DOI:
10.1039/D4TA00665H
(Paper)
J. Mater. Chem. A, 2024,
12, 11848-11856
Enhancing CO2 gasification-reforming of municipal solid waste with Ni/CeO2 and Ni/ZrO2 catalysts†
Received
29th January 2024
, Accepted 15th April 2024
First published on 22nd April 2024
Abstract
The global energy crisis and environmental sustainability challenges are exacerbated by the rapid increase in population and industrialization, necessitating effective management of municipal solid waste. The CO2 gasification-reforming of municipal solid waste with Ni/CeO2 and Ni/ZrO2 catalysts was conducted in a two-stage fixed-bed reactor. A significant increase in gas production from various waste samples (cabbage, poplar leaves, printed paper, PET, and HDPE) was observed, with the 5% Ni/CeO2 demonstrating higher efficiency than the 5% Ni/ZrO2 catalyst. The structural characterization of the catalysts revealed that Ni was more uniformly dispersed on the CeO2 support compared to ZrO2, resulting in enhanced activity of the 5% Ni/CeO2 catalysts. Further exploration into the optimal nickel loading and the ideal reforming temperature was conducted to maximize the efficiency of the CO2 gasification-reforming. The application of 5% Ni/CeO2 catalysts in the CO2 gasification-reforming of simulated municipal solid waste notably increased CO and total gas yields by 223% and 106%, respectively. This advancement holds promise for new technical approaches in resource utilization and the environmentally friendly processing of municipal solid waste.
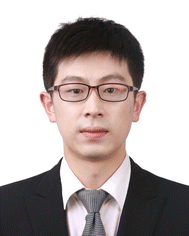 Hui Zhou | Dr Hui Zhou is an Associate Professor and the Director of the Solid Waste and Carbon Cycle Utilization Research Center at Department of Energy and Power Engineering, Tsinghua University, China. His research primarily focuses on heterogeneous catalysis for the thermochemical conversion of solid waste and renewable carbon resources. He has received the Leading Young Scientist of the Nation and the ACS Sustainable Chemistry & Engineering Lectureship Awards. He has served as the Chair for the International Conference on Carbon Capture Science and Technology in 2023. Currently, he is the Executive Editor for the journal Carbon Capture Science & Technology. |
1. Introduction
The escalation in population and rapid industrialization are precipitating a global energy crisis and challenges in environmental sustainability.1,2 The management of municipal solid waste (MSW) is increasingly becoming a critical issue. It is estimated that over two billion tonnes of municipal solid waste are generated globally each year, and this amount is expected to rise sharply in the future.3 Alarmingly, at least a third of this waste is not processed in an environmentally benign manner.4 Comprising predominantly hydrocarbons, municipal solid waste represents a source of renewable energy.5 Traditional waste management methods, such as landfilling and incineration, offer partial solutions by reducing waste volume but often result in energy wastage and environmental pollution.6,7 In contrast, the transformation of municipal solid waste into syngas through gasification emerges as a more sustainable and eco-friendlier alternative.8
Gasification is a process that transforms organic matter into carbon monoxide and hydrogen under high temperatures and a controlled oxygen environment.9 The gasification of municipal solid waste not only reduces the total volume of waste but also enables the recovery of energy, thereby alleviating urban energy pressures.10 Employing CO2 as a gasifying agent facilitates the production of CO-rich syngas, while simultaneously offering a viable method for CO2 utilization.11 To achieve the global objective of reducing CO2 emissions by 15–20% by 2050, the development of CO2 utilization technologies is imperative.12,13 A primary challenge in the CO2 gasification of municipal solid waste is the incomplete cracking and re-polymerization of hydrocarbons, leading to the formation of tar.14 The presence of tar degrades the quality of the syngas and limits its applications.15 Additionally, tar can clog gasifier pipelines, reducing gasification efficiency, and increasing maintenance costs.16
Enhancing the efficiency of tar CO2 reforming through the addition of catalysts is identified as the most effective method for tar removal, and it also increases the yield of syngas.17 Nickel-based catalysts have attracted widespread interest due to their high catalytic activity and cost-effectiveness.18 Nickel-based catalysts can facilitate the breaking of C–C, C–H, and O–H bonds in tar.19 The supports of catalysts play a crucial role in the catalytic performance by improving the dispersion of metal.20,21 Common supports of nickel-based catalysts include Al2O3, SiO2, ZrO2, and CeO2.22–25 ZrO2 exhibits excellent thermal stability and redox properties and can generate strong interaction with loaded metal, making Ni/ZrO2 demonstrate superior catalytic performance in various thermochemical reactions, such as CO2 methanation and CH4 reforming.26,27 It was reported that the reduction of ZrO2 by H2 led to the formation of oxygen vacancies, thus enhancing the catalytic activity of ZrO2-supported catalysts.28 In addition, ZrO2 improved the activity of the nickel-based catalyst in the methane dry reforming at low temperatures.29 CeO2, distinguished by its high oxygen storage capacity and effective oxygen mobility has emerged as an efficient catalyst support in the realm of heterogeneous catalysis.30,31 Liu et al.32 reported that Ni/CeO2 showed good activity and stability during methane dry reforming, which was due to the strong metal-support interactions and the ease of gasification of carbon deposited on the catalysts.
In this work, a comparative analysis was conducted on the catalytic performance of Ni catalysts supported on different supports (CeO2 and ZrO2) in the CO2 gasification-reforming process of municipal solid waste. Through the structural characterization of catalysts, the relationship between the structure and activity of catalysts was revealed. Additionally, the optimal nickel loading on the catalysts and the ideal reforming temperature for the reaction were further explored to maximize the catalyst's effectiveness in the CO2 gasification-reforming process. This study will provide new technical pathways for the resource utilization and environmentally friendly treatment of municipal solid waste.
