Ternary low-temperature phototherapy nano-systems for the treatment of diabetic wounds†
Received
23rd September 2023
, Accepted 29th November 2023
First published on 13th December 2023
Abstract
The physicochemical environment at the sites of chronic diabetic wounds is an ideal habitat for bacteria, which exacerbate the deterioration of the microenvironment at the wound sites and consequently delay wound healing. In recent years, photothermal therapy has been considered an ideal non-antibiotic antimicrobial strategy. However, photothermal therapy alone is prone to cause damage to the body tissues. Herein, a (zeolitic imidazolate framework-8) ZIF-8/(mesoporous polydopamine) MPDA@(deoxyribonuclease I) DNase I ternary nanocomposite system was constructed, which exhibited good antimicrobial and antioxidant properties. Specifically, DNase I was first encapsulated into MPDA nanoparticles (NPs) and then coated with ZIF-8, which rapidly degrades in an acidic bacterial environment, triggering the release of antimicrobial Zn2+ and DNase I, thus enabling low-temperature (∼45 °C) PTT antimicrobial therapy. Meanwhile, the NPs can effectively regulate the oxidative stress environment at the trauma site because of the antioxidant effect of MPDA. Moreover, the experimental results of the diabetic wound infection mouse model showed that the prepared NPs could kill bacteria well and accelerate wound healing. Overall, the phototherapy strategy proposed in this study shows great potential in the treatment of chronically infected wounds.
1. Introduction
Impaired wound healing is one of the most common complications of diabetes, which places a significant burden on both the patient and the healthcare system.1,2 The poor glucose metabolism of diabetic patients leads to the creation of a hyperglycemic microenvironment, which disrupts the normal wound-healing process.3,4 The hyperglycemic microenvironment provides a good habitat for the growth of bacteria.5–7 In addition, skin injuries in diabetic patients tend to heal slowly and are more susceptible to bacterial infections. Infection has serious negative effects on skin wound healing, such as inhibiting cell migration and mitochondrial function, and ultimately inducing apoptosis. Bacterial infection also alters the pH of the wound and promotes lactic acid accumulation, thus accelerating the deterioration of the diabetic wound microenvironment.8 Additionally, bacterial infection leads to a continuous inflammatory state at the wound site, which triggers an imbalance in the local redox state and the production of excess reactive oxygen species (ROS). High concentrations of ROS alter the structure and function of the extracellular matrix (ECM) and directly oxidize ECM components, causing fibroblast dysfunction and excessive matrix remodeling, further delaying the wound healing process.9,10 Antibiotic therapy is the main clinical modality for the treatment of diabetic wound infections.11 However, diabetic wounds often suffer from a mixture of multiple bacterial infections, which makes it difficult to cope with an antibiotic alone.12 Moreover, bacterial resistance is becoming more and more serious due to the massive misuse of antibiotics. Therefore, there is an urgent need to develop highly effective non-antibiotic broad-spectrum antibacterial strategies for the treatment of diabetic-infected wounds.
In recent years, photothermal therapy (PTT) has brought new hope for the treatment of bacterial infections due to its non-invasive and targeted treatment modality.13 Studies have shown that a good in vitro antimicrobial effect usually requires PTT to produce temperatures of 50 °C and higher. The high heat generated by PTT is capable of disrupting bacterial biofilm structures and bacterial DNA to achieve the antimicrobial effect.14 However, excessive temperature can also cause cell/tissue damage around the lesion. Therefore, when PTT is used for in vivo antimicrobial therapy, it is best to control the temperature below 45 °C.15 Polydopamine (PDA), a nanoparticle formed by the autoxidative polymerization of dopamine, has shown a series of advantages in antimicrobial therapy.16 First, PDA is biocompatible and biodegradable.17–19 Second, PDA itself can effectively convert light energy into heat energy, making it an ideal photothermal conversion agent.20,21 In addition, PDA has a unique p-phenylene structure, and in the presence of oxidizing agents, the reduced catechol moiety is readily oxidized to quinone by a proton-coupled process of two consecutive single-electron oxidations, which gives PDA an excellent ability to scavenge ROS and thus serves to alleviate oxidative stress in the microenvironment of the trauma site.22–25 Finally, mesoporous polydopamine (MPDA) is a novel structure of PDA, which possesses a higher specific surface area compared to conventional PDA, which results in better photothermal conversion efficiency, drug loading capacity, and antioxidant properties.25,26
Wound infection is a common complication in diabetic patients, where the interaction between pathogens and host tissues leads to the formation of a micro-acidic environment at the wound site.27 Taking advantage of this property, various acid-sensitive systems have been developed for the treatment of wound infections. Metal–organic frameworks (MOFs) have been widely studied and applied in gas storage, adsorption and separation, sensing, photocatalysis, water or gas contaminant removal, and electrochemistry due to their high specific surface area and tunable porous structure.28 Due to their low toxicity, excellent biodegradability, and easy functionalization, MOFs are also gradually applied to medical and biological fields.29 ZIF-8 is an important MOF consisting of Zn2+ bridged with a 2-methylimidazole ligand.30 Under acidic conditions of bacterial infection, ZIF-8 can be rapidly degraded to achieve a controlled release of Zn2+, which plays an important role in antibacterial and tissue repair processes.
Here, as shown in Fig. 1, we first synthesized MPDA NPs loaded with DNase I, followed by ZIF-8 coating, resulting in the preparation of a ternary nanosystem—ZIF-8/MPDA@DNase I. The ZIF-8 shell of ZIF-8/MPDA@DNase I, which is capable of degrading in the acidic environment of bacterial infection, allows for the controlled release of Zn2+ and DNase I. DNase I is a specific endonuclease that cleaves single- and double-stranded DNA during apoptosis. DNA cleavage occurs preferentially at the phosphodiester bond near the pyrimidine nucleotide. Related studies have reported that DNase I can kill bacteria and inhibit bacterial biofilm formation by cleavage of DNA.31 Based on the photothermal bactericidal effect of MPDA and the antibacterial effect of Zn2+ and DNase I, the nanosystem prepared in this study is expected to achieve efficient bacterial killing and effective biofilm removal under mild NIR irradiation. In addition, based on the unique paraben structure of MPDA, the system has excellent ROS scavenging ability, which can effectively reduce/mitigate oxidative stress damage at the trauma site and accelerate the damage repair process.
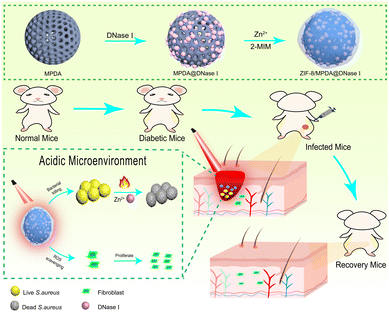 |
| Fig. 1 Schematic graph of the preparation of the ZIF-8/MPDA@DNase I system and wound repair of skin infection in diabetic mice. | |
2. Experimental section
2.1. Materials
2-Methylimidazole (purity 99.5%), DNase I, tris(hydroxymethyl)aminomethane (TRIS) (purity 99%), dopamine hydrochloride (purity 98%), 1,3,5-trimethylbenzene (TMB) (purity 97%) zinc nitrate hexahydrate (purity 98%), and Pluronic F127 were purchased from Aladdin (China).
