DOI:
10.1039/D4TB00961D
(Paper)
J. Mater. Chem. B, 2024,
12, 7519-7531
Sprayable, thermosensitive hydrogels for promoting wound healing based on hollow, porous and pH-sensitive ZnO microspheres†
Received
5th May 2024
, Accepted 19th June 2024
First published on 20th June 2024
Abstract
A solvothermal method and the subsequent heat treatment process were developed to fabricate hollow ZnO particles with hierarchical pores on a large scale. The as-obtained hollow, porous ZnO microspheres with tunable sizes, high specific surface areas, pH sensitivity, antibacterial properties, and high adsorption capacities showed significant advantages for drug delivery. Sprayable hydrogels containing hollow, porous ZnO microspheres and curcumin nanoparticles (CNPs) were prepared to accelerate wound healing. The water-dispersed CNPs promoted both the migration of fibroblasts and angiogenesis and an aqueous solution of Pluronic F127 (a temperature-sensitive phase-change hydrogel material) was shown to be an effective choice for medical dressings. The experimental data suggest that hollow, porous ZnO microspheres can be loaded with additional CNPs to achieve continuous long-term therapeutic effects.
1. Introduction
The skin is the largest organ of the human body, protecting various tissues and organs from physical, mechanical, and chemical damage and invasion by pathogenic microorganisms. When skin wounds occur, contact with pathogenic microorganisms such as bacteria, fungi, and viruses can cause wound infections.1–5 Bacterial infections remain a significant cause of morbidity and mortality in surgical patients.6 It is therefore important to prevent and treat wound infection to inhibit bacterial breeding in wounds.7,8 Medical dressings that cover wounds can replace damaged skin to act as a temporary barrier, protect wounds, and prevent or control wound infection during the process of wound healing.9,10 While dressings are used to cover wounds physically, antibiotics are used to treat bacterial infections at the wound site. However, the widespread nonstandard use of antibiotics has led to the emergence and evolution of drug-resistant bacteria.11–13 Improving the performance of wound dressings and rationally using antibacterial agents are therefore major research focuses in preventing and treating wound infection. Compared with antibiotics, inorganic antibacterial materials have good stability and simple structures and are less likely to induce bacterial resistance, which has led to them gradually becoming the primary materials for preventing wound infection.14–16 ZnO is a multifunctional semiconductor material with good chemical stability, thermal stability, and antimicrobial activity. In addition, ZnO has been listed as a safe zinc compound (21CFR182.8991) by the United States Food and Drug Administration. ZnO has been reported to have antibacterial activity against Gram-negative bacteria such as Pseudomonas aeruginosa and Escherichia coli (E. coli) and Gram-positive bacteria such as Bacillus subtilis and Staphylococcus aureus (S. aureus).17–21 In addition, the morphology and structure of ZnO are easy to control, and ZnO nanomaterials such as nanorods,22,23 nanoflowers,24,25 and microspheres26–29 have been widely used in biomedical applications. However, reports of hollow, porous ZnO drug carriers are limited. Therefore, the development of porous ZnO drug carriers with high drug loading could open further applications.
In addition to preventing bacterial infection, the use of necessary medicines can promote wound healing. Products based on plant extracts have been used in wound healing for centuries, and in recent years, increasing experimental evidence has supported their effectiveness.30–34 Curcumin has been widely reported to promote wound healing owing to its anti-inflammatory activity and its ability to promote cell migration and angiogenesis.35–38 However, owing to its lack of specificity and water solubility, curcumin has low bioavailability. To overcome this hurdle, water-dispersive curcumin nanoparticles have been developed.39–41 The addition of ZnO and curcumin to wound dressings is expected to accelerate wound healing.
The wound dressing material should be nontoxic, nonallergenic, and soft in texture. Studies have shown that a moist environment can promote wound healing. A moist environment is conducive to cell proliferation, differentiation, migration, and the retention of active substances in the exudate.42–44 In addition, wounds do not form scabs under wet conditions, which can prevent the recurrence of mechanical injury and reduce pain. Hydrogels have gradually emerged as ideal dressings because of their flexibility and high water content.45,46 Aqueous solutions of F127—a temperature-sensitive hydrogel—can respond quickly to changes in ambient temperature and realize the phase transition from solution to gel, which has biomedical, tissue engineering, and cell engineering applications.47–49 In controlled drug release in particular, the three-dimensional network structure of temperature-sensitive F127 hydrogels can encapsulate drugs to provide drug storage, which can increase drug loading.50,51 The drug is then released slowly with the degradation or dissolution of the gel in the body, which can prevent the first-pass effect of the drug and prolong the retention time of the drug, achieving continuous administration.
The ability of thermosensitive F127 hydrogels containing CNPs and porous ZnO microspheres to accelerate wound healing was therefore investigated. Scheme 1 outlines the preparation processes and use of the wound dressing. First, ZnO microspheres were allowed to adsorb CNPs and were then mixed with F127 hydrogels containing CNPs. Hollow, porous ZnO microspheres micron-sized porous materials with a high specific surface area, high positive surface charge, low density, and high adsorption capacity were effective for drug delivery and enhancing antibacterial function. The water-dispersed CNPs adsorbed well on the surface of the hollow, porous ZnO microspheres. The final hydrogel mixture containing ZnO microspheres and CNPs was sprayed onto the surface of the wounds using an airbrush, and the temperature of the skin caused the thermosensitive hydrogel to quickly coagulate. Before spraying, the thermosensitive hydrogel wound dressings were precooled to ensure homogeneous liquidity. Furthermore, the ability of the thermosensitive hydrogel to promote wound healing in vivo was investigated, and indicators such as wound healing, fibroblast migration, and angiogenesis were observed using digital imaging, fluorescence imaging, and histological staining. We anticipate those sprayable thermosensitive hydrogels will be of significant value in wound healing applications.
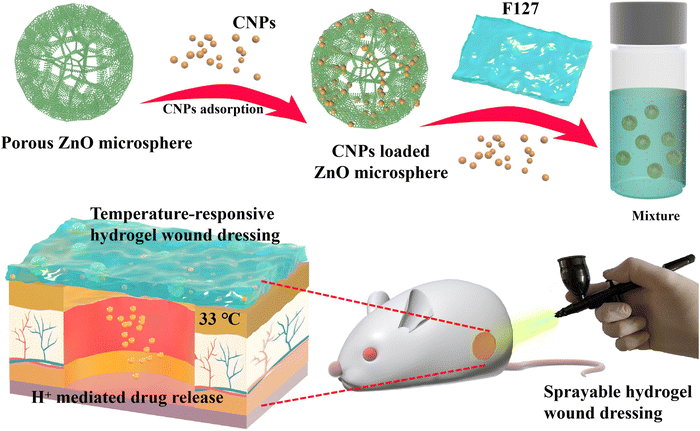 |
| Scheme 1 Preparation processes and usage of woud dressing. | |
2. Experimental section
Materials and reagents
All chemical reagents were of analytical grade and were used without further purification. Zinc nitrate hexahydrate, hexamine (HMTA), ammonium fluoride, trisodium citrate dihydrate, and tetrahydrofuran were obtained from China National Pharmaceutical Industry Corporation Ltd. Curcumin was purchased from Meryer Chemical Technology Co., Ltd. Calcein AM and Hoechst were purchased from Beyotime. A Cell Counting Kit-8 (CCK-8) was purchased from Beijing Labgic Technology Co., Ltd. Pluronic F127 was purchased from Sigma-Aldrich. We purchased mouse fibroblasts (L929) and human umbilical vein endothelial cells (HUVECs) from the China Academy of Sciences and cultured them in DMEM (Fisher, USA) supplemented with 1× penicillin–streptomycin (Fisher, USA) and 10% fetal bovine serum (Fisher, USA) at 37 °C in a humidified 5% CO2 standing temperature incubator.