2. Materials and methods
2.1. Materials
PET and HDPE were both procured from Yangli Electromechanical Technology Co., Ltd. Poplar leaves were gathered from the trees on the Tsinghua University campus in Beijing, China, and the cabbages and paper were sourced from the supermarket at Tsinghua University. Municipal solid waste predominantly comprises approximately 62.6% food waste, 16.9% plastics, 12.8% paper, 4.7% textiles, and 3.0% leaves.33 In this study, cabbage was selected to represent food waste, HDPE was chosen as a surrogate for plastics, and PET (Polyester) stood in for textiles. The simulated municipal solid waste consists of 62.6% cabbage, 16.9% HDPE, 12.8% printed paper, 4.7% PET, and 3.0% poplar leaves. Before the experiments, each sample underwent a thorough preparation process, including drying at 105 °C overnight, followed by grinding and sieving. Table S1† displays the approximate and ultimate analyses of all samples.
2.2. Preparation of catalysts
The CeO2 and ZrO2 supports were synthesized by the precipitation method. For ZrO2 supports, 17.5 mmol of Zr(NO3)4·6H2O (99.99%, Macklin) was dissolved in 350 mL of deionized water and stirred at 70 °C for 10 min. A 25–28% ammonia solution was then added dropwise to the Zr(NO3)4 solution until the pH reached 10, and the mixture was stirred at 70 °C for 4 h. The CeO2 support was synthesized using a similar method, substituting Zr(NO3)4·6H2O with Ce(NO3)3·6H2O (99.99%, Sigma-Aldrich). The resultant solution was vacuum-filtered to yield the solid, which was thoroughly washed with deionized water until the pH stabilized at 7. The samples were then dried overnight at 105 °C, followed by calcination at 700 °C for 3 h with a ramp rate of 5 °C min−1.
Ni/CeO2 and Ni/ZrO2 catalysts were synthesized utilizing the wet impregnation method. Specifically, for the 5% Ni/CeO2 catalyst, 52 mg of Ni(NO3)3·6H2O (99.999%, Sigma-Aldrich) was dissolved in 100 mL of deionized water and stirred for 10 min. Subsequently, 2.00 g of CeO2 support was incorporated, and the suspension was stirred at 90 °C until complete evaporation of the water. The resulting solid was calcined in a muffle furnace at 700 °C for 3 h with a heating rate of 5 °C min−1. Following calcination, the catalysts were reduced under a hydrogen atmosphere at 500 °C for 2 h (5 °C min−1).
2.3. Catalyst characterization
The nickel content in the catalysts was analyzed using inductively coupled plasma spectrometry-atomic emission spectrometry (ICP-AES), employing the Agilent 725 instrument for precise measurement. Comprehensive XRD analysis was conducted on a Bruker AXS GmbH D8 Advance X-ray diffractometer, employing Cu Kα radiation across a broad range of angles from 10° to 90°, which facilitated detailed phase identification and crystal structure analysis. To evaluate the textural properties of the catalysts, N2 adsorption–desorption isotherms were meticulously recorded using a Micromeritics ASAP2012 analyzer. This precise measurement allowed for the accurate determination of specific surface area, average pore diameter, and total pore volume, providing valuable insights into the porosity of the materials. High-resolution SEM (Zeiss, Merlin) was employed for a meticulous investigation of surface morphology to reveal the detailed surface features of catalysts. Furthermore, HAADF-STEM-EDS on the JEOL JEM-2100F instrument was utilized to gain a deeper understanding of the catalysts' elemental distribution and structural characteristics at the nanoscale. The coke deposition on catalysts was studied by the combustion experiments in the air atmosphere from room temperature to 800 °C at a heating rate of 10 °C min−1 in a thermogravimetric analyzer (NETZSCH STA 409C/3/F).
2.4. CO2 gasification-reforming of municipal solid waste
Experimental studies on CO2 gasification-reforming of solid waste samples were conducted in a two-stage fixed-bed reactor connected to a micro-gas chromatograph (Micro-GC) as shown in Fig. 1. In a typical experiment, 1 g of sample was loaded in a crucible for the gasification stage, and 0.1 g of Ni/CeO2 or Ni/ZrO2 catalyst was fixed with quartz wool for the reforming stage. The CO2 flow rate was set at 50 mL min−1. Initially, the temperature in the reforming stage was gradually increased to a predefined target of 600, 700, or 800 °C at a heating rate of 10 °C min−1. This was followed by a steady heating of the gasification stage from room temperature to 600 °C, progressing at a consistent rate of 4 °C min−1, and kept at 600 °C for 30 min. The whole gasification process would last for 3 h. The liquid by-products were gathered by a condenser submerged in an icy water blend. Online gas composition analysis was efficiently conducted using the Micro-GC (Fusion 2-Module System, Inficon), where argon and helium were utilized as carrier gases for Module A (Rt-Molsieve 5A) and Module B (Rt-U-Bond), respectively. Micro-GC was programmed to automatically sample every 2 min, utilizing N2 at a flow rate of 10 mL min−1 as the internal standard for accurate quantification of gas production. Micro-GC extracted only 10 μL volume of gas per analysis. At the end of experiments, total gas production was quantified through analysis of the sample bags with Micro-GC.