2.2. Synthesis of ZIF-8/MPDA@DNase I NPs
MPDA NPs were synthesized using a one-pot method that has been reported in the literature.32 Briefly, 65 mL of deionized water and 60 mL of ethanol were added to a 250 mL volumetric flask. 0.36 g of F127 and 417 μL of TMB were added sequentially to the solution, which was dissolved by ultrasonication and stirred for 30 min at room temperature, followed by the addition of 0.06 g of dopamine hydrochloride and 0.09 g of Tris after dissolution. The crude product was collected after stirring at room temperature for 24 h. The MPDA NPs were obtained after centrifugation at 11
000 rpm several times.
1 mg of DNase I and 2 mg of MPDA was added to 5 mL of 10 mM MgCl2 in PBS solution, stirred for 4 h at room temperature, and then centrifuged to obtain MPDA@DNase I NPs. Then, 490 μL of 2-methylimidazole solution (0.021 g mL−1) was added and left to react at room temperature for 2 h. The ZIF-8/MPDA@DNase I NPs were collected by centrifugation.
2.3. Characterization of NPs
Transmission electron microscopy (TEM, Model FEI, Talos, F200S, Thermo Fisher Scientific, USA) was used to observe the structural morphology of ZIF-8/MPDA@DNase I NPs. X-ray photoelectron spectroscopy (XPS, Model PHI 5400, PerkinElmer Corporation, USA) was used to characterize the chemical composition of different sample surfaces and to study the changes of elemental composition in MPDA@DNase I and ZIF-8/MPDA@DNase I NPs after DNase I loading and coating with ZIF-8. Infrared spectroscopy (FTIR, Model 6300, Bio-Rad Corporation, USA) was used to analyze the infrared spectrograms of the NPs. Measurements of hydrated particle size and zeta potential were performed using the Malvern Zetasizer (Nano ZS, Malvern, UK).
2.4. Photothermal performance of NPs
The photothermal conversion ability of NPs was detected using an 808 nm laser, and real-time temperature changes were recorded using a digital thermometer (HH806AU, Omega, USA) with a thermally coupled probe. Different concentrations of NPs were ultrasonically dispersed in 1 mL of deionized water and then irradiated with the NIR laser to record the temperature changes over 10 min. The temperature changes in different samples were studied at different infrared optical densities (0.5 W cm−2, 1 W cm−2, 1.5 W cm−2). To study the temperature change of ZIF-8/MPDA@DNase I NPs in different concentrations (0.1 mg mL−1, 0.2 mg mL−1, and 0.5 mg mL−1) under 1 W cm−2 infrared density irradiation. The photothermal conversion stability of ZIF-8/MPDA@DNase I NPs was studied by irradiating 0.5 mg mL−1 ZIF-8/MPDA@DNase I NPs several times at 1 W cm−2 infrared density and recording the temperature changes. We also characterized the photothermal properties of ZIF-8/MPDA@DNase I NPs by using infrared thermography. 1 mL of deionized water and 1 mL of 0.2 mg mL−1 ZIF-8/MPDA@DNase I NPs were placed in a centrifuge tube and irradiated with an 808 laser for 10 min and the images and temperature changes were recorded.
2.5. Antioxidant properties of NPs
The total antioxidant capacity of ZIF-8/MPDA@DNase I was evaluated using ABTS and DPPH methods. ABTS was oxidized to ABTS+ in the presence of appropriate oxidants, and the production of ABTS+ was inhibited in the presence of antioxidants. A 7.4 mM solution of ABTS and a 2.6 mM solution of potassium persulfate were prepared and mixed in equal volumes. The NPs of each group were incubated with the prepared solution at 37 °C for 15 min, and the supernatant was taken after centrifugation and detected colorimetrically at 734 nm. The DPPH radical has a single electron and has a strong absorption at 515 nm. When antioxidants were present, the DPPH radical was scavenged and the absorbance at 515 nm decreased. The change of its absorbance in a certain range was proportional to the degree of radical scavenging. The NPs of each group at a concentration of 0.2 mg mL−1 were incubated with DPPH prepared with ethanol (0.1 mM) for 15 min at 37 °C away from light and centrifuged, and the supernatant was taken and colorimetrically detected at 515 nm.
2.6.
In vitro antimicrobial evaluation of NPs
S. aureus and E. coli were selected to evaluate the antibacterial effect of different NPs against Gram-positive and Gram-negative bacteria. The in vitro antibacterial effect of different NPs was evaluated using the plate counting method. The bacterial suspensions were mixed with different NPs (0.2 mg mL−1) in a well plate and incubated at 37 °C for 1 h. The light group was exposed to an 808 nm laser at 1 W cm−2 for 10 min. The well plate was then incubated at 37 °C for 6 h. Each sample was diluted 104 times with sterile PBS solution in a sterile ultra-clean table. 100 μL of the diluted solution was evenly applied to the agar plates and incubated overnight at 37 °C. Representative pictures of colony-forming units were taken with a camera and counted.
2.7. SEM observation
1 mL of E. coli and S. aureus suspensions with a concentration of 1 × 106 CFU mL−1 were added to the well plates containing titanium tablets, respectively. Liquid medium dissolved with different NPs (0.2 mg mL−1) was added to 1 mL per well, and the light group was irradiated with NIR for 10 min. The treated well plates were then incubated at 37 °C for 12 h. The supernatant of the medium was carefully aspirated in a sterile ultra-clean table and then washed three times with PBS. Then, equal amounts of paraformaldehyde (4 wt%) were added to each group until the titanium plates were completely submerged and placed in a refrigerator at 4 °C for 4 h. After fixation, the bacteria were dehydrated according to the following ethanol concentrations in order: 15%, 30%, 50%, 75% and 100%. Equal amounts of tert-butanol were added to each group, and each treatment was performed for 15 min. After drying at room temperature, gold sprayed and observed using SEM.
2.8. Live/dead staining of bacteria
E. coli and S. aureus suspensions at a concentration of 1 × 106 CFU mL−1 were inoculated on confocal dishes, and a liquid medium dissolved with different NPs (0.2 mg mL−1) was added to 1 mL for each group. 808 nm laser (1 W cm−2) was used to irradiate the light group for 10 min and incubated at 37 °C for 12 h. The supernatant of the medium was aspirated in a sterile ultra-clean table using a sterile. The sterile tip dropper was used to aspirate the medium supernatant, which was then washed thrice with sterile PBS. Live bacteria were stained green, and dead bacteria were stained red. The stained bacteria were observed by CLSM, and the images were collected and analyzed.
2.9. Biofilm crystalline violet staining
The biofilm incubation method followed the methods used in previous studies.15 The amount of biofilm was calculated by crystalline violet staining to assess the ability of each group of NPs to inhibit biofilm formation. 1 × 108 CFU mL−1 of S. aureus was added to 24-well plates containing different groups of NPs (0.2 mg mL−1). Then, the light group was irradiated with an 808 nm NIR laser (1 W cm−2) for 10 min and then incubated constantly at 37 °C for 48 h. The plates were gently washed three times with sterile PBS on a sterile ultra-clean table. Each well was stained with crystal violet (0.1%) for 10 min. The stained samples were washed gently with PBS three times. Finally, 500 μL of ethanol was added to each well, and the amount of biofilm production was assessed by detecting the absorbance of the samples at 590 nm.
