DOI:
10.1039/D4TB00725E
(Paper)
J. Mater. Chem. B, 2024,
12, 7814-7825
Hydrogen-treated CoCrMo alloy: a novel approach to enhance biocompatibility and mitigate inflammation in orthopedic implants†
Received
4th April 2024
, Accepted 22nd May 2024
First published on 22nd May 2024
Abstract
In recent decades, orthopedic implants have been widely used as materials to replace human bone tissue functions. Among these, metal implants play a crucial role. Metals with better chemical stability, such as stainless steel, titanium alloys, and cobalt–chromium–molybdenum (CoCrMo) alloy, are commonly used for long-term applications. However, good chemical stability can result in poor tissue integration between the tissue and the implant, leading to potential inflammation risks. This study creates hydrogenated CoCrMo (H-CoCrMo) surfaces, which have shown promise as anti-inflammatory orthopedic implants. Using the electrochemical cathodic hydrogen-charging method, the surface of the CoCrMo alloy was hydrogenated, resulting in improved biocompatibility, reduced free radicals, and an anti-inflammatory response. Hydrogen diffusion to a depth of approximately 106 ± 27 nm on the surface facilitated these effects. This hydrogen-rich surface demonstrated a reduction of 85.2% in free radicals, enhanced hydrophilicity as evidenced by a decrease in a contact angle from 83.5 ± 1.9° to 52.4 ± 2.2°, and an increase of 11.4% in hydroxyapatite deposition surface coverage. The cell study results revealed a suppression of osteosarcoma cell activity to 50.8 ± 2.9%. Finally, the in vivo test suggested the promotion of new bone formation and a reduced inflammatory response. These findings suggest that electrochemical hydrogen charging can effectively modify CoCrMo surfaces, offering a potential solution for improving orthopedic implant outcomes through anti-inflammatory mechanisms.
1. Introduction
Cobalt–chromium–molybdenum (CoCrMo) alloys have been utilized as biomedical implants for several decades. Due to their excellent chemical stability, as well as high wear and corrosion resistance, they are commonly employed in heavy load-bearing implants such as hip joints, knee joints, dental implant materials, etc.1 The surface of these alloys forms a passivation layer primarily composed of Cr2O3, as well as oxides of Co and Mo, serving as the main corrosion-resistant layer.2 This layer protects the material from corrosive behaviors in challenging biological environments, such as pH variations and corrosion caused by bacteria and saliva in oral settings.3 During procedures like hip or knee joint replacement, the materials may face potential mechanically assisted corrosion in high-wear environments. Research indicates a success rate of over 90% for implants, with a lifespan of over 10 years.4 However, long-term use may lead to subtle inflammation due to autoimmune reactions or implant failure caused by aseptic loosening (no infection).5 Periprosthetic joint infection (PJI) is a serious complication occurring in 1% to 2% of primary arthroplasties.6 Therefore, reducing implant failures resulting from autoimmune reactions is crucial for improving the success rate in early implantation surgeries.
PJI is attributed to macrophage activation and response to implant byproducts (e.g. wear particles), leading to the activation of osteoclasts and osteolysis, ultimately resulting in implant failure caused by aseptic loosening.7 This type of macrophage activation is referred to as cell-induced corrosion. Previous studies have indicated that immune cells, such as osteoclasts, exhibit similar corrosion patterns in in vitro experiments with stainless steel and titanium alloys.8,9 Polymorphonuclear leucocytes on hydroxyapatite biomaterial surfaces also display cellular structures resembling the morphology of corrosion patterns.10 Cell-induced inflammatory reactions primarily involve the production of acid or reactive oxygen species (ROS) to attack foreign bodies, whether they are implants or invading bacteria.11 ROS particularly play a crucial role in inflammatory reaction.12 The metal ions released by corrosion within the implant, such as Co2+ and Cr3+ in CoCrMo, is triggered by the acidic environment or the use of iron ions from proteins (ferritin) by cells to accelerate the Fenton reaction, resulting in high concentrations of ROS.13,14 In an in vitro study conducted by Kurtz et al.,15 using open circuit potential (OCP) measurements to simulate ROS interactions with CoCrMo, it was found that the addition of 0.03 M H2O2 in phosphate-buffered saline (PBS) significantly increased CoCrMo's susceptibility to corrosion attack. The corrosion current densities increased by over 40 times compared to PBS alone, and more pronounced corrosion behavior was observed when HCl (pH = 3) was added.15
Among immune cells, osteal macrophages play a critical role in immune surveillance during the process of osseointegration of implant healing and bone homeostasis. Macrophages exhibit M1/M2 macrophage polarizations, with M1 polarization implicated in foreign body rejection. On the other hand, M2 polarization releases several cytokines, including bioactive growth factors such as bone morphogenetic protein-2, vitamin D3, transforming growth factor-β, etc.16 M2 macrophages are associated with higher peri-implant bone volume around stable implants in the early implant stages, contributing to improved osteogenesis.17 However, when ROS produced during inflammatory reactions act through the Fenton reaction, overproduction of ROS induces M1 polarization while inhibiting M2 polarization, leading to inflammation-induced implant failure during the early stages of osseointegration.18 Therefore, the emergence of surface coatings or treatment technologies holds promise in overcoming these challenges.19
This study introduces hydrogen treatment into CoCrMo material using the electrochemical cathodic charging method. By employing electrochemical hydrogen charging, hydrogen atoms can be incorporated into the material's surface, achieving the inhibition of ROS.20 In a previous study by Pixley et al.,21 the principle of degrading Mg to generate H2 was utilized. When Mg-doped mesh was implanted into mice, compared to the pure mesh, the Mg-containing mesh exhibited earlier M2 polarization of macrophages. Additionally, Zhang et al.22 implanted Mg wires into nude mice to inhibit the growth of ovarian cancer. These results serve as successful cases of materials releasing hydrogen at the affected site. This study will conduct comprehensive material investigations on hydrogen-charged CoCrMo (H-CoCrMo), examining its material properties, corrosion resistance, cellular inhibition of ROS, as well as in vivo and in vitro biological activity and inflammatory response.
2. Materials and methods
2.1. Materials
Unless specified otherwise, chemicals and solvents were procured from J. T. Baker (USA). Dulbecco's modified Eagle's medium (DMEM) and alpha-medium (α-MEM) were sourced from Gibco, Thermo Scientific, USA. The cell counting kit-8 (CCK-8) and alizarin red were obtained from Sigma (USA). The CoCrMo alloy (composition: Co 65.24%, Cr 27.68%, Mo 5.51%) was obtained from COSMO BIOTECH CO., LTD.
2.2. Characterization and equipment
The CoCrMo crystal structures were assessed using an X-ray diffractometer (XRD, X’Pert3 Powder; PANalytical, Netherlands). Material characterization was carried out using scanning electron microscopy (SEM, Phenom XL G2, Thermo Scientific, USA) supplemented with energy-dispersive X-ray spectroscopy (EDX) for surface morphology and compositional analysis. Focused ion beam-SEM (FIB-SEM, The Quanta™ 3D DualBeam™, FEI, USA) was used to observed hydrogen diffusion on the surface of H-CoCrMo. White light interferometry (WLI, Chroma 7502, Taiwan) and atomic force microscopy (AFM, Bruker Innova, Germany) were used to analyze the surface morphology and roughnesses. Inductively coupled plasma-optical emission spectroscopy (ICP-OES, Optima 8000, PerkinElmer, USA) was employed to examine the material's degradation and ion release processes; samples before and after hydrogen-charging treatment were immersed in simulated body fluid (SBF) at 37 °C in an incubator for 7, 14, and 28 days, respectively. The contact angle meter (CAM, Model 100SB, Sindatek, Taiwan) and a Nano Indentation Tester (TI-700; Hysitron, USA) were employed to assess material surface properties.