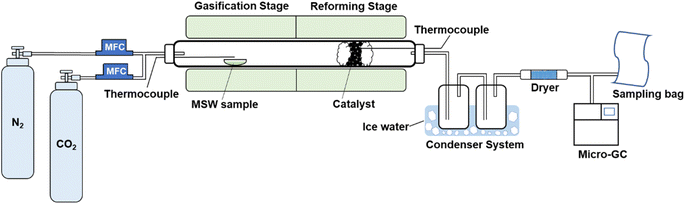 |
| Fig. 1 Schematic diagram of the fixed bed reactor. | |
3. Results and discussion
3.1. CO2 gasification-reforming of municipal solid waste
3.1.1. CO2 gasification-reforming without catalysts.
The CO2 gasification of various municipal solid waste samples, including cabbage, poplar leaves, printed paper, PET, and HDPE, was carried out in the two-stage fixed-bed reactor connected to a Micro-GC. The samples initially underwent gasification to produce volatiles, followed by a secondary reforming with CO2. The reforming segment of the reactor was preheated to 700 °C, after which the municipal solid waste samples were subjected to a gradual heating process, reaching 600 °C at a rate of 4 °C min−1. The online gas production rates of samples during CO2 gasification-reforming are illustrated in Fig. 2. The gas production rates for cabbage, poplar leaves, printed paper, PET, and HDPE peaked at approximately 270, 295, 360, 440, and 485 °C, respectively. Upon examining the initial temperatures for thermal decomposition, it was observed that the synthetic polymers PET and HDPE exhibited markedly superior thermal stability compared to the three other samples, aligning with results related to thermal stability in the pyrolysis process.34
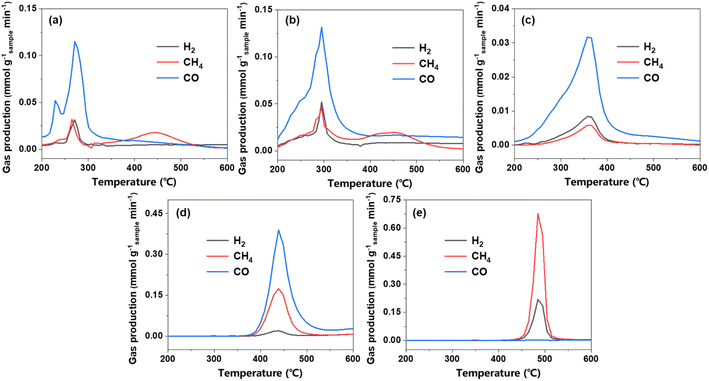 |
| Fig. 2 The gas production during CO2 gasification-reforming of municipal solid wastes without catalysts at a reforming temperature of 700 °C: (a) cabbage, (b) poplar leaves, (c) printed paper, (d) PET, and (e) HDPE. | |
Fig. 3 presents the gas yields for various samples throughout the experimental process. Notably, the CO yields from cabbage, poplar leaves, printed paper, and PET exceeded the yields of H2 and CH4. However, HDPE's CO yield was almost negligible, yet it exhibited the highest CH4 yield among all samples, attributed to the cleavage of chain hydrocarbons.35 In scenarios lacking a catalyst, the total gas yields of these samples were relatively low, following a descending order: PET (8.2 mmol gsample−1) > HDPE (6.5 mmol gsample−1) > poplar leaves (5.6 mmol gsample−1) > cabbage (3.5 mmol gsample−1) > printed paper (1.1 mmol gsample−1).
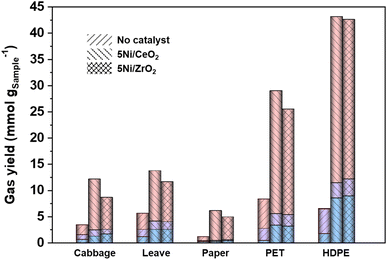 |
| Fig. 3 The gas yields of cabbage, poplar leaves, printed paper, PET, and HDPE during non-catalytic and catalytic CO2 gasification-reforming (blue: H2, purple: CH4, pink: CO). | |
3.1.2. CO2 gasification-reforming with Ni/CeO2 and Ni/ZrO2 catalysts.
Incorporating catalysts into the CO2 gasification-reforming process of municipal solid waste can significantly elevate gas generation, as delineated in Fig. 3. The deployment of 5% Ni/CeO2 catalysts resulted in a substantial increase in the total gas yields from cabbage, poplar leaves, printed paper, PET, and HDPE, augmenting yields by 246%, 145%, 455%, 254%, and 563%, respectively. Similarly, the use of 5% Ni/ZrO2 catalysts enhanced the total gas production for the same materials by 146%, 107%, 345%, 211%, and 555%, respectively. The efficacy of these nickel-based catalysts in boosting gas production was attributed to their role in promoting the cleavage of C–H and C–C bonds within the volatiles.36 Comparative analysis revealed that the 5% Ni/CeO2 catalysts demonstrated superior catalytic activity compared to 5% Ni/ZrO2 catalysts, a phenomenon likely influenced by the support's impact on the dispersion of nickel.37
In the CO2 gasification-reforming processes for various municipal solid waste components, catalysts exhibited distinct levels of activity. Typically, for HDPE, the CO yield in the absence of catalysts was negligible. However, the introduction of the catalyst led to a dramatic increase in CO production, reaching approximately 31 mmol gsample−1, a yield significantly higher than that of the other four samples. Proximate analysis revealed that HDPE's volatile matter content was 100 wt% (Table S1†), however, the gas yield without the catalysts was a mere 6.5 mmol gsample−1. This suggested a substantial presence of condensable components in the volatiles. Catalysts markedly enhanced the reforming efficiency of tar, leading to a significant increase in the gas production of HDPE.