2.10. Evaluation of biocompatibility and antioxidant properties of NPs
NIH-3T3 fibroblasts were used to assess the biocompatibility of NPs. NIH-3T3 fibroblasts were cultured with the high-sugar medium at 37 °C with 5% CO2. The cells were inoculated in well plates at a density of 2 × 104 cells per cm2 and incubated with different groups of NPs for 1 and 3 days. Then, the medium was removed, and 20 μL of CCK-8 solution and 0.2 mL of fresh serum-free high sugar medium were added to each well and incubated at 37 °C for 1 h. Cell viability was assessed by detecting the absorption value at 450 nm in each well. The cytocompatibility of different NPs was further evaluated by using the cell live/dead staining method. NIH-3T3 fibroblasts were inoculated in plates at a density of 2 × 104 cells per cm2 and incubated with different groups of NPs for 1 and 3 days. The culture medium supernatant was aspirated, and the cells were gently washed with sterile PBS solution 3 times. Then, 100 μL of FDA (100 μM) and 100 μL of PI (100 μM) were added successively to stain the cells in a light-proof environment for 10 min. Finally, all samples were observed by inverted fluorescence microscopy and photographed using a digital camera. The ability of different samples to scavenge intracellular ROS was determined using the ROS assay kit. NIH-3T3 fibroblasts were inoculated in confocal dishes at a density of 2 × 104 cells per cm2. An equal amount of high sugar medium was added and incubated in a constant temperature incubator for 24 h. Subsequently, the supernatant of the medium in the confocal dish was gently aspirated, and 1 mL of fresh high-sugar medium containing different NPs (0.2 mg mL−1) was added. After 24 h of incubation at a constant temperature, the medium was aspirated and washed three times using PBS. Then, the DCFH-DA probe was added under protection from light and stained for 25 min. Finally, all samples were observed using CLSM and the images of each group were recorded. The ability of ZIF-8/MPDA@DNase I NPs to scavenge H2O2 from the environment was measured using a ROS assay kit. NIH-3T3 fibroblasts with a density of 2 × 104 cells per cm2 were inoculated in 24-well plates with equal amounts of high sugar medium and then incubated in a constant temperature incubator at 37 °C for 24 h. The medium was aspirated, a fresh high-sugar medium containing different NPs was added, and NIH-3T3 cells were incubated for 24 h. The supernatant was collected, followed by the addition of H2O2 detection solution. The reaction was performed on a shaker at 37 °C for 25 min, and the UV absorption values of each group of solutions were measured at 560 nm using UV-vis.
2.11. Wound healing in diabetic skin
All experimental animal procedures were approved by the Animal Ethics Committee of the Third Military Medical University (SYXK-PLa-20120031). Mice were injected intraperitoneally with the STZ solution and fed a high-sugar and high-fat diet, and mice with a blood glucose level higher than 16.7 mmol L−1 were selected. The mice were fed high-sugar and high-fat chow for the following weeks. Eight groups of eight mice each were set up. They were the PBS group, the PBS + NIR group, the MPDA group, the MPDA + NIR group, the MPDA@DNase I group, the MPDA@DNase I + NIR group, the ZIF-8/MPDA@DNase I group and the ZIF-8/MPDA@DNase I + NIR group, respectively. The mice were anesthetized by preoperative intraperitoneal injection of 2 wt% sodium barbiturate solution. The hair was removed on the back of the mice using a shaving machine. The exposed dorsal area of the mice was cleaned and disinfected, and a circular wound of approximately 7 mm in diameter was cut in the back of each mouse using surgical scissors. 50 μL of S. aureus suspension (1 × 106 CFU mL−1) was taken and injected it into the wound to construct a traumatic infection model, and a certain amount of saline, MPDA, MPDA@DNase I and ZIF-8/MPDA@DNase I solution was added to the wound infection site, respectively. The wounds of the mice in the light group were irradiated with 808 nm NIR (1 W cm−2) for 4 min, and the real-time temperature was recorded by infrared thermography. The size of the infected wounds was recorded. Wound site tissues were collected after 7 and 21 days of wound establishment, and the bacterial elimination ability and wound healing ability were assessed by H&E staining and Masson trichrome staining.
After 7 days of wound establishment, skin tissue was collected from the wound site on the back of some mice and applied evenly on the wound surface using a sterile cotton swab moistened with saline and rotated continuously. Subsequently, the swab was immersed in a sterile MHB liquid medium and incubated overnight at 37 °C. Equal amounts of culture medium were taken from each group, diluted the same number of times for plate coating, and incubated at 37 °C overnight. Representative pictures of colony-forming units were taken with a camera and counted.
2.12. Biosafety evaluation
H&E sections were stained for the major organs (heart, liver, spleen, lungs, and kidneys) of the mice after 21 days of the experiment. Hemolysis experiments were also performed. Diabetic mice were anesthetized by intraperitoneal injection of a 2 wt% sodium barbiturate solution, then the eyeballs of the mice were removed with forceps, blood was collected from the eyes, fresh blood was added to PBS solution in drops, and the supernatant was discarded after several centrifugations (1000 rpm, 10 min) to collect purified erythrocytes. The purified erythrocytes were diluted to 5% (V/V). 1 mL of erythrocytes with 0.2 mg of MPDA, MPDA@DNase I and ZIF-8/MPDA@DNase I NPs was added, respectively, into a centrifuge tube, placed on a shaker at 37 °C and incubated for 1 h. The reaction mixture was centrifuged (1000 rpm) to remove the non-hemolyzed erythrocytes. 100 μL of supernatant was added to a 96-well plate, and the absorbance at 540 nm was detected using an enzyme marker. Triton X-100 at a concentration of 0.1% was used as a positive control, and PBS solution was used as a negative control.
2.13. Statistical analysis
Statistical analyses were performed by one-way analysis of variance (ANOVA) using Origin Pro (version 9.8). Confidence levels were set at 95% and 99%. All data were expressed as mean ± standard deviation (SD).
3. Results and discussion
3.1. Preparation and characterization of ZIF-8/MPDA@DNase I NPs
MPDA was synthesized by the one-pot method, loaded with DNase I, and then coated with ZIF-8. Transmission electron microscopy results showed that the synthesized MPDA NPs showed a regular spherical shape with clear morphology (Fig. 2(A)). After loading DNase I, the NP morphology did not change significantly (Fig. 2(B)). Compared with MPDA@DNase I, ZIF-8/MPDA@DNase I NPs had a well-defined ZIF-8 shell and a uniform distribution of Zn elements on the surface (Fig. 2(C) and (D)). The XPS spectrum (Fig. 2(E)) of ZIF-8/MPDA@DNase I showed a Zn2p3/2 peak at 1021.8 eV, while the binding energy peak at 1044.8 eV was attributed to Zn2p1/2. It also indicated that a ZIF-8 shell layer was formed on the surface of MPDA, which is consistent with literature reports.33 In the FTIR spectrum (Fig. 2(F)), the presence of absorption peaks at 3140 cm−1 corresponds to the C–H stretching vibration of the aromatic group of imidazole, and the absorption peak at 421 cm−1 is attributed to the Zn–N stretching vibration. The FTIR characterization results further confirm the successful encapsulation of the ZIF-8 shell layer. The zeta potential detection results indicated that the negative charge gradually decreased during the construction of ZIF-8/MPDA@DNase I NPs, which might be due to the positively charged ZIF-8 shell layer encapsulated on the surface of MPDA NPs (Fig. 2(G)). According to the literature, DNase I has the maximum emission wavelength at 308 nm due to the presence of residual tyrosine. Aqueous ZIF-8/MPDA@DNase I NPs showed a peak at 308 nm, which was similar to that of a pure DNase I solution (Fig. 2(H)).34 The particle size and particle size distribution of each batch of MPDA, MPDA@DNase I and ZIF-8/MPDA@DNase I NPs were examined using the dynamic light scattering (DLS) technique (Fig. S1, ESI†). The NPs gradually increased in size during the synthesis process.
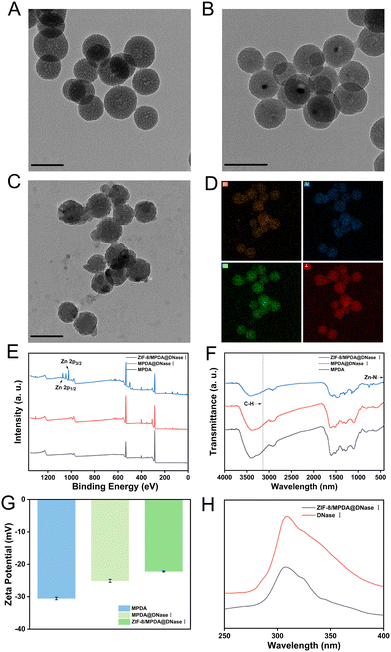 |
| Fig. 2 TEM images of (A) MPDA, (B) MPDA@DNase I and (C) ZIF-8/MPDA@DNase I NPs (scale bar: 200 μm); (D) elemental mapping of ZIF-8/MPDA@DNase I NPs; (E) XPS spectra of MPDA, MPDA@DNase I and ZIF-8/MPDA@DNase I NPs; (F) FTIR spectra of MPDA, MPDA@DNase I, and ZIF-8/MPDA@DNase I NPs; (G) zeta potentials of MPDA, MPDA@DNase I and ZIF-8/MPDA@DNase I NPs; (H) fluorescence patterns of DNase I and ZIF-8/MPDA@DNase INPs. Mean ± SD, n = 3. | |
3.2 Performance of ZIF-8/MPDA@DNase I NPs
MPDA NPs have good photothermal conversion properties, and we investigated the temperature changes in MPDA, MPDA@DNase I and ZIF-8/MPDA@DNase I NPs solutions under 808 nm NIR irradiation. As shown in Fig. 3(A) and (B), the temperature of the 0.2 mg mL−1 MPDA NPs solution increased rapidly from 25 °C to 45 °C after 10 min of 808 nm NIR irradiation (1 W cm−2). For the same concentration of MPDA@DNase I and ZIF-8/MPDA@DNase I NPs solutions, the temperature also increased from 25 °C to 45 °C after 10 min irradiation with 808 nm NIR (1 W cm−2). The above experimental results indicated that the loading of DNase I and the coating of the ZIF-8 shell layer did not affect the photothermal performance of MPDA NPs. The temperature of ZIF-8/MPDA@DNase I NPs solution increased to 37.1 °C after 10 min of NIR irradiation (0.5 W cm−2), and the temperature of ZIF-8/MPDA@DNase I NPs solution increased to 45.2 °C after 10 min of NIR irradiation (1 W cm−2). The temperature of the ZIF-8/MPDA@DNase I NPs solution increased to 52.4 °C after NIR irradiation (1.5 W cm−2) for 10 min (Fig. S2A, ESI†). The above experimental results demonstrated that the temperature change of ZIF-8/MPDA@DNase I NPs showed power density dependence. In addition, we examined the temperature rise of ZIF-8/MPDA@DNase I NPs at different concentrations upon NIR irradiation (1 W cm−2). When the concentration of ZIF-8/MPDA@DNase I NPs was 0.1 mg mL−1, the temperature increased to 38.9 °C after 10 min of NIR irradiation. When the concentration of ZIF-8/MPDA@DNase I NPs was 0.2 mg mL−1, the temperature increased to 45.2 °C after 10 min of NIR irradiation. When the concentration of NPs was 0.5 mg mL−1, the temperature increased to 50 °C after 10 min of NIR irradiation (Fig. S2B, ESI†). The above experimental results indicated that the temperature change of ZIF-8/MPDA@DNase I NPs showed concentration dependence. According to the heating–cooling curve, the photothermal conversion efficiency of ZIF-8/MPDA@DNase I NPs was calculated as about 20.3% according to the equation reported in the related study35 (Fig. S3, ESI†). In addition, as shown in Fig. 3(C), the temperature rise of ZIF-8/MPDA@DNase I NPs did not change much after three cycles of NIR irradiation, indicating that the prepared NPs have good photothermal stability.
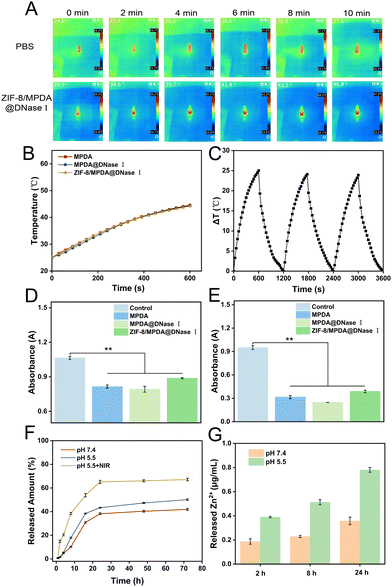 |
| Fig. 3 (A) Thermogram of 0.2 mg mL−1 ZIF-8/MPDA@DNase I NP solution under NIR irradiation; (B) temperature change of MPDA, MPDA@DNase I and ZIF-8/MPDA@DNase I NP solutions (0.2 mg mL−1) under NIR irradiation; (C) temperature changes of ZIF-8/MPDA@DNase I NP solution (0.5 mg mL−1) under NIR irradiation for 3 cycles; (D) DPPH radical scavenging ability of MPDA, MPDA@DNase I and ZIF-8/MPDA@DNase I NPs; (E) ABTS radical scavenging ability of MPDA, MPDA@DNase I and ZIF-8/MPDA@DNase I NPs; (F) the release behavior of ZIF-8/MPDA@DNase I NPs under different conditions of DNase I; (G) Zn2+ release from ZIF-8/MPDA@DNase I NPs at pH 7.4 and 5.5. Mean ± SD, n = 3, **p < 0.01. | |
Reactive oxygen can lead to oxidative damage of intracellular lipids, proteins and DNA, inducing oxidative stress.36 According to the relevant studies, MPDA materials are considered an ideal biomedical material because of their good biocompatibility and photothermal conversion ability, in addition to their excellent antioxidant properties. Therefore, we examined the ROS scavenging ability of different groups of NPs by DPPH and ABTS methods. MPDA NPs could effectively scavenge DPPH (Fig. 3(D)) and ABTS (Fig. 3(E)) radicals, and after DNase I loading and ZIF-8 shell layer modification, the ROS scavenging ability of NPs did not decrease significantly and still had excellent antioxidant properties. The encapsulation rate of DNase I was calculated to be 23.3% by multiple repetitions of the experiment. Related studies have shown that bacterial infection causes a weakly acidic wound microenvironment. Therefore, in vitro drug release mimics the weakly acidic environment during bacterial infection. The experiments were divided into three groups: pH 7.4, pH 5.5 and pH 5.5 + NIR. As shown in Fig. 3(F), the experimental results showed that the percentage of DNase I released in the pH 7.4 group was about 41.0% within 72 h. In the pH 5.5 group, the percentage of DNase I release was about 50.3%, which indicated that the decomposition of ZIF-8 in the acidic environment accelerated the release rate of DNase I. The percentage of DNase I release in the pH 5.5 + NIR group was 67.1%, which may be due to the acidic environment accelerating the decomposition of the ZIF-8 shell as well as the elevated temperature accelerating the decomposition of MPDA, which accelerated the release rate of DNase I. The release rate of Zn ions at pH = 5.5 was significantly faster than that at pH = 7.4 within 24 h, which is consistent with that described in the literature (Fig. 3(G)).37 This demonstrates that ZIF-8/MPDA@DNase I NPs can achieve pH-responsive release of Zn2+.
3.3.
In vitro antibacterial ability of ZIF-8/MPDA@DNase I NPs
Diabetic wound infections not only have a high incidence but also are caused by a variety of pathogenic species, often mixed bacterial infections. We used E. coli and S. aureus, representing Gram-negative and Gram-positive bacteria, respectively, for assessing the in vitro antibacterial effect of the prepared NPs. As shown in Fig. 4(A), the survival rates of S. aureus after treatment with 0.2 mg mL−1 of MPDA, MPDA@DNase I and ZIF-8/MPDA@DNase I NPs were 90.3%, 75.7% and 47.4%, respectively, when compared with the control group without NIR irradiation. The bacterial survival in the MPDA@DNase I group was lower than that in the MPDA group. This indicates that MPDA@DNase I NPs release DNase I and have some antibacterial effect. The bacterial survival rate of ZIF-8/MPDA@DNase I NPs was further decreased compared with the MPDA@DNase I group. This suggests that Zn2+ produced a combined bactericidal effect with DNase I. Similarly, in the absence of NIR irradiation, the survival of E. coli after treatment with 0.2 mg mL−1 of MPDA, MPDA@DNase I and ZIF-8/MPDA@DNase I NPs was 85.1%, 59.9% and 57.0%, respectively, and the survival rates of S. aureus were 90.3%, 75.7% and 47.4%, respectively. After 10 min of 808 nm NIR irradiation, the survival rates of S. aureus in the MPDA, MPDA@DNase I and ZIF-8/MPDA@DNase I NPs groups were 82.8%, 37.8% and 3.2%, respectively, and the survival rates of E. coli were 68.5%, 53.9% and 0.3%, respectively (Fig. S4, ESI†). After NIR irradiation, the survival rate of each group of bacteria showed a significant decrease, which indicated that the high temperature generated by NIR irradiation caused the death of bacteria. Taken together, we conclude that the prepared core–shell nanosystem initially achieved the desired combined antibacterial effect of DNase I, Zn2+ and PTT. The results of live/dead staining of bacteria (Fig. 4(B)) demonstrated that the ZIF-8/MPDA@DNase I NPs exhibited excellent antibacterial properties under NIR irradiation. The control group had a large number of live bacteria (green) and a very small amount of dead bacteria (red). The CLSM images of the MPDA@DNase I and ZIF-8/MPDA@DNase I groups showed a significant decrease in the number of live bacteria and an increase in the red fluorescence of dead bacteria. Under NIR irradiation, the number of dead bacteria further increased, especially in the ZIF-8/MPDA@DNase I NP group. The SEM results showed (Fig. 4(C)) that the outer wall of the bacteria in the control group was intact and more numerous, and their morphology was not significantly damaged after NIR irradiation. In the absence of NIR irradiation, the bacteria in the MPDA, MPDA@DNase I and ZIF-8/MPDA@DNase I groups, all showed a gradual decrease, which was consistent with the results of the plate counting method described above. Under NIR irradiation, the bacteria in the MPDA, MPDA@DNase I and ZIF-8/MPDA@DNase I groups decreased substantially and showed rupture of the bacterial cell wall membrane. This may be due to the high temperature generated by PTT that disrupts the bacterial wall membrane. The hyperglycemic microenvironment of diabetes creates good conditions for bacteria to proliferate and adhere. Once an infection occurs at the wound site, bacteria rapidly adhere and colonize, subsequently forming a biofilm with a three-dimensional structure on the tissue surface. The biofilm is composed of EPS produced by bacterial secretion, and the main components of EPS are proteins, polysaccharides eDNA, etc. eDNA plays an important role in the formation of biofilms and can maintain the stability of the biofilm framework.35,38 The ability of various NPs to inhibit the biofilm of S. aureus was evaluated by using crystalline violet staining. The MPDA@DNase I and ZIF-8/MPDA@DNase I groups had good biofilm inhibition ability with/without NIR irradiation compared to the PBS and MPDA groups. This indicates that DNase I released from MPDA@DNase I and ZIF-8/MPDA@DNase I NPs had a cleavage effect on the bacteria-secreted eDNA, thus inhibiting the formation of bacterial biofilm. Furthermore, the biomass within the bacterial biofilm was counted and calculated, and the biofilm formation in both MPDA@DNase I and ZIF-8/MPDA@DNase I groups was significantly less than the control group with/without NIR irradiation (Fig. 4(D) and (E)). This demonstrated that ZIF-8/MPDA@DNase I NPs could inhibit biofilm formation by scavenging bacterial eDNA.
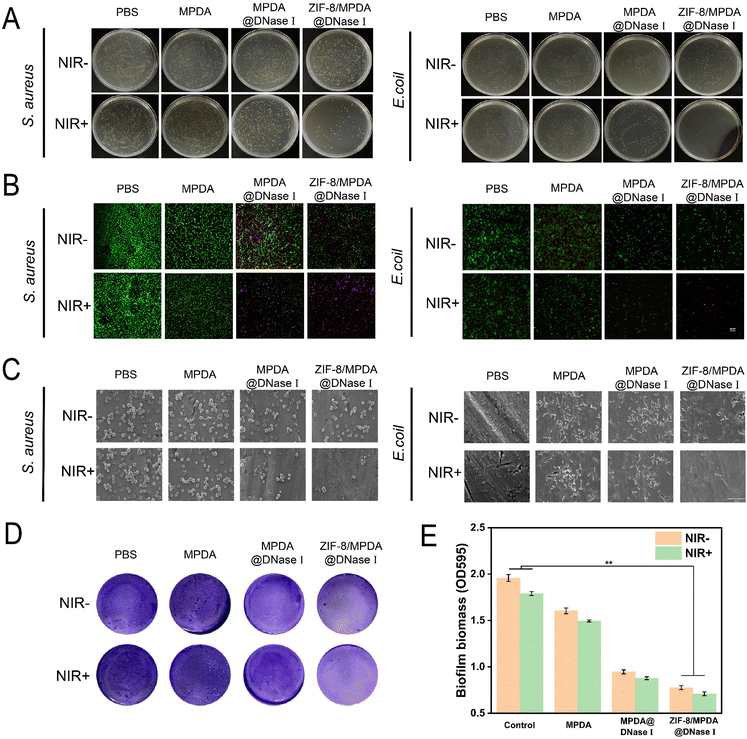 |
| Fig. 4 (A) Photographs of a typical agar plate with S. aureus and E. coli colonies following various treatments; (B) CLSM images of S. aureus and E. coli after different treatments (scale bar: 20 μm); (C) SEM images of S. aureus and E. coli after different treatments (scale bar: 10 μm); (D) crystalline violet staining photographs and (E) relative biomass of biofilms after different treatments. Mean ± SD, n = 3, **p < 0.01. | |
3.4.
In vitro cytocompatibility and antioxidant properties of ZIF-8/MPDA@DNase I NPs
NIH-3T3 cells were co-cultured with NPs. As shown in Fig. 5(A) and (B), there was no significant change in cell morphology of NIH-3T3 in MPDA, MPDA@DNase I and ZIF-8/MPDA@DNase I groups compared to the control group. After 1 day of culture, the cell activity decreased to a lesser extent compared to the control group. After 3 days of culture, the cell viability of the MPDA@DNase I and ZIF-8/MPDA@DNase I groups showed some degree of increase. The intracellular ROS scavenging ability of different samples was measured using a DCFH-DA probe. The CLSM results showed (Fig. 5(C)) that the green fluorescence of MPDA, MPDA@DNase I and ZIF-8/MPDA@DNase I groups was significantly reduced relative to the control group, which indicated that the intracellular ROS was effectively scavenged by NPs. In addition, the scavenging ability of ZIF-8/MPDA@DNase I NPs in H2O2 environment was also determined. Compared with the control group (Fig. 5(D)), the concentration of H2O2 in the medium of MPDA, MPDA@DNase I and ZIF-8/MPDA@DNase I groups decreased, which indicated that the prepared NPs could effectively scavenge H2O2 from the environment. The excellent ROS scavenging ability of the NPs prepared in this study was mainly attributed to the antioxidant ability of MPDA.
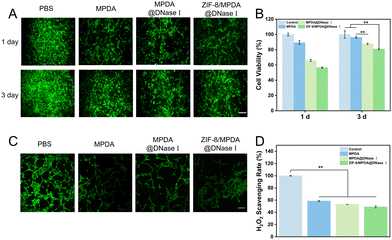 |
| Fig. 5 (A) Live/dead staining images and (B) cell viability of NIH-3T3 cells after 1 and 3 days of culture after different treatments (scale bar: 100 μm); (C) representative fluorescence images of intracellular ROS after different treatments (scale bar: 100 μm); (D) efficiency of H2O2 removal from the environment after different treatments. Mean ± SD, n = 3, **p < 0.01. | |
3.5.
In vivo antibacterial and repair-promoting abilities of ZIF-8/MPDA@DNase I NPs
The temperature change in ZIF-8/MPDA@DNase I NPs under NIR irradiation was detected. The temperature at the wound site in the ZIF-8/MPDA@DNase I group increased to 45.8 °C after 4 min of NIR irradiation. In contrast, there were no significant changes in wound temperature in the PBS group (Fig. 6(A) and (B)). After 7 days of treatment, skin tissues were collected from each group and the in vivo antimicrobial effect of NPs in each group was evaluated by the standard plate counting method. The survival rates of S. aureus in the MPDA, MPDA@DNase I and ZIF-8/MPDA@DNase I groups were 86.8%, 76.2% and 56.6%, respectively, compared with the control group. Under NIR irradiation, the survival rates of S. aureus in the MPDA, MPDA@ DNase I and ZIF-8/MPDA@DNase I groups were 70.8%, 46.0% and 21.6%, respectively (Fig. 6(C) and (D)). As shown in Fig. 6(E), after 21 days of treatment, the wound area of mice in the ZIF-8/MPDA@DNase I + NIR group became significantly smaller. Quantitative analysis showed (Fig. 6(F)) that the relative wound area of the MPDA group decreased to 18.7% after 21 days of treatment. This may be due to the antioxidant effect of MPDA, which cleared ROS from the wound site and thus accelerated the wound healing. The wound healing effect of the MPDA + NIR group was better than that of the MPDA@DNase I group, which may be due to the high PTT antibacterial effect stronger than that of DNase I. The relative wound area of the ZIF-8/MPDA@DNase I group decreased to 8.3%, according to the literature that Zn2+ has a good antibacterial ability and has a wound healing promoting effect. Therefore, we speculate that the release of Zn2+ accelerated the wound healing. The relative wound area of the ZIF-8/MPDA@DNase I + NIR group was the smallest, which was about 5%, indicating that the wound repair effect was most pronounced in the ZIF-8/MPDA@DNase I + NIR group. This was mainly due to the combined antimicrobial effect of photothermal therapy (PTT), DNase I, and Zn2+, resulting in improved infection treatment and accelerated wound healing.
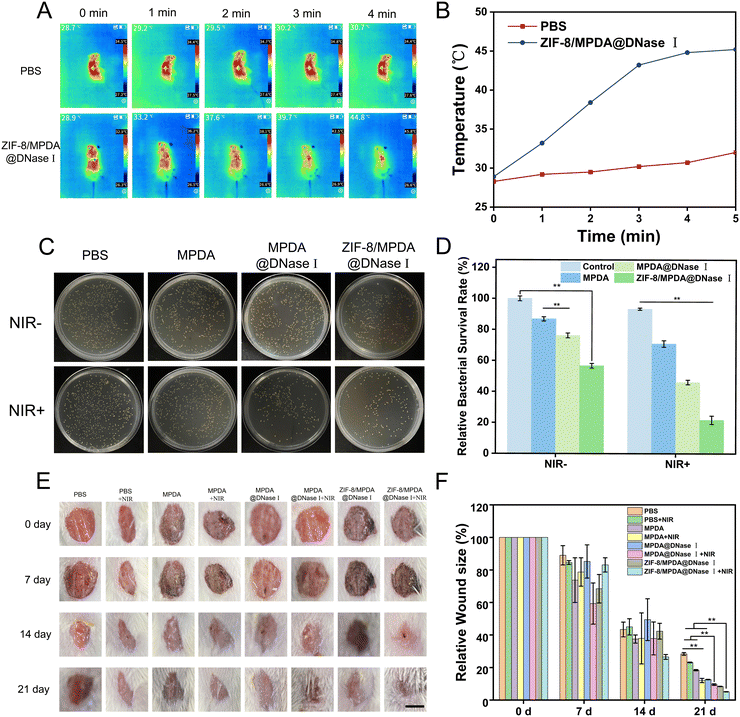 |
| Fig. 6 (A) and (B) NIR irradiation (1 W cm−2) of S. aureus infected wounds of Balb/c mice treated with PBS and ZIF-8/MPDA@DNase I NPs in thermograms and temperature change profiles. (C) photographs of a typical agar plate with S. aureus colonies following various treatments and (D) survival rate after different treatments. (E) photographs of S. aureus-infected wounds from various groups at varying treatment times (scale bar: 5 mm) and (F) relative wound size of the mice. Mean ± SD, n = 3, **p < 0.01. | |
3.6. Histological analysis
Skin tissue sections were selected for H&E staining after 7 and 21 days postoperatively. Microscopic observation showed (Fig. 7(A)) that inflammatory cell infiltration at the wound site was significantly reduced and the epidermis was more intact in the ZIF-8/MPDA@DNase I + NIR group compared to the control group. This indicated that the ZIF-8/MPDA@DNase I + NIR group had a reduced inflammatory response at the wound site and better wound healing. The amount of de novo collagen deposition in tissue sections at 7 and 21 days after treatment in each group was analyzed using Masson triple staining. The results showed (Fig. 7(B)) that under NIR irradiation conditions, more collagen deposition occurred in ZIF-8/MPDA@DNase I nanoparticle-treated wounds. This suggests that the ZIF-8/MPDA@DNase I + NIR group can significantly promote tissue repair.
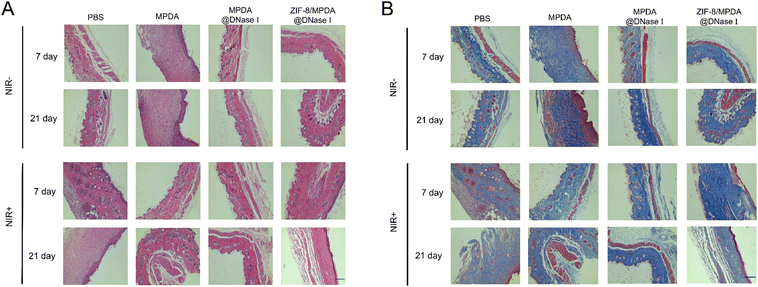 |
| Fig. 7 (A) H&E and (B) Masson trichrome staining images of skin tissues after different treatments (scale bar: 200 μm). | |
3.7. Biosafety evaluation
After 21 days of treatment, the internal organs (heart, liver, spleen, lungs and kidneys) of all groups of mice were analyzed by H&E staining (Fig. 8(A)). The results showed that both ZIF-8/MPDA@DNase I NPs and NIR irradiation did not cause any damage or toxicity to various organs during the treatment period. This demonstrates the good biosafety of ZIF-8/MPDA@DNase I NPs, which provides an effective and safe strategy for the treatment of diabetic wound infections. The hemocompatibility of ZIF-8/MPDA@DNase I NPs was examined by incubating them with fresh red blood cells. Complete hemolysis occurs in the positive control (1% Triton X-100) group. However, no hemolysis was detected in the ZIF-8/MPDA@DNase I NP (0.2 mg mL−1) group, and no significant hemolysis was observed in the MPDA and MPDA@DNase I groups (Fig. 8(B)). This indicates that the NPs prepared in this study have good hemocompatibility.
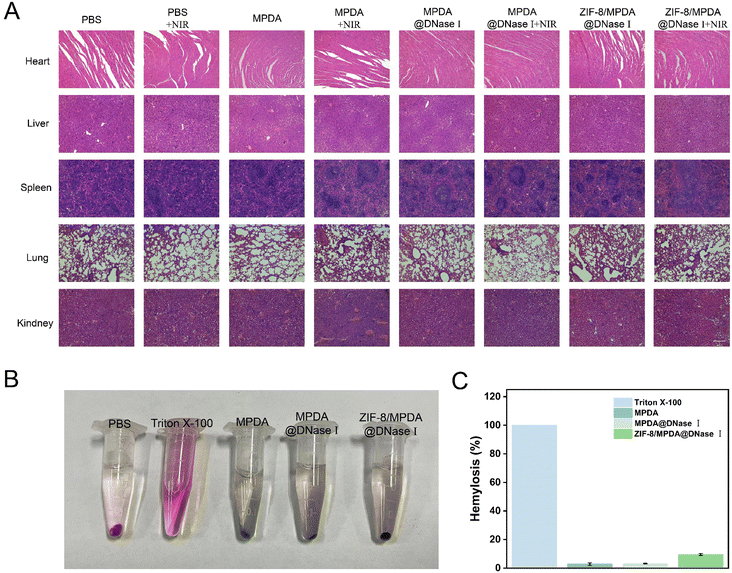 |
| Fig. 8 (A) H&E staining images of heart, liver, spleen, lungs and kidney after 21 days of treatment (scale bar: 200 μm); (B) photographs of erythrocyte suspensions and hemolysis analysis of different treated mice. Triton X-100 (1%) was used as a positive control (100%) and PBS was used as a negative control (0%). Mean ± SD, n = 4. | |
4. Conclusions
In summary, we synthesized a triple nanocomposite system by loading DNase I inside the MPDA NPs and encapsulating ZIF-8 outside, which can promote the healing of diabetic-infected chronic wounds through antimicrobial and antioxidant properties. Compared with single photothermal therapy, the ZIF-8/MPDA@DNase I NPs were able to rapidly release Zn2+ and DNase I under acidic conditions of bacterial infection, and such an antimicrobial mode has been demonstrated to achieve low-temperature and efficient antimicrobial activity in vitro and in vivo, thus avoiding damage to normal tissues caused by excessively high temperatures. Importantly, the nanosystem has good biocompatibility and antioxidant properties, which can promote the healing of diabetic chronic wounds. In conclusion, the constructed ZIF-8/MPDA@DNase I ternary nanocomposite system provides a feasible strategy for the clinical treatment of diabetic chronic wounds.
Conflicts of interest
There are no conflicts to declare.
Acknowledgements
The authors acknowledge the financial support from the National Natural Science Foundation of China (32171327 and 51825302), the State Key Project of Research and Development (2022YFB3804400), the Postdoctoral Science Foundation of China (2021M693735), the Natural Science Foundation of Chongqing (cstc2021jcyj-cxttX0002), and the Fundamental Research Funds for the Central Universities (2023CDJXY-051). The authors acknowledge the Analytical and Testing Center of Chongqing University for the help with the characterization of materials.
References
- M. Q. Shi, Z. Du, Y. C. Qi, W. L. Li, H. Q. Hu, X. H. Lin, S. J. Wang, Z. Tang and M. Zhou, Theranostics, 2022, 12, 6 Search PubMed.
- S. S. Garg, R. Dubey, S. Sharma, A. Vyas and J. Gupta, Int. J. Biol. Macromol., 2023, 125636 CrossRef CAS.
- W. Liu, X. X. Zhai, X. Zhao, Y. J. Cai, X. M. Zhang, K. Xu, J. Weng, J. S. Li and X. Y. Chen, Adv. Healthcare Mater., 2023, e2300297 CrossRef PubMed.
- J. Yang, Z. Y. Chu, Y. C. Jiang, W. Zheng, J. W. Sun, L. L. Xu, Y. Ma, W. N. Wang, M. Shao and H. S. Qian, Adv. Healthcare Mater., 2023, e2300725 CrossRef PubMed.
- X. Zhou, B. B. Zhao, L. L. Wang, L. F. Yang, H. Chen, W. Chen, H. S. Qiao and H. L. Qian, J. Controlled Release, 2023, 359 Search PubMed.
- F. Huang, X. Y. Lu, Y. Yang, Y. S. Yang, Y. Y. Li, L. Kuai, B. Li, H. Q. Dong and J. L. Shi, Adv. Sci., 2023, 10, 2 Search PubMed.
- J. H. Chen, X. F. Bao, T. Meng, J. Sun and X. R. Yang, Chem. Eng. J., 2022, 430 Search PubMed.
- B. L. Tao, C. C. Lin, Y. He, Z. Yuan, M. W. Chen, K. Xu, K. Li, A. Guo, K. Y. Cai and L. X. Chen, Chem. Eng. J., 2021, 423 Search PubMed.
- Q. S. Deng, Y. Gao, B. Y. Rui, X. R. Li, P. L. Liu, Z. Y. Han, Z. Y. Wei, C. R. Zhang, F. Wang, H. Dawes, T. H. Zhu, S. C. Tao and S. C. Guo, Bioact. Mater., 2023, 27 Search PubMed.
- C. W. Wang, Y. H. Liu, X. X. Yang, W. T. Li, X. H. Zhou, Y. Ren, C. R. Zhang, H. Yang, W. Q. Kong, J. W. Wang and H. Y. Niu, Chem. Eng. J., 2022, 450 Search PubMed.
- B. A. Lipsky, P. D. Baker, G. C. Landon and R. Fernau, Clin. Infect. Dis., 1997, 24, 4 CrossRef.
- O. Kandemir, E. Akbay, E. Sahin, A. Milcan and R. Gen, J. Infect., 2007, 54, 5 CrossRef.
- J. W. Hu, Y. Ding, B. L. Tao, Z. Yuan, Y. L. Yang, K. Xu, X. Li, P. Liu and K. Y. Cai, Bioact. Mater., 2022, 18 Search PubMed.
- J. J. Huo, Q. Y. Jia, H. Huang, J. Zhang, P. Li, X. C. Dong and W. Huang, Chem. Soc. Rev., 2021, 50, 15 RSC.
- D. Peng, G. H. Liu, Y. He, P. F. Gao, S. Q. Gou, J. Wu, J. X. Yu, P. Liu and K. Y. Cai, Biomater. Sci., 2021, 9, 22 Search PubMed.
- Y. L. Yu, J. J. Wu, C. C. Lin, X. Qin, F. R. Tay, L. Miao, B. L. Tao and Y. Jiao, Mil. Med. Res., 2023, 10, 1 Search PubMed.
- G. H. Liu, L. Wang, Y. He, L. C. Wang, Z. W. Deng, J. J. Liu, D. Peng, T. Ding, L. Lu, Y. Ding, J. X. Zhang, P. Liu and K. Y. Cai, Adv. Healthcare Mater., 2021, 10, 23 Search PubMed.
- J. K. Zeng, C. J. Gu, X. W. Geng, K. L. Lin, Y. Z. Xie and X. S. Chen, Biomaterials, 2023, 297 Search PubMed.
- Z. H. Han, M. J. Gao, Z. H. Wang, L. C. Peng, Y. B. Zhao and L. Sun, J. Drug Delivery Sci. Technol., 2022, 75 Search PubMed.
- K. Xu, Z. Yuan, Y. Ding, Y. He, K. Li, C. C. Lin, B. L. Tao, Y. L. Yang, X. Li, P. Liu and K. Y. Cai, Appl. Mater. Today, 2021, 24 Search PubMed.
- Y. Xiao, M. R. Xu, N. Lv, C. Cheng, P. Huang, J. B. Li, Y. Hu and M. Sun, Acta Biomater., 2021, 122 Search PubMed.
- M. Hussain, H. N. Suo, Y. Xie, K. Wang, H. Wang, Z. Y. Hou, Y. J. Gao, L. B. Zhang, J. Tao, H. Jiang and J. T. Zhu, ACS Appl. Mater. Interfaces, 2021, 13, 25 Search PubMed.
- Y. Fu, J. H. Zhang, Y. L. Wang, J. Li, J. X. Bao, X. Xu, C. Q. Zhang, Y. W. Li, H. X. Wu and Z. P. Gu, Carbohydr. Polym., 2021, 257 Search PubMed.
- P. Jiang, A. Choi and K. E. Swindle-Reilly, Nanoscale, 2020, 12, 33 Search PubMed.
- N. Y. Chen, S. C. Yao, M. M. Li, Q. Y. Wang, X. X. Sun, X. Feng and Y. Chen, Biomacromolecules, 2023, 24, 4 Search PubMed.
- W. W. Tan, T. Long, Y. Z. Wan, B. C. Li, Z. L. Xu, L. Zhao, C. D. Mu, L. M. Ge and D. F. Li, Carbohydr. Polym., 2023, 312 Search PubMed.
- F. J. Zhang, Q. S. Hu, Y. Wei, W. L. Meng, R. Wang, J. Z. Liu, Y. Nie, R. F. Luo, Y. B. Wang and B. Shen, Chem. Eng. J., 2022, 435 Search PubMed.
- X. Li, X. Y. Shu, Y. X. Shi, H. L. Li and X. B. Pei, Chin. Chem. Lett., 2023, 34, 7 Search PubMed.
- Z. Y. Sun, T. Y. Li, T. X. Mei, Y. Liu, K. R. Wu, W. J. Le and Y. H. Hu, J. Mater. Chem. B, 2023, 11, 15 Search PubMed.
- B. L. Tao, W. K. Zhao, C. C. Lin, Z. Yuan, Y. He, L. Lu, M. W. Chen, Y. Ding, Y. L. Yang, Z. Z. L. Xia and K. Y. Cai, Chem. Eng. J., 2020, 390 Search PubMed.
- K. A. Lacey, L. Serpas, S. Makita, Y. Wang, A. Rashidfarrokhi, C. Soni, S. Gonzalez, A. Moreira, V. J. Torres and B. Reizis, J. Exp. Med., 2023, 220, 6 CrossRef.
- Z. Yuan, B. L. Tao, Y. He, C. Y. Mu, G. H. Liu, J. X. Zhang, Q. Liao, P. Liu and K. Y. Cai, Biomaterials, 2019, 223 Search PubMed.
- Y. Hüsnü Arda and Ç. Ali Emrah, Colloids Surf., A, 2021, 625 Search PubMed.
- S. Tian, L. Z. Su, Y. L. An, H. C. van der Mei, Y. J. Ren, H. J. Busscher and L. Q. Shi, Chem. Eng. J., 2022, 452 Search PubMed.
- Z. Yuan, C. C. Lin, L. L. Dai, Y. He, J. W. Hu, K. Xu, B. L. Tao, P. Liu and K. Y. Cai, Small, 2021, 17, 13 Search PubMed.
- D. J. Yang, Y. Han, Y. L. Wang, Y. Y. Pan, L. Zheng, Z. G. Liu, C. Li, Y. L. Wu and H. Q. Li, Chem. Eng. J., 2023, 469 Search PubMed.
- G. Xie, S. Du, Q. Y. Huang, Q. Hu, D. H. Bi, B. L. Peng, J. Tao, L. B. Zhang and J. T. Zhu, ACS Appl. Mater. Interfaces, 2022, 15, 1 Search PubMed.
- J. J. Wu, P. Shen, X. Qin, Y. L. Yang, C. C. Lin, X. Li, W. B. Geng, P. F. Gao, L. X. Chen, L. Miao, Y. Jiao and B. L. Tao, Chem. Eng. J., 2023, 459 Search PubMed.
|
This journal is © The Royal Society of Chemistry 2024 |