2.3. Electrochemical cathodic hydrogen-charging method
A CoCrMo rod was cut into smaller pieces, each with a diameter of 1 mm and a thickness of 2 mm, using a slow-cutting machine (CML 40, AIPSC, Taiwan). The pieces underwent sequential polishing with 600, 800, and 1200 Cw silicon carbide (SiC) paper. Final polishing was performed at a speed of 200 rpm using a 0.05 μm alumina polishing solution and cloth. Subsequently, the specimens were washed sequentially with acetone, ethanol, and deionized water using ultrasonication. After drying at room temperature to eliminate residual grease and dirt, the specimens were prepared for hydrogenation treatment.
A similar hydrogen charging method has been reported in previous research.12,20 In brief, hydrogen charging was carried out using a direct current (DC) power supply (DP-30032, HOLA, Taiwan) in an electrochemical cell. Platinum and the specimen served as the anode and cathode, respectively. Both electrodes were immersed in a 0.3 wt% ammonium thiocyanate (NH4SCN) and 3 wt% sodium chloride (NaCl) electrolyte. A DC power supply was used to apply various currents (0.0, 1.0, 1.5, and 1.8 A) for hydrogen charging, maintaining current densities of 0, 0.223, 0.335, and 0.402 A m−2 for 24 hours. The calculation formula for current density is shown in eqn (1). Hydrogen bubbles were generated to create a hydrogen-rich environment for hydrogenation. Following hydrogen charging, the specimens were cleaned three times with 95 wt% ethanol and prepared for subsequent experiments.
| 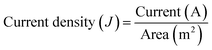 | (1) |
2.4. Electrochemical measurements
All electrochemical experiments were conducted using an electrochemical analyzer (CHI161114E, CH Instruments, USA) equipped with a conventional three-electrode cell. Sandwiching a CoCrMo alloy between Pt plates as the working electrode, with the Pt plate serving as the auxiliary electrode, and using Hg/Hg2Cl2 as the reference electrode, respectively. The active surface area of the working electrode was maintained at 0.25 cm2. Intensive polarization tests were carried out in a PBS solution across a potential range from −2.0 to +2.0 V at a scan rate of 5 mV s−1. The solutions were maintained at a pH of 7.4 and consisted of 8 g L−1 NaCl, 0.2 g L−1 potassium chloride (KCl), 1.434 g L−1 sodium phosphate dibasic dihydrate (Na2HPO4·2H2O), and 0.2 g L−1 potassium dihydrogen phosphate (KH2PO4). Electrochemical tests encompassed OCP and cyclic voltammetry (CV) methods to optimize hydrogen-charging conditions. The corrosion behavior of CoCrMo before and after hydrogenation was assessed using potentiodynamic polarization curves and Tafel plots. The polarization curve measurements were performed in a 3.5 wt% NaCl solution using a Gamry Reference 600 potentiostat. A standard three-electrode system was used for corrosion measurements. A metallic specimen was used as the working electrode (WE), a carbon rod as the counter electrode (CE), and a saturated calomel electrode (SCE) as the reference electrode (RE). The polarization curve measurements were carried out in the potential range from −200 mV to + 1000 mV versus OCP at a scan rate of 1 mV s−1. The corrosion potential (Ecorr), corrosion current density (Icorr), anodic Tafel slope (βa) and cathodic Tafel slope (βc) were obtained with Gamry Echem Analyst software. The tested area on all examined samples for electrochemical tests was 0.785 cm2.
2.5. Hydrogen storage measurement
The gas chromatography-thermal desorption system (GC-TDS) analysis was conducted using a system consisting of a tube furnace and a gas chromatograph (Agilent 7890
A, USA) equipped with a pulsed discharge ionization detector. The flow rate of 6 N helium carrier gas was set at 50 mL min−1. The specimens used for thermal desorption spectroscopy (TDS) were thick sheets measuring 1 cm2 × 2 mm. The specimens were prepared using the electrochemical cathodic hydrogen-charging method. Samples were cleaned and dried for analysis. The hydrogen desorption rate was measured by GC-TDS at a constant heating rate of 2.5 °C min−1, and the hydrogen content was calculated from 25 °C to 300 °C.
2.6.
In vitro bioactivity test
In vitro bioactivity test was used to evaluate the ability of hydroxyapatite (HAp) precipitation on the material surface after immersion into SBF. SBF was used to evaluate H-CoCrMo bioactivity. Crystallography analysis was performed using XRD technique to examine the crystal structure of HAp formation. The elemental compositions were qualitatively and quantitatively evaluated using SEM/EDX to compare their HAp surface coverage and calcium/phosphate ratios, which indicated HAp formation.
2.7. Cell biocompatibility test
All cell lines were procured from the American Type Culture Collection (ATCC, USA). L929 and MG63 cells were cultured in DMEM, while rat bone marrow mesenchymal stem cells (rBMSCs) were cultured in minimum essential α-MEM, maintaining a 37 °C and 5% CO2 environment. Cell biocompatibility assessments were performed on L929, MG63, and rBMSCs cell lines using a CCK-8 assay. CoCrMo and H-CoCrMo sheets underwent sterilization with ultraviolet (UV) light for 30 minutes and were subsequently placed in a 24-well plate. Each well was seeded with 5 × 104 cells in 500 μL of culture medium. The culture medium was refreshed every three days and observed. (EndoSCell, Endoscopic Scanner for Cellular Structure, Dendrite Precision Instruments, China). And then samples were transferred to new wells to eliminate unattached cells, ensuring result accuracy. After being washed thrice with PBS, a 200 μL mixture of CCK-8 reaction reagent and culture medium (ratio 1
:
9) was added to each well, which was then incubated in the dark for 2 hours. After completion of the reaction, the culture medium was transferred to a new 96-well plate, and a multimode microplate reader (MMR, VarioskanTM FLASH, Thermal, USA) was used to measure absorbance at 450 nm wavelength. Cell viability (%) was calculated using eqn (2): |  | (2) |
2.8. Alkaline phosphatase activity (ALP) test
To prepare the substrate buffer solution, 4-nitrophenol powder (0.1856 g) was dissolved in 100 mL of distilled water, and then a mixture of 0.4845 mL of 2-amino-2-methyl-1-propanol and 0.0407 g of magnesium chloride hexahydrate (MgCl2·6H2O) was added. Serial dilutions ranging from 20
000 to 0 μM were made by combining substrate buffer and distilled water in a 1
:
1 ratio, resulting in a total volume of 1000 μL. These diluted substrate buffers (200 μL each) were placed in 96-well plates to generate an alkaline phosphatase (ALP) standard curve, with absorbance measured at 450 nm.
For cell culture experiments, rBMSCs at a density of 1 × 104 cells were directly cultured on CoCrMo and H-CoCrMo sheets for 1, 3, 7, and 14 days. Subsequently, samples were collected and placed in 48-well plates. To extract cellular content, 500 μL of a solution containing 0.1% Triton X-100 in 0.1 M Tris buffer was evenly mixed via ultrasonication for 10 minutes. A 50 μL aliquot of the prepared sample solution was combined with 200 μL of substrate buffer and allowed to react for 60 minutes in a 96-well plate. The reaction was terminated by adding 100 μL of 1 N NaOH. Finally, 200 μL of the resulting solution was transferred to a well in a 96-well plate, and the absorbance was measured at 405 nm to determine the ALP concentration.