The online gas production rates from municipal solid waste during CO2 gasification-reforming with 5% Ni/CeO2 and 5% Ni/ZrO2 catalysts are illustrated in Fig. S1–S5.† The peak temperatures aligned with those observed in the absence of catalysts, as the catalysts did not come into contact with the samples, thereby not influencing their decomposition. Nevertheless, the introduction of these catalysts significantly elevated the maximum values and peak areas of CO, H2, and CH4 production. Moreover, the composition of cabbage, poplar leaves, and printed paper was complex, including cellulose, lignin, and hemicellulose.38 Consequently, their gasification processes were intricate, manifesting multiple peaks in gas generation. In contrast, synthetic polymers such as HDPE and PET, synthesized from monomers, exhibited a simpler structure, thereby resulting in a singular, prominent peak in gas production.39
3.2. Optimization of Ni loading and reforming temperature for the CO2 gasification-reforming
Given its highest gas yields among the five samples, HDPE was chosen to investigate the ideal Ni loading for Ni/CeO2 catalysts and the optimal reforming temperature. First, the pivotal role of CO2 was underscored by a comparative experiments between N2 and CO2 atmospheres. Gas yields for HDPE in both atmospheres with and without the catalysts are depicted in Table S2.† The introduction of the 5% Ni/CeO2 catalyst in a N2 atmosphere had a minimal impact on gas production, suggesting negligible influence on the cracking of HDPE volatiles. Conversely, the catalyst's presence in a CO2 atmosphere markedly enhanced gas production. This boost was credited to the catalyst's facilitation of CO2 reforming of volatile components, leading to substantial syngas generation. The absence of a catalyst in both N2 and CO2 atmospheres showed no notable variation in gas yields, indicating the CO2 reforming of volatiles was difficult to occur in the absence of a catalyst. These findings underscore the significant role of CO2 and the high activity and effectiveness of the Ni/CeO2 catalyst in facilitating the gasification-reforming process of municipal solid waste.
Catalysts with different Ni loadings (2, 5 and 10 wt%) were assessed at CO2 flow rate of 50 mL min−1 and a reforming temperature of 700 °C. As shown in Table S3,† ICP-AES analysis verified the accuracy of Ni/CeO2 catalyst loadings. As the Ni loadings increased, there was a notable decline in the gas production from HDPE, a trend clearly illustrated in Fig. 4 a. The yields of H2, CH4 and CO decreased from 1.9, 2.7 and 7.4 mol gsample−1 gNi−1 with 2% Ni/CeO2 catalysts to 0.7, 0.3 and 2.4 mol gsample−1 gNi−1 with 10% Ni/CeO2 catalysts, respectively. It was reported that with a Ni loading of 2 wt%, the Ni species were predominantly atomic or nanocluster forms, with just 8% as nanoparticles.40 In contrast, Ni nanoparticles constituted 32% at 10 wt% Ni loading.40 Hence, it was surmised that the reaction's active sites predominantly comprised Ni atoms or nanoclusters and the aggregation of Ni led to diminished catalytic effectiveness.
 |
| Fig. 4 Effects of (a) Ni loadings (2, 5 and 10 wt%) and (b) reforming temperatures (600, 700, and 800 °C) on gas production during the CO2 gasification-reforming of HDPE. | |
The impact of varying reforming temperatures (600, 700, and 800 °C) on gas production from HDPE is clearly demonstrated in Fig. 4b. As the reforming temperature increased from 600 to 700 °C, a marked increase in both CO and total gas yields was observed, rising from 13.4 and 21.4 mmol gsample−1 to 31.7 and 43.1 mmol gsample−1, respectively. This phenomenon was ascribed to the endothermic properties inherent in tar's CO2 reforming.41 Necessary heat uptake for the reaction implied a propensity for improved gas production at higher temperatures. Nevertheless, a further rise in the reforming temperature to 800 °C yielded no significant enhancement in gas production, with only a minor increase in CO yield of 1.4 mmol gsample−1. At elevated temperatures, the accelerated tar cracking resulted in the char formation, thereby diminishing the gas output from the CO2 reforming of tar.42 When the temperature was raised from 600 to 800 °C, the H2 yield initially increased and then declined. The H2 production was not only associated with tar CO2 reforming but also closely linked to the reverse water-gas shift reaction (CO2 + H2 → CO + H2O), where an increase in reforming temperature shifted the reaction equilibrium to the right.43
3.3. Stability of the catalyst
The stability experiments were conducted under the reforming temperature of 700 °C and Ni loading of 5 wt%. The stability of 5% Ni/CeO2 catalyst was evaluated over five cycles of CO2 gasification-reforming of HDPE (Fig. 5). The CO yield decreased with the increase of the number of cycles, dropping by 13.8% after five cycles—from 31.7 to 27.3 mmol gsample−1. The H2 and CH4 yields decreased from 8.5 and 2.9 mmol gsample−1 to 6.5 and 1.2 mmol gsample−1, respectively. The catalyst's ability to retain a significant portion of its initial activity, even after five cycles, underscores its potential for long-term application in relevant industrial processes. The deactivation mechanism of catalysts will be discussed in the following session.