Characterization
The morphology of the samples was characterized by field emission scanning electron microscopy (S-4800), scanning electron microscopy (FEI Quanta 250), and transmission electron microscopy (JEM-1400 Plus and JEM-2100). The X-ray diffraction (XRD) patterns were measured using an X-ray diffractometer (D/max 2550 V, Rigaku, Japan, Cu Kα (l = 0.154 nm) radiation at 40 kV and 200 mA). Fluorescence images were obtained by fluorescence microscopy (U-LH100HGAPO, Olympus Corporation). The concentration of the curcumin nanoparticles was measured on a UV-visible spectrophotometer (UV-9000, Aoyi Instruments Shanghai Co., Ltd). The average optical density (OD) was determined by a Universal Microplate Spectrophotometer (Synergy2 SLFPTAD, USA). ZnO microspheres and CNPs were measured with a Zetasizer IV analyzer (Malvern Zetasizer Nano ZS90, Malvern, UK) to determine their size and surface potential.
Preparation of porous and hollow ZnO microspheres
An equimolar ratio of zinc acetate dihydrate (25 mM) and HMTA (25 mM) was dissolved in 50 mL of deionized water, and 5 mM trisodium citrate and ammonium fluoride were then added and ultrasonically dispersed for 15 min at room temperature. After that, the mixture was transferred to a 100 mL Teflon-lined stainless steel autoclave and heated at 90 °C for 6 h. After cooling, the flower-like three-dimensional (3D) ZnO superstructures were collected by the natural sinking method, washed with distilled water several times, and dried in a freezer dryer for 48 h. Subsequently, the dried ZnO microspheres were heated from room temperature to 500 °C at a rate of 4 °C min−1, kept at 500 °C for 2 h, and then naturally cooled to room temperature.
Preparation of CNPs
First, 10 mg of curcumin was dissolved in 10 mL of THF. Then, the solution was slowly injected into 10 mL of deionized water under vigorous stirring at 500 rpm at room temperature in the dark for 48 h. Finally, the residual THF was removed by rotary evaporation.
Preparation of wound dressings containing ZnO@CNPs and CNPs
Pluronic F127 (19% (w/w)) in deionized water was placed in a refrigerator at 4 °C until it was fully dissolved. Hollow porous ZnO microspheres were added to a water dispersion of curcumin nanoparticles and shaken for 30 min. ZnO microspheres loaded with CNPs were separated by centrifugation twice. Finally, 50 mL of 19% Pluronic F127, 500 μg of ZnO microspheres loaded with CNPs, and 36 μg of CNPs were mixed and stored at 4 °C until use.
In vitro antibacterial test
The antibacterial activity of both the CNPs + F127 and CNPs + ZnO + F127 hydrogels against E. coli and S. aureus was evaluated using an inhibition zone assay. First, the density of E. coli and S. aureus was adjusted to 106 CFU mL−1, and then the bacterial suspension was spread on the agar surface for inoculation. The CNPs + F127 and CNPs + ZnO + F127 hydrogels were lyophilized by a freeze dryer and then squashed into wafers 6 mm in diameter by a tablet press. Afterward, the two wafers were placed on the center of agar plates and cocultured with E. coli and S. aureus for 48 h at 37 °C. The inhibition zone was measured visually by subtracting the diameter of each hydrogel from the diameter of the total inhibition zone.
The migration of fibroblasts
Fibroblast migration was evaluated by measuring cell expansion on the wound surface using a scratch assay. The cells were cultured in 6-well plates until they reached complete monolayer confluence. Then, a linear wound was generated using a sterile plastic pipette tip. The scattered fragments of cells were removed by washing with a fresh medium. Cells in the CNP group were treated without DMEM containing 0.72 μg mL−1 CNPs for 0, 24, 48, or 96 h. During the experiments, the cells were stained with Hoechst 33258 to visualize the cell nucleus.
Angiogenesis assay in vitro
Glass slides coated with Matrigel (Corning, 354
230, US) were placed in 96-well plates for cell culture. HUVECs were seeded in prepared 96-well plates at 3 × 105 mL−1 and cultured in a CO2 constant temperature incubator. The morphology was monitored under a light microscope every 2 h.
Drug-release measurement
The CNPs + F127–ZnO hydrogels were divided equally (2 mL) into three sample bottles. Then, 100 μL of saline was added to the three sample bottles at 0 h and 37 °C. Then, at 30 min, all the liquid was removed from the bottle at the indicated intervals, and the bottle was filled with 100 μL of saline. Subsequently, the concentration of the CNPs in the liquid was detected by a UV-visible spectrophotometer. The above procedures were repeated at 1.5 h, 3.5 h, 5.5 h, 7.5, 9.5 h, 23.5 h, 25.5 h, 27.5 h, and 29.5 h.
In vivo wound healing
Twenty six-week-old male BALB/c mice were chosen for the experiment. Wounds on the legs of the mice were created to evaluate the antibacterial and healing abilities of the hydrogels in vivo. The Shanghai Cancer Institute approved all the procedures involved in the animal experiments. The animals were randomly divided into four groups: the control, CNPs + F127 and CNPs + F127–ZnO–CNPs groups. Primarily, we shaved the fur of the legs of all the mice and then made full-thickness wounds. Afterward, the three hydrogels were loaded into a sterilized airbrush in advance and then sprayed and spread onto the wound site evenly. During all the animal experiments, the hydrogels were used for a total of one treatment. Furthermore, macroscopic images of the wounds were recorded by a digital camera, and the data were analyzed by ImageJ software at 0, 3, 6, 9, and 12 days after the operation. The rate of wound closure in the different groups at each time point was calculated by the following formula: healing rate (%) = (Ainitial
wound
area − Aresidual
wound
area
at
each
time
point)/(Ainitial
wound
area × 100).
Histological analysis
Individual wounds were excised, imaged, and fixed by immersion in 4% paraformaldehyde solution for 24 h. The fixed skin grafts were bisected and paraffin-embedded. Serial sections were obtained from each of the paraffin-embedded wounds using a microtome. Sections were collected and stained with H&E and Masson's trichrome stain according to the manufacturer's protocol. Images of the stained samples were captured by a light microscope.
3. Results and discussion
Porous ZnO microspheres are safe metal oxide antimicrobial agents that were also investigated as drug carriers in this study. A new preparation method for ZnO drug carriers was developed. The preparation process is illustrated in Fig. 1a. Uniform, porous, monodisperse microspheres were obtained using a simple solvothermal process. Owing to the introduction of NH4F, the chemical composition of the microspheres was Zn(OH)F rather than ZnO. The scanning electron microscopy (SEM) images in Fig. 1a–d show porous Zn(OH)F microspheres with diameters of 5–20 μm. The SEM images show numerous pores on the surface of the microspheres. These pores are interconnected and extend from the surface of the microsphere to the interior. The walls of the pores are thin and relatively smooth. Following heat treatment, Zn(OH)F decomposed into ZnO and HF. When transitioning to ZnO, the microspheres decreased in size. After HF was removed, smaller secondary pores were formed on the surface of the pore walls. The secondary pores can be seen in Fig. 1e–g.
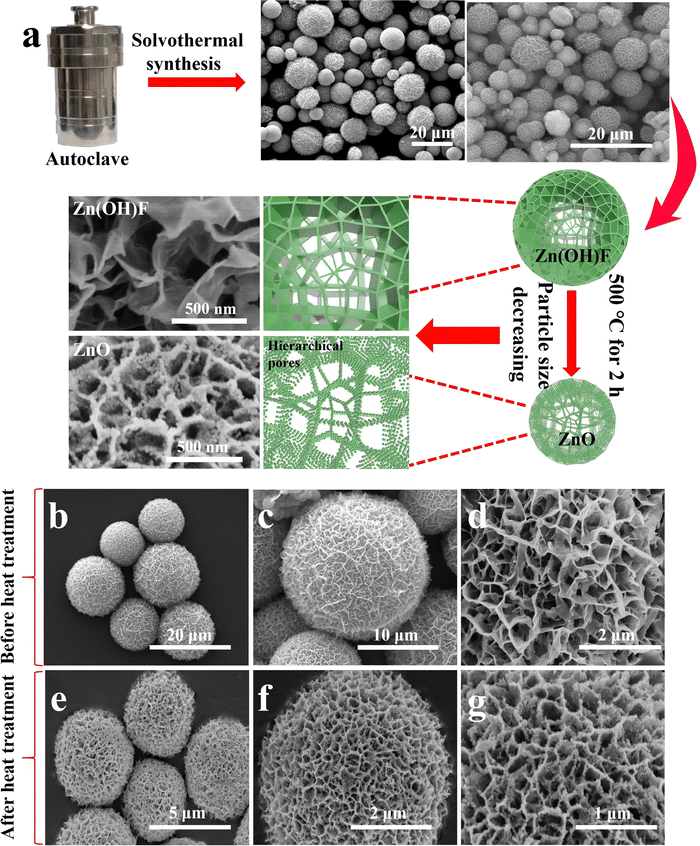 |
| Fig. 1 (a) Schematic diagram of the preparation of porous and hollow ZnO microspheres. (b)–(d) SEM images of Zn(OH)F microspheres. (e)–(g) SEM images of ZnO microspheres. | |
The TEM images in Fig. 2a, b, and Fig. S1 (ESI†) also show that heat treatment resulted in a large number of secondary pores, and Fig. 2a confirms the hollow structure. XRD analysis was carried out to investigate the composition of the microspheres. The XRD pattern of the microspheres before heat treatment is shown in Fig. 2c. Huang reported that zinc oxide preparation in the presence of NH4F resulted in Zn(OH)F.47Fig. 2c shows that the main diffraction peaks of Zn(OH)F centered at 2θ angles of 32.4, 33.7, and 35.5 formed one peak. As shown in Fig. 2d, after the microspheres were heated at 500 °C for 2 h, XRD analysis indicated that the hollow, porous microspheres were typical ZnO. The zeta potentials of the microspheres before and after heat treatment were determined to be −7.93 mV and 21.3 mV, respectively (Fig. 2e). The zeta potentials support the transition from Zn(OH)F to ZnO during heat treatment. ZnO microspheres have a high positive surface charge and are therefore expected to be excellent drug carriers. Sodium alginate, chitosan, and negatively charged drug molecules were effectively adsorbed onto the microspheres. Fig. S2 (ESI†) shows SEM images of the ZnO microspheres with adsorbed sodium alginate and chitosan. In addition, the adsorption capacity of the materials correlated with the specific surface area of the material. The N2 adsorption–desorption isotherms of the ZnO microspheres are shown in Fig. 2f. Owing to their hollow, porous structure, the ZnO microspheres had a high specific surface area of 50 m2 g−1 and a pore volume of 0.28 cm3 g−1.
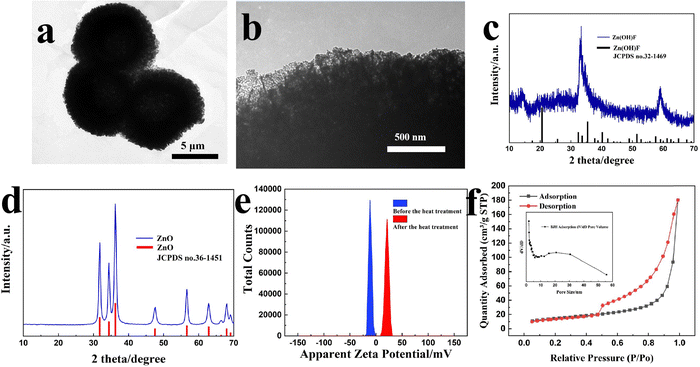 |
| Fig. 2 (a) and (b) TEM images of ZnO microspheres. (c) XRD pattern of the microspheres before heat treatment. (d) XRD pattern of the microspheres after heat treatment. (e) Zeta potential of the ZnO microspheres before and after heat treatment. (f) N2 adsorption–desorption isotherm and pore volume of the ZnO microspheres. | |
Hydrothermal reactions, special compositions, structures, condensed states, and particular morphologies have become increasingly important routes for accessing most inorganic functional materials. The hydrothermal reaction can be used to grow perfect single crystals with thermodynamic equilibrium defects, as well as controlled morphology and particle size, and can achieve ion doping during the synthetic reaction. The mechanism for the formation of hollow, porous Zn(OH)F microspheres was confirmed by SEM imaging. The structures formed in three stages: growth of small Zn(OH)F microspheres, self-assembly of small ZnO microspheres, and Ostwald ripening. The formation process is illustrated in Fig. 3a–c. In the second stage, small microspheres cluster together to form a hollow structure. The SEM images in Fig. 3d correspond to the second stage and show that the outlines of the small microspheres were clearly visible. The straightforward nature and tunability of the hydrothermal reaction are other advantages. Porous microspheres with different particle sizes were obtained by adjusting the concentration of the reactants and the composition of the solvent. When the concentration of the reactants was halved, the particle size decreased (Fig. 3e and f). When the concentration of the reactants was halved and the solvent was replaced with an equal volume mixture of water and PEG 200, the particle size decreased further (Fig. 3i and j). Smaller grain-size ZnO microspheres were then obtained through a simple heat treatment process (Fig. 3g, h, k and l).
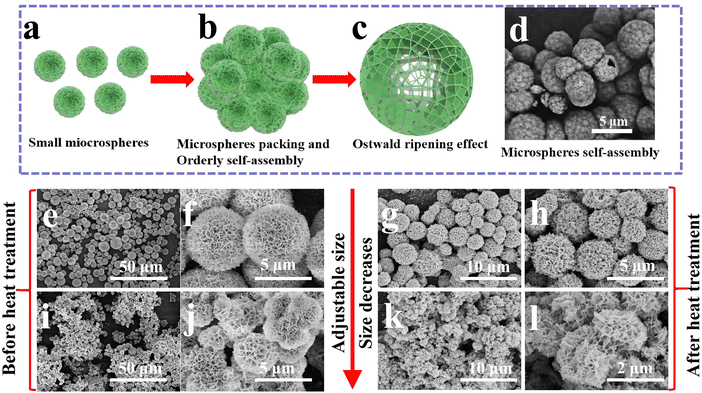 |
| Fig. 3 (a)–(d) Schematic diagrams illustrating the formation of porous and hollow Zn(OH)F microspheres. (e) and (i) SEM images of Zn(OH)F microspheres of different sizes. (f) and (j) are corresponding highly magnified images of (e) and (i), respectively. (g) and (k) SEM images of ZnO microspheres of different sizes. (h) and (l) are corresponding magnified images of (g) and (k), respectively. | |
Using carriers to load drugs is a common way of increasing drug efficacy. Among the many recently developed drug carriers, hollow, porous ZnO microspheres, which have a high specific surface area and positive surface charge, are theoretically ideal drug carriers. The positive charge of ZnO means that negatively charged drugs are easily absorbed. Curcumin, a negatively charged drug in water, was selected as a model to investigate drug adsorbability. Curcumin is difficult to apply clinically owing to its poor water solubility and bioavailability. CNPs were therefore prepared to improve the water solubility and bioavailability of curcumin. TEM imaging revealed that the CNPs were spherical with well-defined and monodisperse morphologies (Fig. 4a–c). The size of the CNPs was measured using dynamic laser light scattering (DLS). As shown in Fig. 4d, the average hydrodynamic diameter of the CNPs was 93 nm. Fig. S3 (ESI†) shows that CNPs at high concentrations were well dispersed in aqueous solution. The zeta potential of the CNPs was −26 mV. Therefore, the negatively charged curcumin nanoparticles could be effectively adsorbed on the surface of the positively charged zinc oxide microspheres. A high concentration of curcumin inhibited cell proliferation, while a low concentration of curcumin promoted cell proliferation. A biocompatibility assay using different concentrations of curcumin nanoparticles was carried out to determine the effect of the curcumin nanoparticle concentration. The data were quantified using a CCK-8 kit. The optical density (OD) values for the 0.72 μg mL−1 CNPs and the control were similar, and the differences were not statistically significant. The OD values increased over time, which suggested that the HEUVCs maintained good viability and continued to proliferate. The ODs of the groups in which the concentration of CNPs was greater than 0.72 μg mL−1 were lower than those of the control group, which suggested that high concentrations of CNPs inhibit cell proliferation. Therefore, 0.72 μg mL−1 CNPs were used in the wound healing experiments. ZnO microspheres were added to an aqueous solution of CNPs to test their adsorption properties. SEM images (Fig. 4g–i) showed that a large number of CNPs covered the surface of the ZnO microspheres. Pores on the surface of the ZnO microspheres were not observed due to the adsorption of the CNPs. UV-vis absorption spectra (Fig. S4 and S5, ESI†) showed that the adsorption capacity of the ZnO microspheres was 54.7 μg mg−1 (1 mg of ZnO microspheres adsorbed 54.7 μg of CNPs).
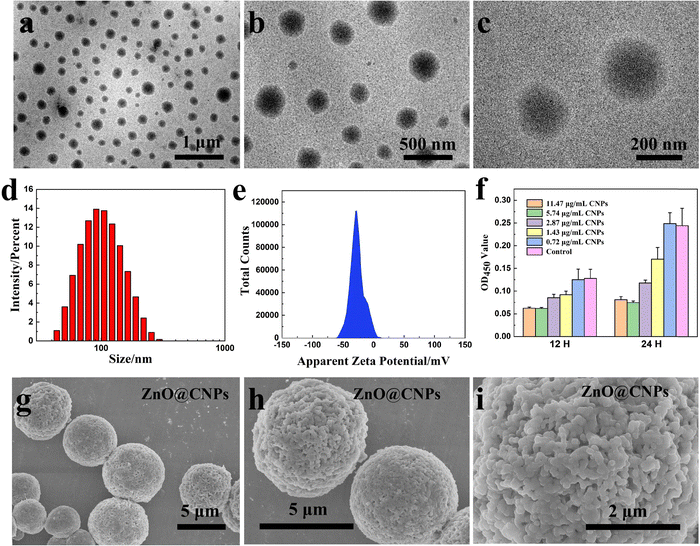 |
| Fig. 4 (a)–(c) TEM images of curcumin nanoparticles. (d) Size distribution of the as-prepared CNPs. (e) Zeta potential of the CNPs. (f) Quantification of the CCK-8 assay results for the different groups at 12 h and 24 h. (g)–(i) FE-SEM images of ZnO microspheres loaded with CNPs. | |
A hydrogel was used as a convenient drug delivery vehicle in the designed system. Hydrogels that form in situ can completely cover the wound area and are generally flowable liquids that rapidly change to gels when they contact physiological environments. For temperature-sensitive hydrogels used in wound healing, the body surface temperature is a native trigger. Pluronic F127, a macromolecular nonionic surfactant, has been reported as a drug carrier that enables the delivery of drugs, growth factors, and nucleic acids. In an aqueous solution, Pluronic F127 showed an obvious sol–gel transition and gel–sol transition. Under normal conditions, the surface temperature of human skin is greater than 21 °C; therefore, an F127 hydrogel with a phase transition temperature of 21 °C was prepared. When the temperature was greater than 21 °C, the aqueous solution transitioned to a gel state and returned to a solution state when the temperature fell below 21 °C (Fig. 5a). We placed the precooled ZnO + F127–CNPs in an airbrush and sprayed the hydrogel onto black cardboard. The cardboard was then placed on a heater, and the spraying process was recorded. As Fig. 5b shows, the sprayed hydrogel was even, with no evident wrinkles, and there was no disruption to the spray flow, which satisfied the design requirements. CNPs + ZnO + F127 transitioned from the sol state to the gel state very quickly when in contact with black cardboard. The letter A was written on the surface of the hydrogel and maintained its shape for an extended period, which showed that the CNPs + ZnO + F127 achieved the gel state. To verify the phase transition temperature of F127 with and without added components, rheological analysis was conducted at various temperatures. As the temperature increased, G′ and G′′ increased. The phase transition temperature was indicated by the point where G′ was equal to G′′. As shown in Fig. 5c, the phase transition temperatures of the three types of F127 hydrogels were approximately 21 °C. Although CNPs and ZnO microspheres were added to the F127 aqueous solution, the phase transition temperature showed no marked change. The morphology of the as-prepared F127 hydrogel was investigated by SEM, which showed uniform porous structures (Fig. 5d and e). To determine the treatment interval, drug release experiments were carried out in vitro. When 100 μL of water was added to the hydrogel, a small amount of the hydrogel dissolved. As additional water was added and the hydrogel gradually dissolved, the amount of curcumin released gradually increased. A simulated experiment showed that the drug was completely released within 48 h. A low level of immune defense and a high risk of bacterial infection often result in delayed wound healing. The anti-E. coli and anti-S. aureus activities of the CNPs + ZnO + F127 were determined using the agar diffusion assay method. Clear inhibition zones toward E. coli and S. aureus were formed by ZnO + F127 and CNPs + ZnO + F127 after 48 h. At 48 h, the zone diameters in the ZnO + F127 group reached 3.33 ± 0.57 mm and 2.33 ± 0.58 mm, respectively (Fig. 5g and h). However, the antibacterial zones in the CNPs + ZnO + F127 group were larger than those in the ZnO + F127 group (4.3 ± 0.58 mm and 3.3 ± 0.58 mm, respectively). These results indicate that CNPs + ZnO + F127 have a better antibacterial effect than does ZnO + F127. In contrast, CNPs + F127 showed no obvious antibacterial activity against E. coli or S. aureus.
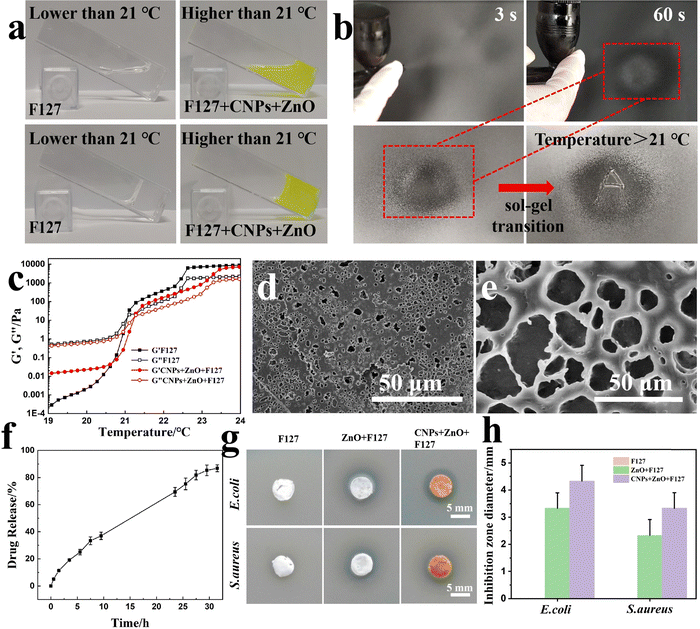 |
| Fig. 5 (a) Photographs of the F127 hydrogel and F127 with CNPs and ZnO microspheres at different temperatures. (b) Photographs of the hydrogel spraying process. (c) Rheological property test of G′ (shear modulus of the elastic component) and G′′ (shear modulus of the viscous component). (d) and (e) SEM images of mixed gels. (f) CNPs released from CNPs + ZnO + F127. (g) Antibacterial sensitivity of gels against E. coli and S. aureus determined by agar diffusion tests at 48 h. (h) Inhibition zone diameters of different groups for E. coli and S. aureus. | |
The ideal dressing should be antibacterial and promote cell migration and angiogenesis. An in vitro scratch assay was carried out on a fibroblast monolayer to monitor the effects of CNPs on wound closure over 72 h (Fig. 6a). After 24 h of exposure to CNPs, the cells moved toward the opening to close the wound faster than those in the control group. At 72 h, the wound had only partially healed in the control group, whereas the wound was almost completely closed in the CNP group. In addition to promoting cell migration, CNPs promoted the formation of new blood vessels. The impact of CNPs on angiogenesis in vitro was evaluated by tube formation assays, as vasculogenesis is a key factor in wound healing and accelerates the repair process. Fig. 6b shows that human umbilical vein endothelial cells (HUEVCs) treated with 0.72 μg mL−1 CNPs exhibited more junctions and longer tube lengths than did control cells. More importantly, over time, the tubes in the CNPs maintained a good shape. In the control group, the HUEVCs were unable to maintain a tubular shape when the HUEVC culture time reached 4 h. Therefore, based on these results, we concluded that ZnO and CNPs could help create new blood vessels.
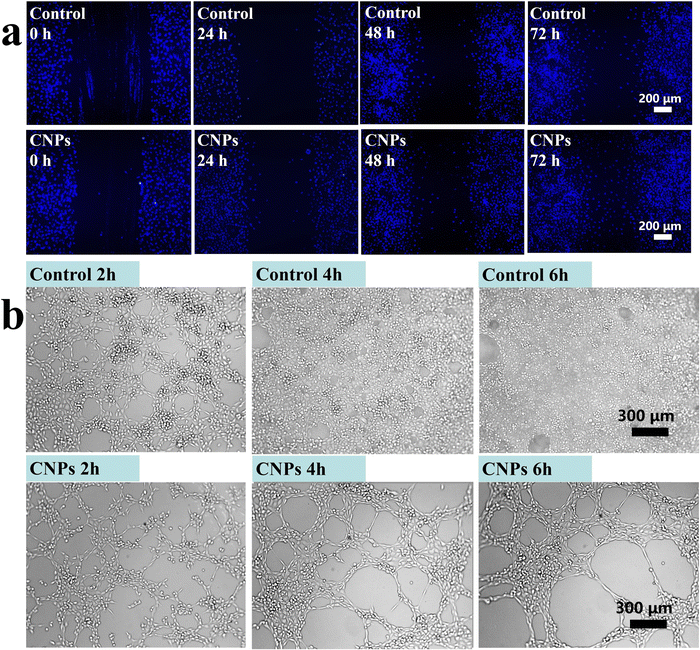 |
| Fig. 6 (a) Representative fluorescence microscopy images of scratches induced in L929 cells without any treatment and treated with CNPs for 0–72 h. Cells were probed with Hoechst 33342 for nuclear fluorescence staining. (b) In vitro vascular tube formation ability of HUVECs in the CNP and control groups at 2 h, 4 h and 6 h. | |
The wound healing efficiency of the F127, CNPs + F127, and CNPs + ZnO + F127 hydrogels was investigated in vivo by creating full-thickness excisional wounds. The healing results at different time points are shown in Fig. 7a–c. On day 3, a large amount of yellow pus was dispersed in the wound bed in the control, F127, and CNPs + F127 groups. By day 6, the amount of yellow pus in the control and F127 groups had decreased, but it was still observed, while there was no yellow pus in the CNPs + F127 and CNPs + ZnO + F127 groups. Very little yellow pus was observed for the CNPs + ZnO + F127 group throughout the whole experiment, which is attributed to the presence of ZnO. In addition, the wounds in the CNP + ZnO + F127 hydrogel-treated group appeared drier and smaller on day 3, which indicated that the infections had been effectively controlled and that the wound repair process was initiated. In the first 6 days, the wound healing area in the control, F127, and CNPs + F127 groups increased rapidly, which was attributed to the infection being controlled by the immune system. As a result of the antibacterial effect of ZnO and the release of CNPs, three days after surgery, 53% of the wounds in the CNPs + ZnO + F127 group were healed, whereas 8%, 14%, and 18% of the wounds in the control, F127 and CNPs + F127 groups were closed, respectively. After 6 days of healing, the wound closure of the CNPs + ZnO + F127-treated group was 63%, which was 9.4% greater than that of the CNPs + F127 group, 28% greater than that of the F127 group, and 40.3% greater than that of the control group. On day 9, the wounds had almost completely healed in the CNPs + ZnO + F127 group, while the percentages of wounds in the control, F127 and CNPs + F127 groups were 45.9%, 66.7%, and 69.4%, respectively. The wounds had not fully healed by day 12 in the control, F127, and CNPs + F127 groups. The in vivo healing results indicated that the incorporation of CNPs and ZnO@CNPs into F127 dressings accelerated wound healing, particularly initially.
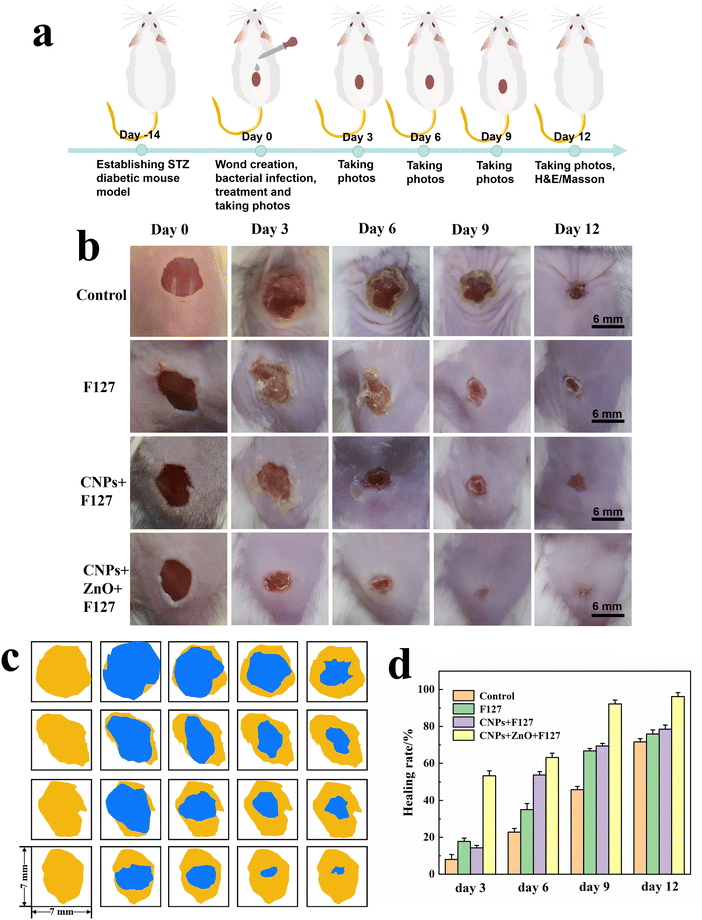 |
| Fig. 7 Infected diabetic wound healing studies in vivo. (a) The process of the animal experiment. (b) Photographs of wound tissues after different treatments on days 0, 3, 6, 9, and 12. (c) Wound healing boundaries in different groups in vivo. The yellow skin tone area indicates the initial wound area, and the blue area indicates the wound area at 3, 6, 9, and 12 days. (d) Wound healing rate in different groups at each time point. | |
Samples of the wounds at each time point were harvested and embedded in paraffin for hematoxylin and eosin (HE) and Masson's trichrome staining. Fig. 8a shows that there was essentially no continuous epidermis structure in the control and F127 groups after 12 days of treatment, while regenerated continuous epidermis was observed for the CNPs + F127 and CNPs + ZnO + F127 groups. As the wound heals, the epithelial thickness of the wound tissue decreases. A thick epidermal tissue structure formed in the CNPs + F127 group after 12 days. The thickness of the epidermal tissue structure decreased markedly in the CNPs + ZnO + F127 group over the same period and was similar to that of normal tissue. The CNPs + ZnO + F127 group had the thinnest wound epithelial tissue sections, suggesting a better epithelialization ability (Fig. 8c) and better promotion of wound healing. In addition, in the control and F127 groups, the wound defects were still obvious, with numerous neutrophils. The defect was almost closed in the CNPs + ZnO + F127 group after 12 days, and there were numerous hair follicles at the healing site, which showed almost no difference from normal tissue. In contrast, hair follicle tissue began to appear in the CNPs + F127 group but had not yet formed. Collagen deposition is an important marker for tissue repair. Masson's trichrome staining images showing the extent of collagen deposition are presented in Fig. 8b and d, with collagen stained blue. After 12 days of healing, the collagen deposition in the CNPs + ZnO + F127 group was much denser than that in the control, F127, and CNPs + F127 groups. In the CNPs + ZnO + F127 group, a large amount of collagen was distributed in a network near the epidermal tissue, which indicated that the wound had essentially healed. There was a large light blue area in the CNPs + F127 group, which indicates that the wound tissues were still in the process of repair. The least collagen deposition was observed for the control and F127 groups, indicating that the wounds in the control and F127 groups were the slowest to heal. Taken together, the above results indicate that CNPs + ZnO + F127 have outstanding wound repair properties, promote angiogenesis and migration of fibroblasts and accelerate the wound healing process.
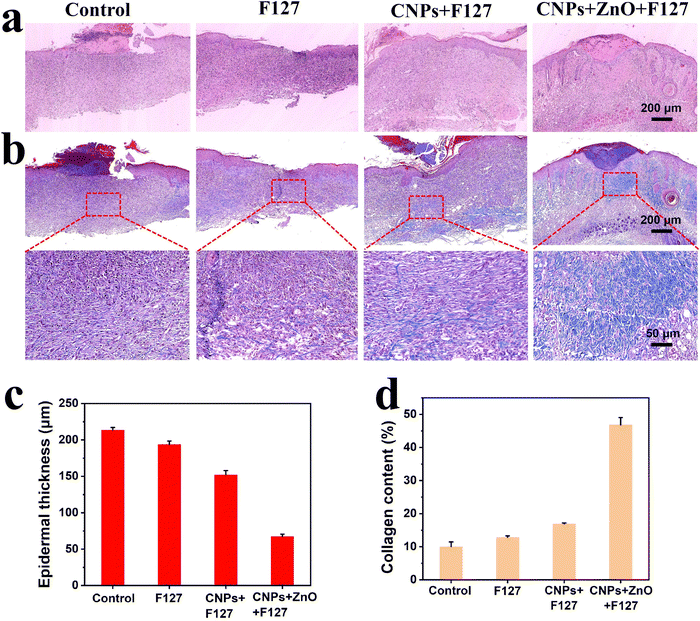 |
| Fig. 8 Histological analysis of wounds in the control, F127, CNPs + F127, and CNPs + ZnO + F127 groups. (a) HE-stained skin tissue sections from the control, F127, CNPs + F127, and CNPs + ZnO + F127 groups on day 12. (b) Masson-stained skin tissue sections from each group at 12 days. (c) Quantification of wound epithelial thickness in the different groups. (d) Quantification of wound collagen in the different groups. | |
4. Conclusions
We successfully prepared hollow, porous ZnO microspheres using a solvothermal method and subsequent heat treatment process. The as-obtained ZnO microspheres exhibited a high specific surface area and good adsorption properties. Based on the antibacterial effects and adsorption of the porous ZnO microspheres, a thermosensitive hydrogel dressing containing CNPs and ZnO@CNPs was designed for wound healing applications. Experimental and theoretical studies revealed that porous ZnO microspheres were excellent drug carriers and that hydrogels containing CNPs and ZnO@CNPs exhibited sprayable, antibacterial, and temperature-responsive properties, as well as the ability to promote angiogenesis and fibroblast migration. Animal experiments further demonstrated that hydrogels containing CNPs and ZnO@CNPs accelerated the wound-healing process. In addition, our prepared porous ZnO microspheres can theoretically adsorb other drugs and release them in a nonneutral environment. We therefore hope our work can be applied to the treatment of a wide range of diseases.
Data availability
All relevant data are within the manuscript and its additional files. The data are available from the corresponding author on reasonable request.
Conflicts of interest
The authors declare no competing financial interests.
Acknowledgements
This work was supported by the National Natural Science Foundation of China (82272829, 82372552), the Excellent Youth of Natural Science Research Projects in Anhui Province Universities (2023AH030060), the Basic and Clinical Cooperative Research and Promotion Program of Anhui Medical University (2021xkjT028), Research Fund of Anhui Institute of Translational Medicine (2022zhyx-C01), and Postdoctoral Research Activities in Anhui Province (2022B583). The authors would like to thank the Shiyan laboratory (https://www.shiyanjia.com) for their help in language polishing.
References
- W. Wang, Y. Cui, X. Wei, Y. Zang, X. Chen, L. Cheng and X. Wang, ACS Nano, 2024, 18(24), 15845–15863 CrossRef CAS PubMed.
- J. Shan, X. Jin, C. Zhang, M. Huang, J. Xing, Q. Li, Y. Cui, Q. Niu, X. L. Chen and X. Wang, Acta Pharm. Sin. B, 2024, 14, 2298–2316 CrossRef CAS PubMed.
- M. Zhao, M. Kang, J. Wang, R. Yang, X. Zhong, Q. Xie, S. Zhou, Z. Zhang, J. Zheng, Y. Zhang, S. Guo, W. Lin, J. Huang, G. Guo, Y. Fu, B. Li, Z. Fan, X. Li, D. Wang, X. Chen, B. Z. Tang and Y. Liao, Adv. Mater., 2024, 2401369 CrossRef PubMed.
- W. Gao, Y. Wang, Y. Zheng and X. Cai, Acc. Mater. Res., 2024 DOI:10.1021/accountsmr.3c00260.
- L. Chen, Y. Guo, L. Chen, K. Hu, L. Ruan, P. Li, X. Cai, B. Li, Q. Shou and G. Jiang, ACS Appl. Bio Mater., 2023, 6, 2184–2195 CrossRef CAS PubMed.
- S. He, M. Lin, Q. Zheng, B. Liang, X. He, Y. Zhang, Q. Xu, H. Deng, K. Fan and W. Chen, Adv. Healthcare Mater., 2024, 2303548 CrossRef PubMed.
- S. A. Ermolaeva, A. F. Varfolomeev, M. Y. Chernukha, D. S. Yurov, M. M. Vasiliev, A. A. Kaminskaya, M. M. Moisenovich, J. M. Romanova, A. N. Murashev, I. I. Selezneva, T. Shimizu, E. V. Sysolyatina, I. A. Shaginyan, O. F. Petrov, E. I. Mayevsky, V. E. Fortov, G. E. Morfill, B. S. Naroditsky and A. L. Gintsburg, J. Med. Microbiol., 2011, 60, 75–83 CrossRef CAS PubMed.
-
S. H. Feng and G. H. Li, in Modern Inorganic Synthetic Chemistry, ed. R. Xu and Y. Xu, Elsevier, Amsterdam, 2nd edn, 2017, pp. 73–104 DOI:10.1016/B978-0-444-63591-4.00004-5.
- A. Aytimur, S. Koçyiğit, İ. Uslu and F. Gökmeşe, Int. J. Polym. Mater. Polym. Biomater., 2015, 64, 111–116 CrossRef CAS.
- M. Abrigo, S. L. McArthur and P. Kingshott, Macromol. Biosci., 2014, 14, 772–792 CrossRef CAS PubMed.
- M. Godoy-Gallardo, U. Eckhard, L. M. Delgado, Y. J. D. de Roo Puente, M. Hoyos-Nogués, F. J. Gil and R. A. Perez, Bioact. Mater., 2021, 6, 4470–4490 CAS.
- Z. Lu, Y. Wu, Z. Cong, Y. Qian, X. Wu, N. Shao, Z. Qiao, H. Zhang, Y. She, K. Chen, H. Xiang, B. Sun, Q. Yu, Y. Yuan, H. Lin, M. Zhu and R. Liu, Bioact. Mater., 2021, 6, 4531–4541 CAS.
- Y. Zhang, T. Qiu, F. Jiang, S. Amzil, Y. Wang, H. Fu, C. Yang, Z. Fang, J. Huang and G. Dai, Appl. Surf. Sci., 2021, 556, 149818 CrossRef CAS.
- W. Zhang, H. Chen, H. Tian, Q. Niu, J. Xing, T. Wang, X. Chen and X. Wang, J. Mater. Chem. B, 2023, 11, 7641–7653 RSC.
- M. I. Khan, P. Paul, S. K. Behera, B. Jena, S. K. Tripathy, C. Stålsby Lundborg and A. Mishra, Chem. Eng. J., 2021, 417, 128025 CrossRef CAS.
- P. Panchal, D. R. Paul, A. Sharma, P. Choudhary, P. Meena and S. P. Nehra, J. Colloid Interface Sci., 2020, 563, 370–380 CrossRef CAS PubMed.
- Y. Wei, Y. B. Chong, H. Du, J. Kong and C. He, Mater. Des., 2018, 139, 153–161 CrossRef CAS.
- Y. Wang, J. Ma, Q. Xu and J. Zhang, Mater. Des., 2017, 113, 240–245 CrossRef CAS.
- M. Ramani, S. Ponnusamy, C. Muthamizhchelvan, J. Cullen, S. Krishnamurthy and E. Marsili, Colloids Surf., B, 2013, 105, 24–30 CrossRef CAS PubMed.
- M. Li, S. Pokhrel, X. Jin, L. Mädler, R. Damoiseaux and E. M. V. Hoek, Environ. Sci. Technol., 2011, 45, 755–761 CrossRef CAS PubMed.
- T. Wu, Y. Fu, S. Guo, Y. Shi, Y. Zhang, Z. Fan, B. Yang, B. Ding and Y. Liao, Aggregate, 2024, 5, e402 CrossRef CAS.
- M. Saravanan, V. Gopinath, M. K. Chaurasia, A. Syed, F. Ameen and N. Purushothaman, Microb. Pathog., 2018, 115, 57–63 CrossRef CAS PubMed.
- K. Kavithaa, M. Paulpandi, T. Ponraj, K. Murugan and S. Sumathi, Karbala Int. J. Modern Sci., 2016, 2, 46–55 CrossRef.
- K. Hoon Seo, J. Markus, V. Soshnikova, K. H. Oh, G. Anandapadmanaban, Z. Elizabeth Jimenez Perez, R. Mathiyalagan, Y. J. Kim and D. C. Yang, Inorg. Nano-Met. Chem., 2019, 49, 1–6 CrossRef CAS.
- S. M. H. Akhter, Z. Mahmood, S. Ahmad and F. Mohammad, Bionanoscience, 2018, 8, 811–817 CrossRef.
- A. Happy, M. Soumya, S. Venkat Kumar, S. Rajeshkumar, R. D. Sheba, T. Lakshmi and V. Deepak Nallaswamy, Biochem. Biophys. Rep., 2019, 17, 208–211 Search PubMed.
- G. Sharmila, C. Muthukumaran, K. Sandiya, S. Santhiya, R. S. Pradeep, N. M. Kumar, N. Suriyanarayanan and M. Thirumarimurugan, J. Nanostruct. Chem., 2018, 8, 293–299 CrossRef CAS.
- V. V. Shinde, D. S. Dalavi, S. S. Mali, C. K. Hong, J. H. Kim and P. S. Patil, Appl. Surf. Sci., 2014, 307, 495–502 CrossRef CAS.
- S. Jafarirad, M. Mehrabi, B. Divband and M. Kosari-Nasab, Mater. Sci. Eng. C, 2016, 59, 296–302 CrossRef CAS PubMed.
- A. D. T. Phan, G. Netzel, P. Chhim, M. E. Netzel and Y. Sultanbawa, Foods, 2019, 8, 258 CrossRef PubMed.
- S. Mickymaray, M. S. Al Aboody, P. K. Rath, P. Annamalai and T. Nooruddin, Asian Pac. J. Trop. Biomed., 2016, 6, 185–191 CrossRef CAS.
- B. Casciaro, A. Calcaterra, F. Cappiello, M. Mori, M. R. Loffredo, F. Ghirga, M. L. Mangoni, B. Botta and D. Quaglio, Toxins, 2019, 11, 511 CrossRef CAS PubMed.
- K. Ganesan and B. Xu, Int. J. Mol. Sci., 2017, 18, 2390 CrossRef PubMed.
- A. Chen, H. Tian, N. Yang, Z. Zhang, G.-Y. Yang, W. Cui and Y. Tang, Extracell. Vesicles Circ. Nucleic Acids, 2022, 3, 323–356 CAS.
- S. A. Shah, M. Sohail, M. U. Minhas, S. Khan, Z. Hussain, A. Mahmood, M. Kousar, H. E. Thu, M. Abbasi and M. U. R. Kashif, Int. J. Biol. Macromol., 2021, 185, 350–368 CrossRef CAS PubMed.
- Z. Zhang and X. Zhang, J. Biomed. Nanotechnol., 2021, 17, 1160–1169 CrossRef CAS PubMed.
- P. A. M. Chagas, R. Schneider, D. M. dos Santos, A. J. G. Otuka, C. R. Mendonça and D. S. Correa, React. Funct. Polym., 2021, 163, 104889 CrossRef CAS.
- I. M. Adel, M. F. ElMeligy, A. A. Abdelkhalek and N. A. Elkasabgy, Eur. J. Pharm. Sci., 2021, 164, 105888 CrossRef CAS PubMed.
- N. C. Adusumilli, B. Mordorski, J. Nosanchuk, J. M. Friedman and A. J. Friedman, Exp. Dermatol., 2021, 30, 705–709 CrossRef CAS PubMed.
- H. Tian, J. Yan, W. Zhang, H. Li, S. Jiang, H. Qian, X. Chen, X. Dai and X. Wang, Acta Biomater., 2023, 167, 449–462 CrossRef CAS PubMed.
- Q. Leng, Y. Li, X. Pang, B. Wang, Z. Wu, Y. Lu, K. Xiong, L. Zhao, P. Zhou and S. Fu, Drug Delivery, 2020, 27, 1676–1685 CrossRef CAS PubMed.
- H. C. Korting, C. Schöllmann and R. J. White, J. Eur. Acad. Dermatol. Venereol., 2011, 25, 130–137 CrossRef CAS PubMed.
- M. Farahani and A. Shafiee, Adv. Healthcare Mater., 2021, 2100477 CrossRef CAS PubMed.
- L. Jiang and S. C. J. Loo, ACS Appl. Bio Mater., 2021, 4, 3849–3862 CrossRef CAS PubMed.
- Y. Cui, W. Zhang, J. Shan, J. He, Q. Niu, C. Zhu, W. Wang, X.-L. Chen and X. Wang, Adv. Healthcare Mater., 2023, 13, 2302566 CrossRef PubMed.
- W. Qiu, H. Han, M. Li, N. Li, Q. Wang, X. Qin, X. Wang, J. Yu, Y. zhou, Y. Li, F. Li and D. Wu, J. Colloid Interface Sci., 2021, 596, 312–323 CrossRef CAS PubMed.
- R. N. Shamma, R. H. Sayed, H. Madry, N. S. El Sayed and M. Cucchiarini, Tissue Eng., Part B, 2022, 28, 451–463 CrossRef CAS PubMed.
- A. S. Kulkarni, S. R. Tapase, K. M. Kodam and V. S. Shinde, Colloids Surf., B, 2021, 205, 111834 CrossRef CAS PubMed.
- J. Youn, J. H. Choi, S. Lee, S. W. Lee, B. K. Moon, J. E. Song and G. Khang, Materials, 2021, 14, 1287 CrossRef CAS PubMed.
- D. Dantas Lopes dos Santos, J. F. Besegato, P. B. G. de Melo, J. A. Oshiro Junior, M. Chorilli, D. Deng, V. S. Bagnato and A. N. D. S. Rastelli, Photochem. Photobiol., 2021, 97, 1072–1088 CrossRef CAS PubMed.
- M. H. Sultan, W. A. Mahdi and Y. M. Kwon, Processes, 2020, 8, 1320 CrossRef CAS.
|
This journal is © The Royal Society of Chemistry 2024 |