2.9. Free radical scavenging ability test
1,1-Diphenyl-2-picrylhydrazyl (DPPH) reagent (0.5 mM) was dissolved in ethanol. CoCrMo2 and H-CoCrMo sheets were immersed in 5 mL of DPPH solution in the dark for 6 days. The samples were collected, and the absorbance was measured at 517 nm every 24 h. The DPPH free radical-scavenging effect (%) was calculated using eqn (2): |  | (3) |
2.10. Reactive oxygen species (ROS) concentration
A 10 μM solution of 2′,7′-dichlorofluorescein diacetate (DCFH-DA) was prepared in dimethyl sulfoxide (DMSO). H-CoCrMo sheets were sterilized in a 48-well plate using UV light for 30 minutes. rBMSCs or MG63 cells (5 × 104) were then seeded on the sheets with 200 μL of culture medium and cultured in a 5% CO2 at 37 °C environment for 1 hour. Following incubation, the culture media were collected, mixed with 500 μL of DCFH-DA, and placed in an incubator for 30 minutes in the dark. The 48-well plate was washed twice with PBS and replaced with 1 mL of PBS. The concentration of ROS was measured using a fluorescence microscope (ECLIPSE 50i, Nikon, Japan) with excitation wavelengths of 485 and 530 nm.
2.11. Experimental setup and animal implantation
Femur implantation.
A femur implantation experiment was conducted using five-week-old Sprague Dawley (SD) rats. The experimental design included a control group (femur drilling only), a CoCrMo group, and an H-CoCrMo group, each replicated three times. Rod-like samples, measuring 1 mm in diameter and 20 mm in length, were prepared for implantation. The implantation experiments spanned two observation periods: early implantation at 2 weeks and subsequent reactions between tissues and implants at 4 weeks post-implantation. Experimental results were evaluated using computed tomography (CT) scanning and histological analysis. Bone density analysis from CT images was performed using ImageJ software.
Anesthesia was administered to the SD rats through intraperitoneal injection (Zoletil50
:
Rompun20 mixed in a 1
:
2 ratio), with a dosage of 0.1 mL per 100 g of rat body weight. Rats were immobilized on the operating table, and measures were taken to prevent biting and maintain eye moisture. The implant site was created using the trephine technique, and the alloy rod was inserted into the femur through the intramedullary canal. The wound was cleaned, sutured with absorbable sutures, and treated with Betadine and gold-iodine ointments to prevent infection. Rats were monitored in a warm observation area and transferred back to the feeding area upon regaining mobility. Postoperatively, the rats’ pathological conditions were regularly observed, and pain scores were recorded following experimental animal specifications. Rats were sacrificed at 2 and 4 weeks post-implantation for further testing and histological examinations. The procedures involving animals were approved by the ethics committee of Chang Gung Memorial Hospital (Taoyuan City, Taiwan). All experiments were approved by the Institutional Animal Care and Use Committee (IACUC) under Affidavit no. 2021092701.
2.12. Statistical analysis
The data in this study were collected in quintuplicate, and statistical analysis was performed using Origin one-way ANOVA to assess numerical differences between groups. The significance levels of differences were categorized as follows: * denotes a significant difference when p < 0.05 and ** indicates highly significant differences with p < 0.01.
3. Results
3.1. Electrochemical hydrogenation treatment characterization
CoCrMo alloy is renowned for its high chemical stability, making it challenging for hydrogen ions to diffuse through its surface. Electrochemical hydrogenation treatment emerges as a promising technique for such surface treatments. The choice of electrolyte and charging current plays a crucial role in achieving optimized hydrogen charging. The CV testing method was employed to optimize the charging conditions, using three types of electrolytes: NaOH + NaCl + NH4SCN and H2SO4 + NH4SCN (shown in Fig. S1, ESI†), and NaCl + NH4SCN (shown in Fig. 1(a)). Among these electrolytes, NaCl + NH4SCN proved to be more efficient for hydrogenation compared to the other two. The redox curves indicated an increase in hydrogenation with an increasing current, reaching up to 18A (current density of 0.402 A cm−2). The concentration of hydrogen charged onto the surface was measured using GC-TDS. Samples were heated to 300 °C at a rate of 2.5 °C min−1 to accelerate hydrogen release. The H-CoCrMo sample exhibited an initial hydrogen release at 30 °C, reaching maximum release at 144 °C. In contrast, the CoCrMo sample showed no release until 110 °C. It is worth noting that the hydrogen release at such high temperatures may be attributed to a certain amount of hydrogen mixture introduced during CoCrMo metallic processing. This hydrogen mixture can mitigate oxidation during forging and annealing processes.23 Consequently, CoCrMo released hydrogen at a higher temperature compared to H-CoCrMO, which released hydrogen at room temperature. In conclusion, the total accumulated hydrogen concentration is 0.157 ppmw for H-CoCrMo and 0.410 ppmw for CoCrMo.
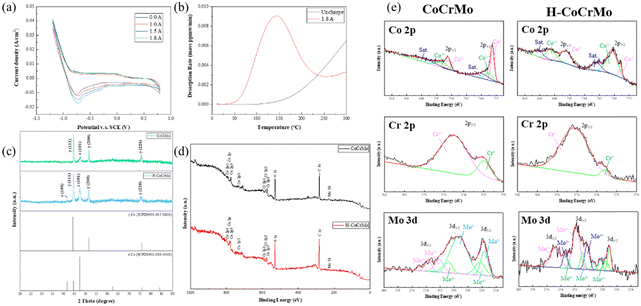 |
| Fig. 1 (a) Cyclic voltammetry (CV) curves of CoCrMo alloy charging with NaCl + NH4SCN electrolyte in the presence of PBS; (b) thermal desorption spectral (TDS) measurement of H-CoCrMo sheets at a heating rate of 2.5 °C min−1; (c) XRD patterns of CoCrMo and H-CoCrMo alloys; (d) XPS full spectrum of CoCrMo and H-CoCrMo alloys; and (e) Co 2p, Cr 2p, and Mo 3d spectra of CoCrMo and H-CoCrMo alloys. | |
The XRD patterns of CoCrMo and H-CoCrMo, as illustrated in Fig. 1(c), indicate a decrease in the ε phase after the hydrogenation treatment. X-ray photoelectron spectroscopy (XPS) was employed to analyze the surface elements of CoCrMo and H-CoCrMo alloys. Fig. 1(d) displays the full spectrum of CoCrMo and H-CoCrMo, while (e) represents the Co 2p, Cr 2p, and Mo 3d spectra of both alloys. The XPS results reveal peak spectra corresponding to Co, Cr, and Mo metals and their oxides before and after hydrogen charging. Peaks associated with these elements are observable under both conditions. Notably, after hydrogen charging, signals from various metal types are significantly reduced, indicating the formation of a protective oxide layer upon re-exposure to air. As depicted in Fig. 1(e), the Co 2p spectrum suggests that Co primarily remains in a metallic state. In the Cr 2p and Mo 3d spectra, noticeable oxide formation is evident.24 Higher binding energies in elements coordinating with metal ions lead to XPS signal shifts, as seen in Fig. 1(c) of the XRD data, illustrating a direct relationship between the increased ε characteristic peaks of H-CoCrMo, composed of Cr- and Mo-rich phases. The cathodic hydrogen charging process reduces the surface of the CoCrMo alloy, converting the passivation layer back to a metallic state. Upon exposure to air, Cr and Mo metals rapidly oxidize, forming films such as Cr2O3 and MoO3.3,25 Subsequent results indicate that these oxides enhance corrosion resistance.