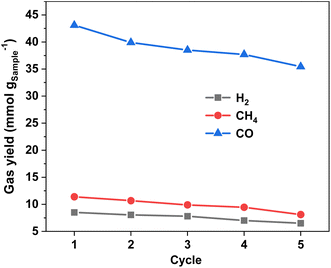 |
| Fig. 5 The stability of 5% Ni/CeO2 catalyst through five cycles of CO2 gasification-reforming of HDPE. | |
3.4. Characterization of fresh and used Ni/CeO2 and Ni/ZrO2 catalysts
Scanning electron microscopy (SEM) analysis of the CeO2 and ZrO2 supports revealed an irregular block-like structural morphology as shown in Fig. S6.† CeO2 and ZrO2 displayed similar specific surface areas, approximately 137 and 136 m2 g−1 respectively, as detailed in Table 1. However, the incorporation of 5 wt% Ni led to a significant decrease in the specific surface area, dropping to 52 m2 g−1 for 5% Ni/CeO2 and 53 m2 g−1 for 5% Ni/ZrO2. This decrease primarily stemmed from the clogging of support pores by Ni species or from sintering of the catalyst during calcination.44 Concurrently, both the average pore diameter and total pore volume experienced a decline. Specifically, 5% Ni/CeO2 exhibited an average pore diameter and total pore volume of 12.3 nm and 0.18 cm3 g−1, respectively, which were obviously lower than those of pure CeO2 support. Similarly, 5% Ni/ZrO2 showed a reduction in both average pore diameter and total pore volume compared to ZrO2 support. The N2 adsorption–desorption isotherms for all the materials revealed Type IV isotherms, featuring typical H1 type hysteresis loops as shown in Fig. S7,† highlighting their mesoporous characteristics.45
Table 1 Specific surface area and pore characteristics of the supports and catalysts
Material |
Specific surface area (m2 g−1) |
Average pore diameter (nm) |
Total pore volume (cm3 g−1) |
CeO2 |
137 |
22.1 |
0.77 |
ZrO2 |
136 |
21.2 |
0.76 |
5% Ni/CeO2 |
52 |
12.3 |
0.18 |
5% Ni/ZrO2 |
53 |
8.0 |
0.13 |
Prominent XRD diffraction peaks at 28.5, 33.1, 47.5, and 56.3° were identified for CeO2 and 5% Ni/CeO2, corresponding accurately to the cubic fluorite structure typical of CeO2, as shown in Fig. 6. In the case of ZrO2 and 5% Ni/ZrO2, the detection of both tetragonal and monoclinic phases was consistent with results reported in previous studies.46 The Ni diffraction peak, located around 44.6°, was evident in the 5% Ni/CeO2 and 5% Ni/ZrO2 catalysts. Significantly, the Ni diffraction peak in the 5% Ni/ZrO2 catalyst exhibited greater prominence and sharper definition relative to its counterpart in the 5% Ni/CeO2 catalyst. This observation implied a more efficient dispersion and a diminution in the size of Ni nanoparticles on the CeO2 support.46
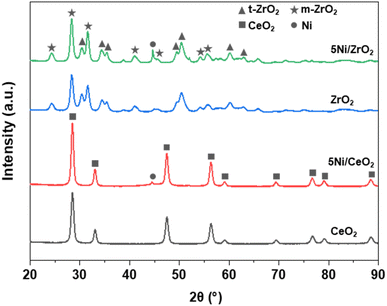 |
| Fig. 6 XRD patterns of CeO2, ZrO2, 5% Ni/CeO2, and 5% Ni/ZrO2 catalysts. | |
High-resolution transmission electron microscopy (HR-TEM) images of the 5% Ni/CeO2 and 5% Ni/ZrO2 revealed that the catalysts' structures comprised agglomerations of numerous particles, each measuring between 10–20 nm in diameter (Fig. S8†). Given that both cerium and zirconium possessed higher atomic numbers than nickel, Ni nanoparticles were not readily discernible on the CeO2 and ZrO2 using HR-TEM due to contrast limitations. To overcome this, high-angle annular dark-field scanning transmission electron microscopy (HAADF-STEM) coupled with energy-dispersive X-ray spectroscopy (EDS) was employed to examine Ni distribution on these supports. The HAADF-STEM-EDS analysis clearly showed a more uniform dispersion of Ni on the CeO2 support compared to ZrO2 as shown in Fig. 7. This enhanced dispersion correlated with the findings from XRD analysis and likely contributed to the superior catalytic activity observed in the 5% Ni/CeO2 catalyst during CO2 gasification-reforming of municipal solid wastes due to the increased surface area for reactions and better interaction between the Ni particles and the CeO2 surface.47
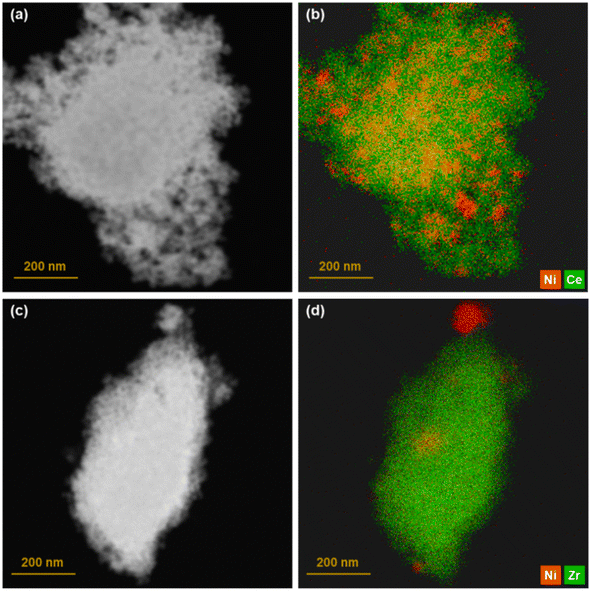 |
| Fig. 7 HAADF-STEM-EDS images of (a and b) 5% Ni/CeO2 and (c and d) 5% Ni/ZrO2 catalysts. | |
TGA and SEM analyses were performed on the used catalysts after 5 cycles to reveal the deactivation mechanism. TGA experiments were performed under air atmosphere to evaluate the type and amount of the deposited coke, as shown in Fig. S9a.† The two peaks of the DTG curve at about 400 and 600 °C correspond to the combustion of amorphous carbon and carbon nanotubes, respectively.48,49 Predominantly, the carbon deposits identified in this study were in the form of carbon nanotubes. The total carbon deposit was around 40 wt%. This observation was in line with findings from other research, which had highlighted the role of Ni-based catalysts in fostering the formation of carbon nanotubes during the pyrolysis and gasification of plastics.50,51 The morphology of the carbon nanotubes were further examined using SEM (Fig. S9b†).
3.5. CO2 gasification-reforming of simulated municipal solid wastes with Ni/CeO2 catalysts
The 5% Ni/CeO2 catalysts were employed for the CO2 gasification-reforming of simulated municipal solid waste. It was reported that municipal solid waste predominantly comprises approximately 62.6% food waste, 16.9% plastics, 12.8% paper, 4.7% textiles, and 3.0% leaves.33 The simulated municipal solid waste used in this study was composed of 62.6% cabbage, 16.9% HDPE, 12.8% printed paper, 4.7% PET, and 3.0% poplar leaves. As demonstrated in Table 2, in the absence of catalysts, the production of CO and total gas were 2.0 and 4.4 mmol gsample−1, respectively. The introduction of the 5% Ni/CeO2 catalyst led to a significant uptick in gas yields, with CO and total gas outputs rising by 223% and 106%, achieving 6.8 and 9.1 mmol gsample−1, respectively. A comparison between the experimental and theoretical gas yields calculated by the sum of individual components of the municipal solid waste highlighted a significant discrepancy. The theoretical predictions substantially outstripped the experimental results. This disparity underscored the complexity of the CO2 gasification-reforming process of actual municipal solid waste due to the intricate interactions among various components, and the gas yields cannot be accurately determined by summing the results of individual component gasification.52
Table 2 The experimental and theoretical gas yields of simulated municipal solid wastea
Condition |
Gas yield (mmol gsample−1) |
H2 |
CH4 |
CO |
Total gas |
Remark: reaction conditions: reforming temperature of 700 °C.
|
Without catalyst |
1.1 |
1.3 |
2.0 |
4.4 |
With 5% Ni/CeO2 catalyst |
Experimental values |
1.1 |
1.3 |
6.8 |
9.1 |
Theoretical values |
2.4 |
1.4 |
13.6 |
17.4 |
4. Conclusions
The CO2 gasification-reforming of municipal solid waste was investigated in a two-stage fixed bed reactor. In scenarios lacking a catalyst, the total gas yields of these samples were relatively low, following a descending order: PET > HDPE > poplar leaves > cabbage > printed paper. In addition, the synthetic polymers PET and HDPE exhibited markedly superior thermal stability compared to the three other samples. Incorporating 5% Ni/CeO2 and 5% Ni/ZrO2 catalysts into the CO2 gasification-reforming process of municipal solid waste significantly elevated gas generation, and the 5% Ni/CeO2 catalysts demonstrated superior catalytic activity. XRD and HAADF-STEM-EDS analysis clearly showed a more uniform dispersion of Ni on the CeO2 support compared to ZrO2, leading to enhanced activity of the 5% Ni/CeO2 catalysts. Comparative studies of catalysts with different Ni loadings (2, 5 and 10 wt%) demonstrated that Ni aggregation led to a decrease in catalytic activity. Furthermore, stability testing revealed that after five reaction cycles, the catalyst experienced a certain level of deactivation, primarily due to coke deposition. Using 5% Ni/CeO2 catalysts in CO2 gasification-reforming of simulated municipal solid waste significantly enhanced CO and total gas production, with increases of 223% and 106%, respectively.
Conflicts of interest
There are no conflicts to declare.
Acknowledgements
This work was supported by the National Natural Science Foundation of China (52276202), National Key R&D Program of China (2023YFC3905701), Huaneng Group Science and Technology Research Project (KTHT-U23GCZH01, KTHT-U22YYJC12), Tsinghua-Jiangyin Innovation Special Fund (TJISF), Tsinghua-Toyota Joint Research Fund, and State Key Laboratory of Chemical Engineering (SKL-ChE-22A01).
References
- W. Deng, Y. Feng, J. Fu, H. Guo, Y. Guo and B. Han,
et al., Catalytic conversion of lignocellulosic biomass into chemicals and fuels, Green Energy Environ., 2023, 8, 10–114 CrossRef CAS.
- Z. Wang, Z. Sun, H. Yin, H. Wei, Z. Peng and Y. X. Pang,
et al., The role of machine learning in carbon neutrality: Catalyst property prediction, design, and synthesis for carbon dioxide reduction, eScience, 2023, 3, 100136 CrossRef.
-
T. Bank, Trends in solid waste management, 2021, vol. 7 Search PubMed.
- M. H. Khoshgoftar Manesh, S. Davadgaran and S. A. Mousavi Rabeti, Gasification potential of municipal solid waste in Iran: Application of life cycle assessment, risk analysis, and machine learning, J. Clean. Prod., 2024, 434, 140177 CrossRef.
- M. Irfan, A. Li, L. Zhang, G. Ji, Y. Gao and S. Khushk, Hydrogen-rich syngas from wet municipal solid waste gasification using Ni/Waste marble powder catalyst promoted by transition metals, Waste Manage., 2021, 132, 96–104 CrossRef CAS PubMed.
- H. Zhou, A. Meng, Y. Long, Q. Li and Y. Zhang, An overview of characteristics of municipal solid waste fuel in China: Physical, chemical composition and heating value, Renew. Sustain. Energy Rev., 2014, 36, 107–122 CrossRef CAS.
-
X. Y. Ding, Y. J. Huang, X. X. Dong, J. Q. Zhao, M. Z. Yu, X. Q. Tian, et al., In-situ catalytic decomposition of emitted ammonia from municipal solid waste gasification by Ni-M bimetallic catalysts supported on sewage sludge-derived biochar, Waste Disposal & Sustainable Energy, 2023, vol. 5, pp. 113–124 Search PubMed.
- S. Zhang, S. Yu, Q. Li, B. A. Mohamed, Y. Zhang and H. Zhou, Insight into the relationship between CO2 gasification characteristics and char structure of biomass, Biomass Bioenergy, 2022, 163, 106537 CrossRef CAS.
- A. Molino, S. Chianese and D. Musmarra, Biomass gasification technology: The state of the art overview, J. Energy Chem., 2016, 25, 10–25 CrossRef.
- R. Mishra, E. Singh, A. Kumar, A. Ghosh, S. Lo and S. Kumar, Co-gasification of solid waste and its impact on final product yields, J. Clean. Prod., 2022, 374, 133989 CrossRef CAS.
- S. Zhang, M. Wu, Z. Qian, Q. Li, Y. Zhang and H. Zhou, CO rich syngas production from catalytic CO2 gasification-reforming of biomass components on Ni/CeO2, Fuel, 2024, 357, 130087 CrossRef CAS.
- G. Liu, S. Sun, H. Sun, Y. Zhang, J. Lv and Y. Wang,
et al., Integrated CO2 capture and utilisation: A promising step contributing to carbon neutrality, Carbon Capture Sci. Technol., 2023, 7, 100116 CrossRef CAS.
- M. Wang and J. Luo, A coupled electrochemical system for CO2 capture, conversion and product purification, eScience, 2023, 3, 100155 CrossRef.
- B. Wang, R. Gupta, L. Bei, Q. Wan and L. Sun, A review on gasification of municipal solid waste (MSW): Syngas production, tar formation, mineral transformation and industrial challenges, Int. J. Hydrogen Energy, 2023, 48, 26676–26706 CrossRef CAS.
- M. Irfan, A. Li, L. Zhang, G. Ji, Y. Gao and S. Khushk, Hydrogen-rich syngas from wet municipal solid waste gasification using Ni/Waste marble powder catalyst promoted by transition metals, Waste Manage., 2021, 132, 96–104 CrossRef CAS PubMed.
-
A. S. Al-Rahbi and P. T. Williams, Decomposition of biomass gasification tar model compounds over waste tire pyrolysis char, Waste Disposal & Sustainable Energy, 2022, vol. 4, pp. 75–89 Search PubMed.
- F. L. Chan and A. Tanksale, Review of recent developments in Ni-based catalysts for biomass gasification, Renew. Sustain. Energy Rev., 2014, 38, 428–438 CrossRef CAS.
- A. Galadima, A. Masudi and O. Muraza, Catalyst development for
tar reduction in biomass gasification: Recent progress and the way forward, J. Environ. Manage., 2022, 305, 114274 CrossRef CAS PubMed.
- A. M. Ruppert, M. Niewiadomski, J. Grams and W. Kwapiński, Optimization of Ni/ZrO2 catalytic performance in thermochemical cellulose conversion for enhanced hydrogen production, Appl. Catal. B: Environ., 2014, 145, 85–90 CrossRef CAS.
- Z. Zhang, L. Liu, B. Shen and C. Wu, Preparation, modification and development of Ni-based catalysts for catalytic reforming of tar produced from biomass gasification, Renew. Sustain. Energy Rev., 2018, 94, 1086–1109 CrossRef CAS.
- H. Guo, D. Si, H. Zhu, Q. Li, Y. Huang and R. Cao, Ni single-atom sites supported on carbon aerogel for highly efficient electroreduction of carbon dioxide with industrial current densities, eScience, 2022, 2, 295–303 CrossRef.
- N. Srisiriwat, S. Therdthianwong and A. Therdthianwong, Oxidative steam reforming of ethanol over Ni/Al2O3 catalysts promoted by CeO2, ZrO2 and CeO2–ZrO2, Int. J. Hydrogen Energy, 2009, 34, 2224–2234 CrossRef CAS.
- S. O. Omarov, D. A. Sladkovskiy, K. D. Martinson, M. Peurla, A. Aho and D. Y. Murzin,
et al., Influence of the initial state of ZrO2 on genesis, activity and stability of Ni/ZrO2 catalysts for steam reforming of glycerol, Appl. Catal., A, 2021, 616, 118098 CrossRef CAS.
- S. Ratchahat, S. Kodama, W. Tanthapanichakoon and H. Sekiguchi, CO2 gasification of biomass wastes enhanced by Ni/Al2O3 catalyst in molten eutectic carbonate salt, Int. J. Hydrogen Energy, 2015, 40, 11809–11822 CrossRef CAS.
- J. Yang, X. Lu, C. Han, H. Liu, D. Gong and L. Mo,
et al., Glycine-assisted preparation of highly dispersed Ni/SiO2 catalyst for low-temperature dry reforming of methane, Int. J. Hydrogen Energy, 2022, 47, 32071–32080 CrossRef CAS.
- J. Ren, F. Zeng, C. Mebrahtu, Z. D. Wang and R. Palkovits, Promotional effects of Ru and Fe on Ni/ZrO2 catalyst during CO2 methanation: A comparative evaluation of the mechanism, J. Energy Chem., 2023, 86, 351–361 CrossRef CAS.
- W. Zheng, K. Sun, H. Liu, Y. Liang and B. Xu, Nanocomposite Ni/ZrO2: Highly active and stable catalyst for H2 production via cyclic stepwise methane reforming reactions, Int. J. Hydrogen Energy, 2012, 37, 11735–11747 CrossRef CAS.
- D. Eder and R. Kramer, The stoichiometry of hydrogen reduced zirconia and its influence on catalytic activity - Part 1: Volumetric and conductivity studies, Phys. Chem. Chem. Phys., 2002, 4, 795–801 RSC.
- Y. Wang, L. Yao, Y. Wang, S. Wang, Q. Zhao and D. Mao,
et al., Low-Temperature Catalytic CO2 Dry Reforming of Methane on Ni-Si/ZrO2 Catalyst, ACS Catal., 2018, 8, 6495–6506 CrossRef CAS.
- H. Zhou, H. Wang, A. D. Sadow and I. I. Slowing, Toward hydrogen economy: Selective guaiacol hydrogenolysis under ambient hydrogen pressure, Appl. Catal. B: Environ., 2020, 270, 118890 CrossRef CAS.
- Y. Tang, Y. Wei, Z. Wang, S. Zhang, Y. Li and L. Nguyen,
et al., Synergy of Single-Atom Ni1 and Ru1 Sites on CeO2 for Dry Reforming of CH4, J. Am. Chem. Soc., 2019, 141, 7283–7293 CrossRef CAS PubMed.
- Z. Liu, D. C. Grinter, P. G. Lustemberg, T. Nguyen-Phan, Y. Zhou and S. Luo,
et al., Dry Reforming of Methane on a Highly-Active Ni-CeO2 Catalyst: Effects of Metal-Support Interactions on C–H Bond Breaking, Angew. Chem., Int. Ed., 2016, 55, 7455–7459 CrossRef CAS PubMed.
- F. Tang, Z. Yu, Y. Li, L. Chen and X. Ma, Catalytic co-pyrolysis behaviors, product characteristics and kinetics of rural solid waste and chlorella vulgaris, Bioresour. Technol., 2020, 299, 122636 CrossRef CAS PubMed.
- X. Zhang, H. Lei, L. Zhu, X. Zhu, M. Qian and G. Yadavalli,
et al., Thermal behavior and kinetic study for catalytic co-pyrolysis of biomass with plastics, Bioresour. Technol., 2016, 220, 233–238 CrossRef CAS PubMed.
- E. Parparita, M. A. Uddin, T. Watanabe, Y. Kato, J. Yanik and C. Vasile, Gas production by steam gasification of polypropylene/biomass waste composites in a dual-bed reactor, J. Mater. Cycles Waste Manage., 2015, 17, 756–768 CrossRef CAS.
- C. Wu, Z. Wang, J. Huang and P. T. Williams, Pyrolysis/gasification of cellulose, hemicellulose and lignin for hydrogen production in the presence of various nickel-based catalysts, Fuel, 2013, 106, 697–706 CrossRef CAS.
- K. Wang, B. Dou, B. Jiang, Q. Zhang, M. Li and H. Chen,
et al., Effect of support on hydrogen production from chemical looping steam reforming of ethanol over Ni-based oxygen carriers, Int. J. Hydrogen Energy, 2016, 41, 17334–17347 CrossRef CAS.
- H. Zhou, A. Meng, Y. Long, Q. Li and Y. Zhang, An overview of characteristics of municipal solid waste fuel in China: Physical, chemical composition and heating value, Renew. Sustain. Energy Rev., 2014, 36, 107–122 CrossRef CAS.
- R. K. Singh, B. Ruj, A. K. Sadhukhan and P. Gupta, A TG-FTIR investigation on the co-pyrolysis of the waste HDPE, PP, PS and PET under high heating conditions, J. Energy Inst., 2020, 93, 1020–1035 CrossRef CAS.
- M. Akri, S. Zhao, X. Y. Li, K. T. Zang, A. F. Lee and M. A. Isaacs,
et al., Atomically dispersed nickel as coke-resistant active sites for methane dry reforming, Nat. Commun., 2019, 10, 5181 CrossRef PubMed.
- L. Devi, K. J. Ptasinski and F. J. Janssen, A review of the primary measures for tar elimination in biomass gasification processes, Biomass Bioenergy, 2003, 24, 125–140 CrossRef CAS.
- M. Zhai, X. Wang, Y. Zhang, P. Dong, G. Qi and Y. Huang, Characteristics of rice husk tar secondary thermal cracking, Energy, 2015, 93, 1321–1327 CrossRef CAS.
- J. M. Saad and P. T. Williams, Pyrolysis-catalytic dry (CO2) reforming of waste plastics for syngas production: Influence of process parameters, Fuel, 2017, 193, 7–14 CrossRef CAS.
- H. Zhou, J. M. Saad, Q. Li and Y. Xu, Steam reforming of polystyrene at a low temperature for high H2/CO gas with bimetallic Ni-Fe/ZrO2 catalyst, Waste Manage., 2020, 104, 42–50 CrossRef CAS PubMed.
- M. Aziz, A. A. Jalil, S. Triwahyono, R. R. Mukti, Y. H. Taufiq-Yap and M. R. Sazegar, Highly active Ni-promoted mesostructured silica nanoparticles for CO2 methanation, