blue-shift. For C14TATP, the νNH band at 470 K is observed at 3279 cm−1, which is blue-shifted by approximately 20 cm−1 from that observed at 300 K. In contrast, the νNH band for C14DATP at 460 K is observed at 3279 cm−1, with a blue-shift of 12 cm−1 compared to that at 300 K. The changes in the FWHM of the νNH bands for C14TATP and C14DATP upon increasing the temperature from 300 to 470 K are +14 and 0 cm−1, respectively. The broadening of the νNH band for C14TATP is most likely due to an effect of randomness arising from thermal motion and disordering of hydrogen-bonding interactions. Therefore, the temperature increase results in thermal fluctuations in the N–H⋯O
hydrogen-bonding sites of C14TATP, leading to a change so that they can respond to external electric fields. On the other hand, given the fact that the νNH band of C14DATP remains almost intact with the temperature change and remains broad over a wide range including room temperature, and the N–H⋯O
hydrogen bonds with different strengths coexist in the assembly (Fig. S9, ESI†). We presume that in C14DATP having only two alkyl amide chains, a closest-packing of alkyl chains prevails over the formation of a structure with optimal hydrogen bonding between the amide groups, leading to randomness within the 2D structure and in turn the insensitivity of the νNH band to the temperature change.
Dielectric properties
Dielectric constants in molecular assemblies can vary in response to changes in the dipole moment of component molecules. The application of external electric fields to the C14TATP and C14DATP assemblies and/or temperature changes are expected to vary the orientation of amide groups involved in polar amide hydrogen bonds, resulting in a change in dielectric constants. Thus, we measured the temperature- and frequency-dependent real part dielectric constants (ε1) of C14TATP and C14DATP. According to the DSC charts (Fig. 1a), both compounds exhibit the S1–S2 phase transition near room temperature. As shown in Fig. 5a, C14TATP displays no dielectric anomaly at around the S1–S2 phase transition at 300 K. Consistent with the fact that C14TATP has no intermediate phase such as the liquid crystal and plastic crystal, its temperature-dependent dielectric response was monotonous, and the dielectric response associated with changes in the dipole moment of the amide group was only observed in the higher temperature region. A periodic N–H⋯O
hydrogen bonding and a closest-packed structure in the 2D plane of the three alkyl-chains in C14TATP induced a rigid crystal lattice and dielectric response only at high temperatures.
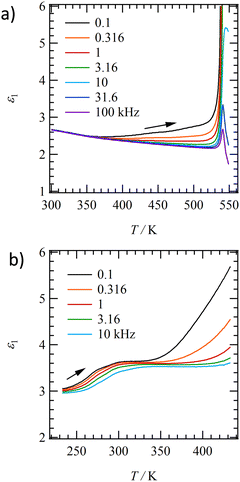 |
| Fig. 5 Temperature- and frequency-dependent ε1 of (a) C14TATP and (b) C14DATP. | |
The temperature- and frequency-dependent dielectric constants ε1 of C14DATP (Fig. 5b) are different from those of C14TATP. A frequency-dependent broad peak varying from ε1 ∼3 to 3.5 was observed around 290 K, where the S1–S2 phase transition of C14DATP occurs. Upon further heating, the ε1 value at a low frequency of 100 Hz increased from ∼350 K. Compared to C14TATP, the observed frequency- and temperature-dependence of C14DATP is more pronounced, and it can be concluded that the change in the dipole moment of C14DATP is greatly affected by the external field in the assembly. The imaginary part of the dielectric constant (ε2) shows a frequency-dependent broad peak near the S1–S2 phase transition point, which is the characteristic of a single-Debye type relaxation process for the dielectric response. The random 2D arrangement of two alkylamide chains in C14DATP reduced the crystallinity and uniformity of the intermolecular N–H⋯O
hydrogen-bonding network. The loose packing structure of C14DATP induced broad T- and f-dependent dipole responses. These results are consistent with the fact that the νNH band broadens around room temperature in the IR spectra of C14DATP.
P–E hysteresis curves
Fig. 6 shows the frequency-dependent polarization-electric field (P–E) curves and current-electric field (I–E) plots of C14TATP and C14DATP at 400 K. For both compounds, ferroelectric behavior with a P–E hysteresis was observed in the temperature range above 400 K, where the frequency-dependence is observed in the dielectric measurements. The effect of the leakage current on the P–E hysteresis of C14DATP near the melting point at 448 K becomes larger than that of C14TATP. The current (I)–E plots at 400 K show the I-maximum values corresponding to the polarization inversion of ferroelectricity. In addition, the symmetry in the ferroelectric phase is confirmed by SHG microscopy (Fig. S13, ESI†). The SHG activity of C14TATP is about 20% of that of granulated sugar at 298 K, supporting symmetry breaking. The fact that the sample shows SHG activity even when heated to 400 K is consistent with its ferroelectricity. In addition, SHG activity in the S2 phase is finely spread throughout the image. This corresponds to asymmetric, fine domains spread throughout in the molecule assembly structures. Similar P–E hysteresis curves have been observed in multiple alkylamide-substituted benzene derivatives that exhibit a Colh liquid-crystal phase.36–40
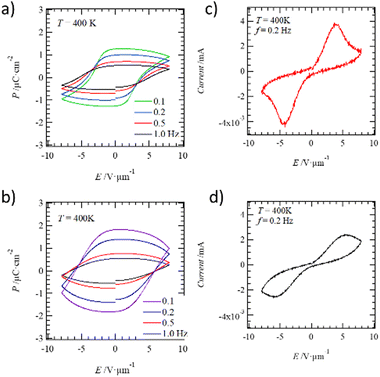 |
| Fig. 6 Frequency-dependent P–E hysteresis curves of (a) C14TATP and (b) C14DATP. I–E plots of (c) C14TATP and (d) C14DATP at T = 400 K and f = 0.2 Hz. | |
In C14TATP and C14DATP, ferroelectricity was also observed in the P–E curves in the 2D structure in the solid state. The inversion motion of the hydrogen-bonded alkylamide chains within the 2D structure due to the electric field resulted in the polarization inversion and ferroelectricity. The thermally induced partial melting of the alkyl chains plays an important role in the reversal motion of the polar amide groups in hydrogen-bonding states. The remanent polarization (Pr) and coercive electric field (Ec) of C14TATP at 400 K with f = 0.2 Hz were 0.99 μC cm−2 and 3.88 V μm−1, respectively, while those of C14DATP at 400 K with f = 0.2 Hz were 1.38 μC cm−2 and 5.82 V μm−1. The Pr value of C14DATP is slightly larger than that of C14TATP; however, the magnitude of Pr for both compounds are same to each other accompanying a difference of leakage current. The similarity in Pr between C14TATP and C14DATP can be accounted for a scenario that only two of the three alkylamide chains in the former are involved in hydrogen-bonding interactions, and thus their contribution to the magnitude of Pr is the same as in C14DATP. On the other hand, the Ec value of C14TATP was smaller than that of C14DATP. This is because the formation of regular intermolecular N–H⋯O
hydrogen bonds provides a constant barrier height for the dipole inversion. In the contrary, the disordered N–H⋯O
hydrogen-bonds in the 2D structure of C14DATP generates a random energy barrier for the dipole inversion, which is thought to be the result of the increase in Ec.
Conclusions
1,8,13-Tris(dodecyloxy)triptycene having three alkyl chains at the 1, 8, and 13-positions of triptycene is known to form a particular 2D structure due to a combination of the nested packing of the triptycene moiety and van der Waals interactions of the three alkyl chains. Inspired by this finding, we prepared hydrogen-bonding triptycene derivatives by introducing alkyl amide –CONHC14H29 chains instead of the –OC12H25 chains. The phase transition behavior, molecular assembly structure, dielectric response, and ferroelectricity of C14TATP with three –CONHC14H29 chains, as well as those of C14DATP with two –CONHC14H29 chains, were investigated to evaluate the effect of the number of alkylamide chains on the molecular assembly structure and physical properties. 1D N–H⋯O
hydrogen bonded chains were observed in both compounds, and ferroelectricity due to the inversion dynamics of the polar amide units was observed in the higher-temperature solid S2 phase above 400 K. Different dielectric responses between C14TATP and C14DATP were observed, and the dielectric response of the latter is dominated by the disordered packing of the alkylamide chains. The νNH band of C14DATP in the assembly was broadened compared to that of C14TATP, indicating the presence of disordered N–H⋯O
hydrogen bonding interactions. The P–E hysteresis was observed in the high-temperature S1 phase for both compounds, which is consistent with the presence of polarization inversion dynamics of the polar amide groups in the C14DATP assembly. The Pr values were comparable for both compounds, whereas the Ec values varied depending on the number of alkyl amide chains present. The intermolecular N–H⋯O
hydrogen bonds of C14DATP were more disordered than those of C14TATP, and the presence of structural randomness resulted in an increase in the polarization inversion energy. The design of alkylamide chains allows for the development of diverse ferroelectric molecular assemblies, and further design of π-electronic systems could lead to the creation of diverse multifunctional molecular materials.
Conflicts of interest
There are no conflicts to declare.
Acknowledgements
This work was supported by the Grant-in-Aid for Scientific Research on KAKENHI (Grant numbers: JP20H05865, JP22K19004, and JP20H05862 for T. A., and JP21H05024, JP21H04690, and JP20H05868 for Ta. F.), the Japan Science and Technology Agency, Core Research for Evolutional Science and Technology (Grant number: JPMJCR18I4), and the “Crossover Alliance to Create the Future with People, Intelligence and Material” project supported by the Ministry of Education, Culture, Sports, Science, and Technology.
Notes and references
- L. B. Coleman, M. J. Cohen, D. J. Sandman, F. G. Yamagishi, A. F. Garito and A. J. Heeger, Superconducting Fluctuations and the Peierls Instability in an Organic Solid, Solid State Commun., 1973, 12, 1125–1132 CrossRef CAS.
-
J. C. Scott, Semiconductor and Semimetals: High Conducting Quasi-One-Dimensional Organic Crystals, Academic Press, San Diego, 1988 Search PubMed.
- H. T. Nicolai, M. Kuik, G. A. H. Wetzelaer, B. Boer, C. Campbell, C. Risko, J. L. Brédas and P. W. M. Blom, Unification of Trap-Limited Electron Transport in Semiconducting Polymers, Nat. Mater., 2012, 11, 882–887 CrossRef CAS PubMed.
- O. Sachnik, X. Tan, D. Dou, C. Haese, N. Kinaret, K. H. Lin, D. Andrienko, M. Baumgarten, R. Graf, G. J. A. H. Wetzelaer, J. J. Michels and P. W. H. Blom, Elimination of Charge-Carrier Trapping by Molecular Design, Nat. Mater., 2023, 22, 1114–1120 CrossRef CAS PubMed.
- D. Jérome and H. J. Schulz, Organic Conductors and Superconductors, Adv. Phys., 1982, 31, 299–490 CrossRef.
- G. Saito and Y. Yoshida, Development of Conductive Organic Molecular Assemblies: Organic Metals, Superconductors, and Exotic Functional Materials, Bull. Chem. Soc. Jpn., 2007, 80, 1–137 CrossRef CAS.
- H. Ebata, T. Izawa, E. Miyazaki, K. Takimiya, M. Ikeda, H. Kuwabara and T. Yui, Highly Soluble [1]Benzothieno[3,2-b]benzothiophene (BTBT) Derivatives for High-Performance, Solution-Processed Organic Field-Effect Transistors, J. Am. Chem. Soc., 2007, 129, 15732–15733 CrossRef CAS PubMed.
- H. Minemawari, M. Tanaka, S. Tsuzuki, S. Inoue, T. Yamada, R. Kumai, Y. Shimoi and T. Hasegawa, Enhanced Layered-Herringbone Packing Due to Long Alkyl Chain Substitution in Solution-Processable Organic Semiconductors, Chem. Mater., 2017, 29, 1245–1254 CrossRef CAS.
- Z. F. Yao, J. Y. Wang and J. Pei, Control of π–π Stacking via Crystal Engineering in Organic Conjugated Small Molecule Crystals, Cryst. Grow. Des., 2018, 18, 7–15 CrossRef CAS.
- H. Abe, A. Kawasaki, T. Takeda, N. Hoshino, W. Matsuda, S. Seki and T. Akutagawa, Crystal Lattice Design of H2O-Tolerant n-Type Semiconducting Dianionic Naphthalenediimide Derivatives, J. Am. Chem. Soc., 2021, 143, 1046–1060 CrossRef CAS PubMed.
- B. K. Blodgett, Films Built by Depositing Successive Monomolecular Layers on a Solid Surface, J. Am. Chem. Soc., 1935, 57, 1007 CrossRef.
- O. N. Oliveira, L. Caseli and K. Ariga, The Past and the Future of Langmuir and Langmuir–Blodgett Films, Chem. Rev., 2022, 122, 6459–6513 CrossRef PubMed.
- W. Q. Liao, Y. Zhang, C. L. Hu, J. G. Mao, H. Y. Ye, P. F. Li, S. D. Huang and R. G. Xiong, A Lead-Halide Perovskite Molecular Ferroelectric Semiconductor, Nat. Commun., 2015, 6, 7338 CrossRef PubMed.
- Z. Sun, X. Liu, T. Khan, C. Ji, A. M. Asghar, S. Zhao, L. Li, M. Hong and J. Luo, A Photoferroelectric Perovskite-Type Organometallic Halide with Exceptional Anisotropy of Bulk Photovoltaic Effects, Angew. Chem., Int. Ed., 2016, 55, 6545–6550 CrossRef CAS PubMed.
- K. Taniguchi, M. Nishio, N. Abe, P. J. Huang, S. Kimura, T. Arima and H. Miyasaka, Magneto-Electric Directional Anisotropy in Polar Soft Ferromagnets of Two-Dimensional Organic–Inorganic Hybrid Perovskites, Angew. Chem., Int. Ed., 2021, 60, 14350–14354 CrossRef CAS PubMed.
- N. Hoshino, S. Tamura and T. Akutagawa, Negative-to-Positive Thermal Conductivity Temperature Coefficient Transition Induced by Dynamic Fluctuations of the Alkyl Chains in the Layered Complex (C4H9NH3)2CuCl4, Chem. – Eur. J., 2020, 26, 2610–2618 CrossRef CAS PubMed.
- N. Seiki, Y. Shoji, T. Kajitani, F. Ishiwari, A. Kosaka, T. Hikima, M. Takata, T. Someya and T. Fukushima, Rational Synthesis of Organic Thin Films with Exceptional Long-Range Structural Integrity, Science, 2015, 348, 1122–1126 CrossRef CAS PubMed.
- F. K. C. Leung, F. Ishiwari, T. Kajitani, Y. Shoji, T. Hikima, M. Takata, A. Saeki, S. Seki, Y. M. A. Yamada and T. Fukushima, Supramolecular Scaffold for Tailoring the Two-Dimensional Assembly of Functional Molecular Units into Organic Thin Films, J. Am. Chem. Soc., 2016, 138, 11727–11733 CrossRef CAS PubMed.
- F. Ishiwari, G. Nascimbeni, E. Sauter, H. Tago, Y. Shoji, S. Fujii, M. Kiguchi, T. Tada, M. Zharnikov, E. Zojer and T. Fukushima, Triptycene Tripods for the Formation of Highly Uniform and Densely Packed Self-Assembled Monolayers with Controlled Molecular Orientation, J. Am. Chem. Soc., 2019, 141, 5995–6005 CrossRef CAS PubMed.
- T. Yokota, T. Kajitani, R. Shidachi, T. Tokuhara, M. Kaltenbrunner, Y. Shoji, F. Ishiwari, T. Sekitani, T. Fukushima and T. Someya, A Few-Layer Molecular Film on Polymer Substrates to Enhance the Performance of Organic Devices, Nat. Nanotechnol., 2018, 13, 139–144 CrossRef CAS PubMed.
- M. Kondo, T. Kajitani, T. Uemura, Y. Noda, F. Ishiwari, Y. Shoji, T. Araki, S. Yoshimoto, T. Fukushima and T. Sekitani, Highly-Ordered Triptycene Modifier Layer Based on Blade Coating for Ultraflexible Organic Transistors, Sci. Rep., 2019, 9, 9200 CrossRef PubMed.
- M. Kondo, T. Uemura, F. Ishiwari, T. Kajitani, Y. Shoji, M. Morita, N. Namba, Y. Inoue, Y. Noda, T. Araki, T. Fukushima and T. Sekitani, Ultralow-Noise Organic Transistors Based on Polymeric Gate Dielectrics with Self-Assembled Modifiers, ACS Appl. Mater. Interfaces, 2019, 11, 41561–41569 CrossRef CAS PubMed.
- M. Sugiyama, S. Jancke, T. Uemura, M. Kondo, Y. Inoue, N. Namba, T. Araki, T. Fukushima and T. Sekitani, Mobility Enhancement of DNTT and BTBT Derivative Organic Thin-Film Transistors by Triptycene Molecule Modification, Org. Electron., 2021, 96, 106219 CrossRef CAS.
- F. Ishiwari, G. Okabe, H. Ogiwara, T. Kajitani, M. Tokita, M. Takata and T. Fukushima, Terminal Functionalization with a Triptycene Motif that Dramatically Changes the Structural and Physical Properties of an Amorphous Polymer, J. Am. Chem. Soc., 2018, 140, 13497–13502 CrossRef CAS PubMed.
- Y. Chen, F. Ishiwari, T. Fukui, T. Kajitani, H. Liu, X. Liang, K. Nakajima, M. Tokita and T. Fukushima, Overcoming the Entropy of Polymer Chains by Making a Plane with Terminal Groups: a Thermoplastic PDMS with a Long-Range 1D Structural Order, Chem. Sci., 2023, 14, 2431–2440 RSC.
- T. Akutagawa, Chemical Design and Physical Properties of Dynamic Molecular Assemblies, Bull. Chem. Soc. Jpn., 2021, 94, 1400–1420 CrossRef CAS.
- T. Akutagawa, T. Takeda and N. Hoshino, Dynamics of Proton, Ion, Molecule, and Crystal Lattice in Functional Molecular Assemblies, Chem. Commun., 2021, 57, 8378–8401 RSC.
- T. Akutagawa, H. Koshinaka, D. Sato, S. Takeda, S. Noro, H. Takahasi, R. Kumai, Y. Tokura and T. Nakamura, Ferroelectricity and Polarity Control in Solid State Flip-Flop Supramolecular Rotators, Nat. Mater., 2009, 8, 342–347 CrossRef CAS PubMed.
- T. Takeda, M. Ozawa and T. Akutagawa, Jumping Crystal of a Hydrogen-Bonded Organic Framework Induced by the Collective Molecular Motion of a Twisted π System, Angew. Chem., Int. Ed., 2019, 58, 10345–10352 CrossRef CAS PubMed.
- D. W. Fu, H. L. Cai, Y. Liu, Q. Ye, W. Zhang, Y. Zhang, X. Y. Chen, G. Giovannetti, M. Capone, J. Li and R. G. Xiong, Diisopropylammonium Bromide is a High-Temperature Molecular Ferroelectric Crystal, Science, 2013, 339, 425–428 CrossRef CAS PubMed.
- S. Furukawa, J. Wu, M. Koyama, K. Hayashi, N. Hoshino, T. Takeda, Y. Suzuki, J. Kawamata, M. Saito and T. Akutagawa, Ferroelectric Columnar Assemblies from the Bowl-to-Bowl Inversion of Aromatic Cores, Nat. Commun., 2021, 12, 768 CrossRef CAS PubMed.
- H. Y. Liu, H. Y. Zhang, X. G. Chen and R. G. Xiong, Molecular Design Principles for Ferroelectrics: Ferroelectrochemistry, J. Am. Chem. Soc., 2020, 142, 15205–15218 CrossRef CAS PubMed.
- T. Akutagawa, S. Takeda, T. Hasegawa and T. Nakamura, Proton-Transfer and Dielectric Phase Transition in Molecular Conductor (HDABCO+)2(TCNQ)3, J. Am. Chem. Soc., 2004, 126, 291–294 CrossRef CAS PubMed.
- G. Yuan, Y. Kimura, T. Kobayashi, T. Takeda, N. Hoshino and T. Akutagawa, Ion Polarisation-Assisted Hydrogen-Bonded Ferroelectrics in Liquid Crystalline Domains, Chem. Sci., 2021, 12, 13520–13529 RSC.
- X. Chen, X. Han and D. Q. Shen, PVDF-Based Ferroelectric Polymers in Modern Flexible Electronics, Adv. Electron. Mater., 2017, 3, 1600460 CrossRef.
- J. Ichikawa, N. Hoshino, T. Takeda and T. Akutagawa, Collective In-plane Molecular Rotator Based on Dibromoiodomesitylene π-Stacks, J. Am. Chem. Soc., 2015, 137, 13155–13160 CrossRef CAS PubMed.
- Y. Matsunaga, M. Miyajima, Y. Nakayasu, S. Sakai and M. Yonenaga, Design of Novel Mesomorphic Compounds: N,N′,N′′-Trialkyl-1,3,5-benzenetricarboxamides, Bull. Chem. Soc. Jpn., 1988, 61, 207–210 CrossRef CAS.
- T. F. A. D. Greef, M. M. J. Smulders, M. Wolffs, A. P. H. J. Schenning, R. P. Sijbesma and E. W. Meijer, Supramolecular Polymerization, Chem. Rev., 2009, 109, 5687–5754 CrossRef PubMed.
- T. Takeda and T. Akutagawa, Chemical Design of Organic Ferroelectrics Using Dynamics of Alkylamide Chains, Chem. Commun., 2022, 58, 11898–11912 RSC.
- Y. Shishido, H. Anetai, T. Takeda, N. Hoshino, S. Noro, T. Nakamura and T. Akutagawa, Molecular Assembly and Ferroelectric Response of Benzenecarboxamides Bearing Multiple −CONHC14H29 Chains, J. Phys. Chem. C, 2014, 118, 21204–21214 CrossRef CAS.
- C. F. C. Fitié, W. S. C. Roelofs, M. Kemerink and R. P. Sijbesma, Remnant Polarization in Thin Films from a Columnar Liquid Crystal, J. Am. Chem. Soc., 2010, 132, 6892–6893 CrossRef PubMed.
- C. F. C. Fitié, W. S. C. Roelofs, P. C. M. M. Magusin, M. Wubbenhorst, M. Kemerink and R. P. Sijbesma, Polar Switching in Trialkylbenzene-1,3,5-tricarboxamides, J. Phys. Chem. B, 2012, 116, 3928–3937 CrossRef PubMed.
- I. Urbanaviciute, X. Meng, T. D. Cornelissen, A. V. Gorbunov, S. Bhattacharjee, R. P. Sijbesma and M. Kemerink, Tuning the Ferroelectric Properties of Trialkylbenzene-1,3,5-tricarboxamide (BTA), Adv. Electron. Mater., 2017, 3, 1600530 CrossRef.
- I. Urbanaviciute, S. Bhattacharjee, M. Biler, J. A. M. Lugger, T. D. Cornelissen, P. Norman, M. Linares, R. P. Sijbesma and M. Kemerink, Suppressing Depolarization by Tail Substitution in an Organic Supramolecular Ferroelectric, Phys. Chem. Chem. Phys., 2019, 21, 2069–2079 RSC.
- M. Kawana, R. Mizoue, T. Takeda, N. Hoshino and T. Akutagawa, Simple Molecular Ferroelectrics: N,N′-Dialkyl-terephthalamide Derivatives in the Solid Phase, J. Mater. Chem. C, 2022, 10, 4208–4217 RSC.
- H. Anetai, Y. Wada, T. Takeda, N. Hoshino, S. Yamamoto, M. Mitsuishi, T. Takenobu and T. Akutagawa, Fluorescent Ferroelectrics of Hydrogen-Bonded Pyrene Derivatives, J. Phys. Chem. Lett., 2015, 6, 1813–1818 CrossRef CAS PubMed.
- H. Anetai, K. Sambe, T. Takeda, N. Hoshino and T. Akutagawa, Nanoscale Effects in One-Dimensional Columnar Supramolecular Ferroelectrics, Chem. Eur. J., 2019, 25, 11233–11239 CrossRef CAS PubMed.
- J. Wu, T. Takeda, N. Hoshino, Y. Suzuki, J. Kawamata and T. Akutagawa, Ferroelectricity of a Tetraphenylporphyrin Derivative Bearing −CONHC14H29 Chains at 500 K, J. Phys. Chem. C, 2019, 123, 22439–22446 CrossRef CAS.
- J. Wu, Q. Zhu, T. Takeda, N. Hoshino and T. Akutagawa, Ferroelectricity of Hydrogen-Bonded Azobenzene Derivatives, ACS Appl. Electron. Mater., 2021, 3, 3521–3529 CrossRef CAS.
- H. Anetai, T. Takeda, N. Hoshino, H. Kobayashi, N. Saito, M. Shigeno, M. Yamaguchi and T. Akutagawa, Ferroelectric Alkylamide-Substituted Helicene Derivative with Two-Dimensional Hydrogen-Bonding Lamellar Phase, J. Am. Chem. Soc., 2019, 141, 2391–2397 CrossRef CAS PubMed.
- J. Wu, T. Takeda, N. Hoshino and T. Akutagawa, Ferroelectric Low-Voltage ON/OFF Switching of Chiral Benzene-1,3,5-tricarboxamide Derivative, J. Mater. Chem. C, 2020, 8, 10283–10289 RSC.
- J. Wu, T. Takeda, N. Hoshino and T. Akutagawa, Ferroelectrics Coupled with Uni-Directional Rotation in Liquid Crystals, J. Phys. Chem. C, 2022, 126, 3864–3871 CrossRef CAS.
- S. Das, G. Nascimbeni, R. O. Morena, F. Ishiwari, Y. Shoji, T. Fukushima, M. Buck, E. Zojer and M. Zharnikov, Porous Honeycomb Self-Assembled Monolayers: Tripodal Adsorption and Hidden Chirality of Carboxylate Anchored Triptycenes on Ag, ACS Nano, 2021, 15, 11168–11179 CrossRef CAS PubMed.
- M. E. Tauchert, T. R. Kaiser, A. P. V. Gçthlich, F. Rominger, D. C. M. Warth and P. Hofmann, Phosphonite Ligand Design for Nickel-Catalyzed 2-Methyl-3-butenenitrile Isomerization and Styrene Hydrocyanation, ChemCatChem, 2010, 2, 674–682 CrossRef CAS.
- Crystal structure: single crystal structure analysis software. Ver. 3.6, Rigaku corporation and molecular structure corporation, 2004.
-
G. M. Sheldrick, SHELX2014 Programs for Crystal Structure Analysis, Universitat Göttingen: Göttingen, Germany, 2014.
|
This journal is © The Royal Society of Chemistry 2024 |
Click here to see how this site uses Cookies. View our privacy policy here.