3.2. Surface and element observation
Observing the surface morphology provides a direct method for discerning the effects of hydrogenation on the CoCrMo surface. SEM and EDX mapping images in Fig. 2 depict changes in surface morphology and the analysis of element distribution after hydrogenation. In Fig. 2(a), the CoCrMo surface appears smooth with minor traces of mechanical polishing. Fig. 2(b) reveals round-black precipitates on the surface of H-CoCrMo. Fig. 2(c) EDX spectra suggest a homogeneous element distribution without significant precipitation. To characterize these differences, EDX was utilized to screen the element distribution. Fig. 2(d) indicates a distinct distribution of Co, Cr, Mo, and O. The black precipitates exhibit a high Cr and Mo oxidants signal, aligning with XRD and XPS results.
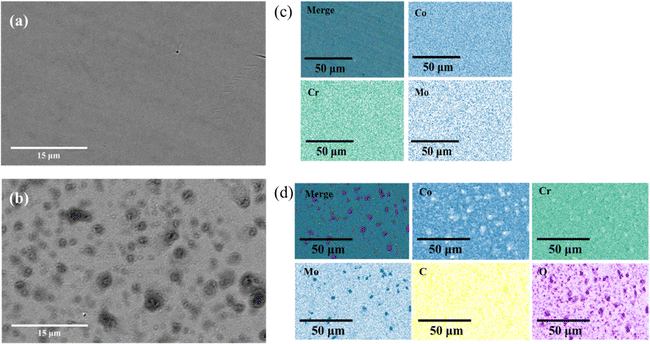 |
| Fig. 2 SEM surface morphology images of (a) CoCrMo and (b) H-CoCrMo alloys; (c) and (d) are EDX element mappings of CoCrMo and H-CoCrMo alloys according to elements. | |
3.3. Material property characterization
Electrochemical cathodic hydrogen charging involves the use of a hydrogen-rich electrolyte to control the direction of electric current in an electric field. This drives hydrogen ions to the material surface for reduction. During this reduction process, hydrogen ions diffuse onto the material surface, thereby altering the surface properties of the material. As depicted in Fig. 3(a) and (b), the CoCrMo material exhibits a relatively smooth surface after mechanical polishing, with surface roughness measured as 1.39 ± 0.39 μm using WLI and 0.81 ± 0.15 μm using AFM. On the other hand, as shown in Fig. 3(c) and (d) for H-CoCrMo after hydrogen charging, there is a noticeable presence of granular precipitates on the material surface. This phenomenon aligns with the presence of precipitates indicated in the Fig. 2(c) SEM image. The surface roughness measured through WLI and AFM is 3.57 ± 0.33 μm and 12.73 ± 1.65 μm, respectively. Meanwhile, the hydrogen diffusion depth is monitored using SEM, and the image formed using the backscattered electron image (BEI) mode is shown in Fig. 3(e). Samples were created with a cross-section of 2 μm width and 1 μm depth using focused ion beam SEM (FIB-SEM). The red arrow highlights the diffusion of the hydrogen layer, represented as a darker layer. The hydrogen diffusion depth is about 106 ± 27 nm. The hydrogen-diffused layer can change surface properties to create a hydrophilic surface.26 Surface hydrophilicity is determined using contact angle measurement (CAM) as depicted in Fig. 3(f). The water contact angle of CoCrMo is 83.57 ± 1.92°, and for H-CoCrMo, it is 52.40 ± 2.29°. These results suggest that hydrogenation treatment can improve the hydrophilicity of the material. This increase may be attributed to two main factors: higher surface roughness and the hydrogenated surface. The hydrogen-rich surface can provide hydrophilic cations on the surface, which can react with water molecules and OH− to improve hydrophilicity.26 The mechanical property tests on the material surface are shown in Fig. 3(g) and (h). It can be observed that both Young's modulus and surface microhardness of the material exhibit a significant decrease after the hydrogenation treatment. The potentiodynamic polarization patterns reveal the corrosion potential (Ecorr) of CoCrMo and H-CoCrMo, which changes from −248 mV to 171 mV, as listed in Table 1. The representative pothentiodynamic polarization patterns are shown in Fig. 3(i). The hydrogen treatment brings about significant changes in the surface properties of the material. The appearance of surface precipitates notably enhances surface roughness, leading to improved hydrophilicity. Moreover, the decrease in surface hardness renders it more conducive to bone tissue integration. Finally, the material surface shows higher activity and an increased potential for oxidation.
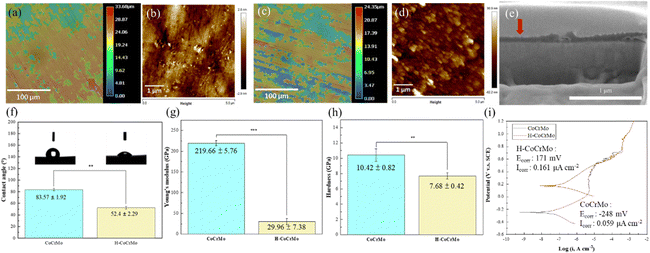 |
| Fig. 3 The surface property characterization of (a) and (b) CoCrMo and (c) and (d) H-CoCrMo using white light interferometry (WLI) and atomic force microscopy (AFM). (e) FIB-SEM BEI mode cross-section image depicting the hydrogen diffusion depth from the surface of the H-CoCrMo sheet (the red arrow indicates the hydrogen diffusion region). (f) displays water contact angle measurements, while (g) and (h) show surface mechanical properties measurements using a nano-indenter before and after hydrogenation treatment of CoCrMo. (i) depicts potentiodynamic polarization patterns of CoCrMo and H-CoCrMo sheets in 3.5 wt% NaCl (scan rate = 1 mV s−1) to assess the corrosion resistance of the samples. Here, Ecorr indicates the corrosion potential, and Icorr represents the corrosion current. | |
Table 1 Electrochemical corrosion parameters of different specimens obtained from polarization curves
Specimen |
E
corr mVSCE |
I
corr μA cm−2 |
β
a mV decade−1 |
β
c mV decade−1 |
CoCrMo |
−248 |
0.059 |
140.7 |
−153.0 |
H-CoCrMo |
171 |
0.161 |
188.4 |
−78.8 |
3.4.
In vitro bioactivity test
The bioactivity test used in this study involves immersing materials in simulated body fluid (SBF) to observe the formation of apatite. The procedure follows the method outlined by Kokubo et al.27 Bioactive materials are substances that can form bonds similar to bone components, specifically HAp, when in contact with living bone. Although some studies suggest that this method may not be the most rigorous for bioactivity testing,28 it still serves as an indicator for in vitro bioactivity. In this experiment, we not only investigate the formation of apatite but also employ an ALP assay to further confirm the extracellular bioactivity at the cellular level.
In Fig. 4(a), the surface morphologies of CoCrMo and H-CoCrMo after immersion in SBF for 7, 14, and 21 days are illustrated. It can be observed that both material surfaces exhibit spherical precipitates after 14 days of experimentation, indicating the initial formation of apatite in a metastable state. After 21 days, the CoCrMo material continues to maintain spherical precipitates, whilr H-CoCrMo begins to exhibit continuous precipitation, suggesting a higher efficiency in reaching a thermodynamically stable state. Therefore, in Fig. 4(b), the calculation of apatite coverage reveals that H-CoCrMo suggest slightly higher coverage than the CoCrMo sample group. Additionally, the results of the EDX elemental analysis indicate a Ca/P ratio of 1.59 for H-CoCrMo (as shown in Fig. S2, ESI†), which closely aligns with the Ca/P ratio of hydroxyapatite (HA), typically around 1.6.29,30 In the FTIR spectrum presented in Fig. 4(c), H-CoCrMo, after 14 days of experimentation, exhibits significant bands corresponding to HA, as evidenced by bands characteristic of HA in the FTIR spectrum. The PO43− vibration modes can be observed at around 560 to 600 and 953 to 1100 cm−1, and HPO42− is identified at 874 cm−1. Accompanied by the C–H vibration at 1384 cm−1, the O–H vibrations at 1633 and 3432 cm−1, and the CO32− at 1457 cm−1.31
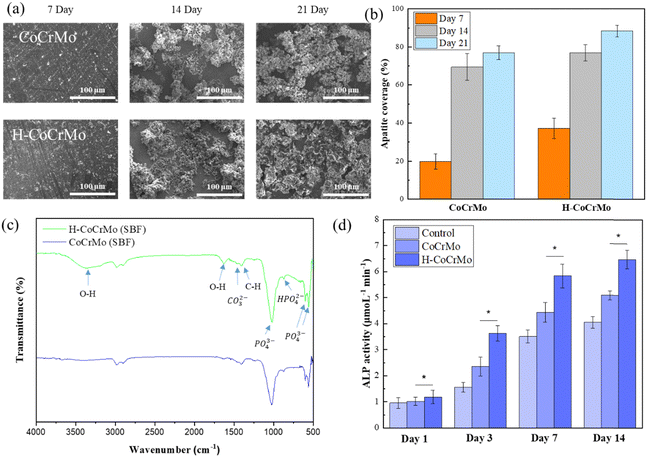 |
| Fig. 4 (a) SEM observations of in vitro bioactivity tests for CoCrMo and H-CoCrMo sheets immersed in SBF for 7, 14, and 21 days; (b) apatite coverage on the surface of the materials. Additionally, (c) FTIR spectrum of CoCrMo and H-CoCrMo after 14 days in vitro bioactivity tests were performed by soaking into SBF to evaluate the potential formation of apatite. Finally, (d) an ALP activity test was conducted using rBMSCs co-cultured on CoCrMo and H-CoCrMo samples for 1, 3, 7, and 14 days. | |
The use of SBF for assessing full bioactivity has been questioned due to its inability to simulate cellular responses to materials, despite its aim to mimic the plasma environment.27,28 While apatite deposition can be promoted by altering the surface morphology, pH values, or the quantity of surface total OH groups,29 the bonding of materials with live bone requires interaction with cells. Therefore, the ALP assay is employed to measure the differentiation behavior of rBMSCs. As illustrated in Fig. 4(d), the ALP activity of H-CoCrMo increases over time, indicating a trend of rBMSCs differentiating into osteoblasts. This HAp formation and increased ALP activity demonstrate that hydrogenation treatment contributes to enhancing in vitro bioactivity.
3.5. Cellular responses and the effect of ROS
CoCrMo is commonly used for bioimplants due to its excellent mechanical properties and corrosion resistance. However, previous studies have indicated that ion release from the metal surface may lead to cellular toxicity.32 In this study, ICP-OES was employed to detect ion release, as shown in Table S1 (ESI†). Interestingly, no ion release was detected in a biologically simulated environment. Nonetheless, untreated CoCrMo does not provide an optimal surface for cell attachment and differentiation initially.33Fig. 5(a) and (b) illustrates the results of cell viability experiments with L929 and rBMSCs, indicating that the biological activity of CoCrMo and H-CoCrMo was 63.9 ± 4.3% and 74.9 ± 4.9%, respectively, on the first day. As the co-cultivation time increased, biological activity also rose, reaching 92.3 ± 6.5% for CoCrMo and 95.6 ± 5.0% for H-CoCrMo on the seventh day. These results suggest that the hydrophilic surface of H-CoCrMo is more favorable for cell attachment and differentiation compared to CoCrMo.
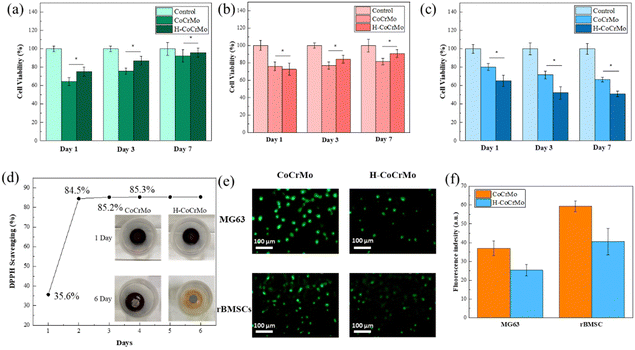 |
| Fig. 5 (a)–(c) Cell viabilities of L929, rBMSCs, and MG63 cells on CoCrMo and H-CoCrMo for 1, 3, and 7 days. (d) ROS scavenging abilities using the DPPH assay over 6 days in vitro. The DPPH assay in the figure exhibits a deep purple color, and through the hydrogen reduction process, it eliminates ROS, leading to an overall tendency to turn a light yellow color. (e) DCFH-DA assay presents fluorescent images of ROS in MG63 and rBMSCs cultured on CoCrMo and H-CoCrMo, and (f) quantitative analysis of ROS levels in MG63 and rBMSCs to observe cellular responses using the DCFH-DA assay. | |
In Fig. 5(c), the cell viability on the MG63 human osteosarcoma cell line is compared with that of normal cells. On the first day, the cell viability of CoCrMo was 80.1 ± 3.6%, while that of H-CoCrMo was 65.1 ± 5.9%. By the seventh day, cell viability changed to 66.4 ± 2.5% for CoCrMo and 50.8 ± 2.9% for H-CoCrMo. The viability of MG63 cells on H-CoCrMo decreased over time. In Fig. 5(d), the DPPH assay demonstrates an ROS scavenging capability of 84.5% after two days of reaction, with the reagent exhibiting a bright yellow color. Fig. 5(e) and (f) show the DCFH-DA assay to examine cellular oxidative stress and antioxidant effects. It is evident that H-CoCrMo has a suppressive effect on ROS for both rBMSCs and MG63 cell types. However, it exhibits a more pronounced inhibitory effect on MG63. The hydrogenation treatment enhances the biocompatibility of CoCrMo, simultaneously showcasing excellent ROS scavenging capabilities and inhibiting the proliferation of human osteosarcoma. This could serve as a potential application for anti-inflammatory and anti-cancer purposes.
3.6.
In vivo femur implantation
X-ray images are currently one of the most widely used methods in clinical settings to assess bone density. In Fig. 6(a)–(f), X-ray images illustrate the bone density at the interfaces of the control, CoCrMo and H-CoCrMo with the surrounding bone at both two and four weeks post-implantation. The X-ray images for bone density observation were analyzed using ImageJ software to compare the bone density at the interface with the surrounding materials. After two weeks of implantation, the bone density for the control group was 62.6 ± 2.4%, and it reached 68.7 ± 2.2% at four weeks post-implantation. For CoCrMo, the bone density increased from 72.7 ± 4.2% at two weeks to 76.6 ± 6.9% by the fourth week. In contrast, H-CoCrMo exhibited a bone density of 77.6 ± 5.4% at two weeks post-surgery, which further increased to 85.8 ± 3.64% by the fourth week. Therefore, H-CoCrMo exhibited a significant increase in bone density at the interface with the material.
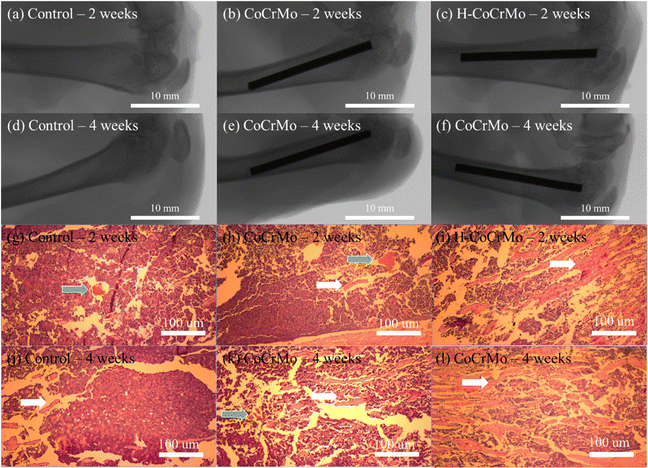 |
| Fig. 6 (a)–(f) X-ray images showing side views to observe CoCrMo and H-CoCrMo implants at 2 and 4 weeks post-implantation, revealing bone density near the implant. (g)–(l) Histological analysis of femoral implantation to observe biological responses using H&E staining (green arrows indicate inflammatory responses, and white arrows indicate new bone formation). | |
Fig. 6(g)–(l) illustrate the histological H&E staining results of the femur implantation surgery. Changes in the tissue surrounding the implant were observed at 2 and 4 weeks post-implantation. In the control and CoCrMo groups, an inflammatory response and a small amount of new bone tissue were evident in the surrounding tissue after two weeks post-implantation. In contrast, the H-CoCrMo group showed no observable inflammatory response, with a significant amount of bone tissue present in the images. After four weeks of surgery, the CoCrMo group still exhibited a minor inflammatory response, while the H-CoCrMo group primarily showcased dominance of new bone tissue regeneration. Combining the results from in vitro and in vivo experiments confirms that H-CoCrMo can significantly reduce the generation of inflammatory responses and contribute to mineral deposition, forming HAp on the material surface. This promotes new bone formation, suggesting that the electrochemical hydrogen charging treatment holds potential as a treatment for optimizing orthopedic implant surfaces.
4. Discussion
The crystal structure of CoCrMo plays a crucial role in determining the mechanical properties of the material. For example, the γ phase (face-centered cubic, FCC structure) is effective in enhancing the alloys’ ductility.34 The presence of the ε phase (hexagonal close-packed, HCP structure), forming two different lattices, creates obstacles for dislocation movement, thereby inducing strengthening. This strengthening has the potential to reduce the wear rate, making it a promising material for implants.35 The XRD patterns of CoCrMo and H-CoCrMo exhibit a significant increase in the ε phase compared to CoCrMo. This phase transition is attributed to the stability of the ε phase during room temperature processing.36 In the SEM images, round-black precipitates can clearly be observed on the surface of H-CoCrM, where the bright surface and black precipitates are dominated by the γ phase and ε phase.37 It is worth mentioning that in our previous study, similar treatments were applied to 316L stainless steel. Post cathodic hydrogen charging, a body-centered cubic (BCC) α-ferrite phase was formed with the (110) plane, and significant phase precipitation was observed on the surface in SEM images, thereby altering the surface morphology and properties. However, this did not affect the overall material properties.20 The ε phase is primarily composed of Cr and Mo.38 Generally, under the influence of external energy, the transformation of the metastable γ phase into the ε phase is referred to as martensitic transformation. Through prolonged heat treatment, a stable FCC to HCP transition can be achieved.39 Consequently, the microstructure tends to form a Cr and Mo-rich honeycomb structure, thereby enhancing its mechanical properties.38 However, H-CoCrMo displays spherical precipitates, leading to a deterioration in the material's surface mechanical properties. These precipitates may result from two mechanisms, namely, the Bernard Marangoni driven instability (BMI) and the particle accumulated structure (PAS) formation mechanisms.40 Both mechanisms can indicate external forces during the alloy phase transformation process, such as surface tension, temperature variations, compositional gradients, and the generation of spherical grains during solidification-melting processes. The γ phase of CoCrMo is primarily composed of CoCr; consequently, Mo is ejected to the grain boundary, generating a metastable structure of Cr and Mo-rich ε phase. This results in a significant decrease in surface mechanical properties.41 Although the crystal structure of H-CoCrMo tends to exhibit more ε phase, the reduction of the stable γ phase is replaced by spherical grains, indicating that the influence of phase transformation on mechanical properties is weaker than that of precipitates. Finally, the granular grains formed on the material surface are composed of a Cr and Mo-rich ε phase. Upon contact with oxygen in the air, a passivation film rapidly forms, significantly enhancing the overall corrosion resistance.
The application of CoCrMo in orthopedic implants requires experience with complex biological environments. Therefore, corrosion resistance is not the only important property; surface characteristics also play a significant role in biological activity. Compared to CoCrMo, H-CoCrMo treated with hydrogenation not only generates Cr and Mo-rich ε phases but also increases surface roughness and enhances hydrophilicity. This behavior provides a physical environment conducive to cell attachment.33 After implantation as biomaterials, implants will undergo long-term biocorrosion in the body, where there is a high concentration of Cl− ions and low concentrations of other inorganic compounds such as Ca2+, PO43−, and HCO3−. Among these, Cl− ions may accelerate corrosion. Therefore, the formation of a passive protective layer on the surface is essential.42 For H-CoCrMo, Mo-rich precipitation may confer better resistance to biocorrosion compared to CoCr-rich phases, which can lead to non-uniform biocorrosion surface features. Thus, it provides a rougher surface for cell attachment, thereby promoting integration between tissue and material.43 Additionally, hydrogenation treatment also aids in cell attachment functionality. Cell adhesion to their substrate plays a vital role in cell development. Paola et al.'s study indicates that ROS produced after integrin receptor engagement during cell adhesion and spreading affect low molecular weight protein tyrosine phosphatases (LMW-PTP), which are involved in the regulation of cell proliferation, cytoskeletal organization, and focal adhesion formation.44 However, ROS can oxidize LMW-PTP.45 Therefore, H-CoCrMo's ability to eliminate ROS can lead to better performance in cell viability experiments with L929 and rBMSCs compared to CoCrMo.
Another important function of H-CoCrMo is to serve as a carrier for hydrogen, releasing hydrogen to the implant site to provide a high concentration of hydrogen. Through its reductive properties, it reduces the post-surgery secretion of ROS by cells. ROS, such as hydrogen peroxide, not only emerge as by-products of immune responses but also play a crucial role in cancer cells, acting as mediators for cellular signaling.46 ROS can upregulate the generation of pro-inflammatory cytokines like tumor necrosis factor-alpha (TNF-α), interleukin-1 beta (IL-1β), and interleukin 6 (IL-6), inhibiting the osteogenic differentiation of BMSCs and promoting osteoclast proliferation, leading to bone defect lesions.47,48 In this study, H-CoCrMo functions as a hydrogen delivery implant, creating a hydrogen-rich environment through hydrogen diffusion release. Hydrogen is efficient in scavenging free radicals as an antioxidant, thereby reducing the generation of ROS and oxidative stress.49 Molecules and ions involved in free radical reactions act as primary or secondary messengers.50 Consequently, this reduction damages the mitochondrial metabolism of cancer cells and induces apoptosis.51 Another mechanism contributing to the antioxidant effect of hydrogen is its impact on signal-regulating kinase-1 (ASK1) and the regulatory pathway of mitogen-activated protein kinase (p38-MAPK), mediating the antioxidant activity of the compound and its anti-apoptotic properties to facilitate bone regeneration.52 Simultaneously, molecular hydrogen influences ASK1- and p38 MAPK-dependent regulatory pathways, which are also implicated in inflammation.53 Although these cellular pathways are complex, the action of hydrogen involves reducing ROS, thereby altering the signaling pathways of molecules and ions between cells. During inflammation, hydrogen exhibits several potential anti-inflammatory mechanisms. It reduces neutrophil and macrophage infiltration by inhibiting the expression of adhesion molecules and chemokines. It also decreases the levels of proinflammatory cytokines, colony-stimulating factors, and high mobility group box 1 (HMGB-1) protein. Additionally, hydrogen suppresses the main inflammatory cascade controlled by nuclear factor-kappa B (NF-κB) protein, which is activated by various inflammatory stimuli.54,55 This suppression occurs through the inhibition of NF-κB activity, blocking its translocation to the nucleus, and an increase in the level of inhibitor of kappa B (IκB), thereby preventing NF-κB from binding to DNA.54 Another potential mechanism involves the activation of interleukin-10 (IL-10), which suppresses inflammation locally or systemically. Moreover, hydrogen modulates the balance between T-helper cell subtypes, T-regulatory lymphocytes, and mast cells, thereby contributing to its anti-inflammatory and immunomodulatory effects.55In vivo experiments conducted in this study demonstrated significant anti-inflammatory effects.
5. Conclusions
The electrochemical hydrogen charging method can direct hydrogen to the surface of CoCrMo for modification, allowing for minimal and uniform treatment of the surface without compromising its overall properties. Under the influence of an electric field and hydrogen reduction, the surface of H-CoCrMo undergoes the formation of spherical ε phase precipitation. This crystal structure, along with the formation of Cr and Mo-rich oxides, enhances the material's corrosion resistance. This hydrogen treatment makes the surface more hydrophilic compared to CoCrMo, making it more suitable for apatite precipitation and cell attachment and proliferation. In both in vitro and in vivo tests, the mechanism of ROS scavenging is employed to suppress inflammatory reactions, thereby promoting enhanced bone integration between tissue and material, making this treatment potentially useful for orthopedic implant modification.
Ethics approval and consent to participate
All experiments were conducted with the approval of the Institutional Animal Care and Use Committee of Chang Gung Memorial Hospital (2021092701).
Data availability
The datasets used and/or analyzed during the current study are available from the corresponding authors on reasonable request.
Author contributions
Yu-Chien Lin: writing – original draft, writing – review and editing, methodology, and formal analysis. Chih-Chien Hu: writing – review and editing, methodology, data curation, and visualization. Wai-Ching Liu: writing – original draft, writing – review and editing, methodology, and formal analysis. Udesh Dhawan: writing – original draft, writing – review and editing, methodology, and formal analysis. Yu-Chieh Chen: investigation, formal analysis, data curation, and validation. Yueh-Lien Lee: resources, methodology, and project administration. Hung-Wei Yen: conceptualization, methodology, supervision, writing – review and editing, and funding acquisition. Yi-Jie Kuo: conceptualization, methodology, supervision, writing – review and editing, and funding acquisition. Ren-Jei Chung: conceptualization, methodology, supervision, project administration, funding acquisition, and writing – review and editing.
Conflicts of interest
The authors declare that they have no known competing financial interests or personal relationships that could have appeared to influence the work reported in this paper.
Acknowledgements
Technical assistance from the Precision Analysis and Material Research Center of National Taipei University of Technology (Taipei Tech) is appreciated. Assistance with X-ray images from the Taiwan Mouse Clinic (TMC) is appreciated. The authors are grateful for the financial support received from the National Science and Technology Council of Taiwan (MOST 108-2628-E-027-003-MY3; and MOST 109-2224-E-002-002); the National Taipei University of Technology and Chang Gung Memorial Hospital Joint Research Program (NTUT-CGMH-111-01 [CORPG3M0191]); and the Chang Gung Memorial Hospital Research Program (CMRPG3M1861).
References
- D. de Castro Girão, M. Béreš, A. L. Jardini, R. Maciel Filho, C. C. Silva, A. de Siervo, H. F. G. de Abreu and W. S. Araújo, Mater. Sci. Eng., C, 2020, 107, 110305 Search PubMed.
- O. Öztürk, U. u Türkan and A. E. Eroglu, Surf. Coat. Technol., 2006, 200, 5687–5697 CrossRef.
- S. De Aguiar, M. Nicolai, M. Almeida and A. Gomes, Bio-Med. Mater. Eng., 2015, 25, 53–66 Search PubMed.
- S. B. Goodman, Y. T. Konttinen and M. Takagi, J. Long-Term Eff. Med. Implants, 2014, 24, 253–257 CrossRef.
- R. Supra and D. K. Agrawal, Orthop. J. Sports Med., 2023, 5, 9 Search PubMed.
- P. Izakovicova, O. Borens and A. Trampuz, EFORT Open Rev., 2019, 4, 482 Search PubMed.
- M. Couto, D. P. Vasconcelos, D. M. Sousa, B. Sousa, F. Conceição, E. Neto, M. Lamghari and C. J. Alves, Front. Mater., 2020, 7, 274 Search PubMed.
- D. Cadosch, E. Chan, O. P. Gautschi, H. P. Simmen and L. Filgueira, J. Orthop. Res., 2009, 27, 841–846 Search PubMed.
- D. Cadosch, M. S. Al-Mushaiqri, O. P. Gautschi, J. Meagher, H. P. Simmen and L. Filgueira, J. Biomed. Mater. Res., Part A, 2010, 95, 1004–1010 Search PubMed.
- F. Velard, J. Braux, J. Amedee and P. Laquerriere, Acta Biomater., 2013, 9, 4956–4963 Search PubMed.
- G. M. Rosen, S. Pou, C. L. Ramos, M. S. Cohen and B. E. Britigan, FASEB J., 1995, 9, 200–209 CrossRef CAS.
- Y.-C. Lin, C.-C. Hu, T.-T. Nguyen, U. Dhawan, C.-Y. Chou, Y.-L. Lee, H.-W. Yen, Y.-J. Kuo and R.-J. Chung, J. Mater. Res. Technol., 2023, 1504–1513 Search PubMed.
- J. Prousek, Pure Appl. Chem., 2007, 79, 2325–2338 CrossRef CAS.
- P. Biemond, A. J. Swaak, H. G. Van Eijk and J. F. Koster, Arthritis Rheum., 1986, 29, 1187–1193 Search PubMed.
- J. L. Gilbert, S. Sivan, Y. Liu, S. B. Kocagöz, C. M. Arnholt and S. M. Kurtz, J. Biomed. Mater. Res., Part A, 2015, 103, 211–223 CrossRef.
- R. M. Yang, H. S. Talib, R. J. Miron and T. G. Wiedemann, Compendium, 2022, 43(10), 15488578 Search PubMed.
- S. Landgraeber, M. Jäger, J. J. Jacobs and N. J. Hallab, Mediators Inflammation, 2014, 2014, 185150 Search PubMed.
- B. Zhang, Y. Yang, J. Yi, Z. Zhao and R. Ye, J. Periodontal Res., 2021, 56, 991–1005 CrossRef CAS.
- K. Rahmouni, A. Besnard, K. Oulmi, C. Nouveau, A. Hidoussi, L. Aissani and M. Zaabat, Surf. Coat. Technol., 2022, 436, 128310 CrossRef CAS.
- Y.-C. Lin, U. Dhawan, Y.-C. Liu, Y.-L. Lee, X. Liu, H.-W. Yen, C.-C. Hu and R.-J. Chung, Surf. Coat. Technol., 2024, 130499 CrossRef CAS.
- U. Adhikari, X. An, N. Rijal, T. Hopkins, S. Khanal, T. Chavez, R. Tatu, J. Sankar, K. J. Little and D. B. Hom, Acta Biomater., 2019, 98, 215–234 CrossRef CAS.
- S. Qiao, Y. Wang, R. Zan, H. Wu, Y. Sun, H. Peng, R. Zhang, Y. Song, J. Ni and S. Zhang, ACS Biomater. Sci. Eng., 2020, 6, 1755–1763 CrossRef CAS.
- K. Gupta, J. Phase Equilib. Diffus., 2005, 26, 87 CrossRef CAS.
- S. N. Ogugua, O. M. Ntwaeaborwa and H. C. Swart, Bol. Soc. Esp. Ceram. Vidrio, 2021, 60, 147–162 Search PubMed.
- I. J. Badovinac, I. K. Piltaver, R. Peter, I. Saric and M. Petravic, Appl. Surf. Sci., 2019, 471, 475–481 CrossRef.
- S. Tanaka, S. Takaya, T. Kumeda, N. Hoshi, K. Miyatake and M. Nakamura, Int. J. Hydrogen Energy, 2021, 46, 28078–28086 CrossRef CAS.
- T. Kokubo and H. Takadama, Biomaterials, 2006, 27, 2907–2915 CrossRef CAS.
- M. Bohner and J. Lemaitre, Biomaterials, 2009, 30, 2175–2179 CrossRef CAS.
- S. Ferraris, S. Yamaguchi, N. Barbani, M. Cazzola, C. Cristallini, M. Miola, E. Vernè and S. Spriano, Acta Biomater., 2020, 102, 468–480 CrossRef CAS.
- H. Liu, H. Yazici, C. Ergun, T. J. Webster and H. Bermek, Acta Biomater., 2008, 4, 1472–1479 Search PubMed.
- S. Thirumurugan, Y.-C. Lin, G.-Y. Lin, K.-S. Fan, Y.-H. Liang, Y.-J. Kuo and R.-J. Chung, J. Aust. Ceram. Soc., 2022, 58, 1357–1366 Search PubMed.
- I. Suci, S. Sukaryo and E. Rohaeti, IOP Conf. Ser. Earth Environ. Sci., 2018, 184, 012010 CrossRef.
- B. Lohberger, N. Stuendl, D. Glaenzer, B. Rinner, N. Donohue, H. C. Lichtenegger, L. Ploszczanski and A. Leithner, Sci. Rep., 2020, 10, 1682 CrossRef CAS.
- S.-H. Lee, N. Nomura and A. Chiba, Mater. Trans., 2008, 49, 260–264 CrossRef CAS.
- J. Qi, L. Ma, P. Gong and W. Rainforth, Wear, 2023, 518, 204649 CrossRef.
- K. Ueki, M. Kasamatsu, K. Ueda, Y. Koizumi, D. Wei, A. Chiba and T. Narushima, Metals, 2020, 10, 71 CrossRef CAS.
- Y. Chen, Y. Li, S. Kurosu, K. Yamanaka, N. Tang, Y. Koizumi and A. Chiba, Wear, 2014, 310, 51–62 CrossRef CAS.
- S.-H. Sun, Y. Koizumi, S. Kurosu, Y.-P. Li, H. Matsumoto and A. Chiba, Acta Mater., 2014, 64, 154–168 CrossRef CAS.
- Y. Zhang, W. Lin, Z. Zhai, Y. Wu, R. Yang and Z. Zhang, Mater. Sci. Eng., A, 2023, 862, 144449 Search PubMed.
- K. Prashanth and J. Eckert, J. Alloys Compd., 2017, 707, 27–34 CrossRef CAS.
- M. Zhang, Y. Yang, C. Song, Y. Bai and Z. Xiao, J. Alloys Compd., 2018, 750, 878–886 CrossRef CAS.
- F. Khorashadizade, S. Abazari, M. Rajabi, H. R. Bakhsheshi-Rad, A. F. Ismail, S. Sharif, S. Ramakrishna and F. Berto, J. Mater. Res. Technol., 2021, 15, 6034–6066 CrossRef CAS.
- S. Mazumder, K. Man, M. Radhakrishnan, M. V. Pantawane, S. Palaniappan, S. M. Patil, Y. Yang and N. B. Dahotre, Biomater. Adv., 2023, 150, 213415 CrossRef CAS.
- G. Raugei, G. Ramponi and P. Chiarugi, Cell. Mol. Life Sci., 2002, 59, 941–949 CrossRef CAS.
- P. Chiarugi, G. Pani, E. Giannoni, L. Taddei, R. Colavitti, G. Raugei, M. Symons, S. Borrello, T. Galeotti and G. Ramponi, J. Cell Biol., 2003, 161, 933–944 CrossRef CAS.
- M. N. Z. M. Noor, A. S. Alauddin, Y. H. Wong, C. Y. Looi, E. H. Wong, P. Madhavan and C. H. Yeong, Asian Pac. J. Cancer Prev., 2023, 24, 37 CrossRef CAS.
- X. Yang, Y. Li, Q. Huang, X. Liu, R. Zhang and Q. Feng, J. Biomed. Mater. Res., Part A, 2018, 106, 1822–1831 CrossRef CAS.
- M. Li, J. Yan, X. Chen, W. Tam, L. Zhou, T. Liu, G. Pan, J. Lin, H. Yang and M. Pei, J. Cell. Biochem., 2018, 119, 4928–4944 CrossRef CAS.
- Y. Arai, H. Park, S. Park, D. Kim, I. Baek, L. Jeong, B. J. Kim, K. Park, D. Lee and S.-H. Lee, J. Controlled Release, 2020, 328, 596–607 CrossRef CAS.
- M. Genestra, Cell. Signalling, 2007, 19, 1807–1819 CrossRef CAS.
- S. Li, R. Liao, X. Sheng, X. Luo, X. Zhang, X. Wen, J. Zhou and K. Peng, Front. Oncol., 2019, 696 CrossRef.
- Z. Fu and J. Zhang, J. Zhejiang Univ., Sci., B, 2022, 23, 102–122 CrossRef CAS.
- M. Y. Artamonov, A. K. Martusevich, F. A. Pyatakovich, I. A. Minenko, S. V. Dlin and T. W. LeBaron, Antioxidants, 2023, 12, 636 CrossRef CAS.
- A. Shao, H. Wu, Y. Hong, S. Tu, X. Sun, Q. Wu, Q. Zhao, J. Zhang and J. Sheng, Mol. Neurobiol., 2016, 53, 3462–3476 CrossRef CAS.
- S. N. Radyuk, Curr. Pharm. Des., 2021, 27, 626–735 CrossRef CAS.
|
This journal is © The Royal Society of Chemistry 2024 |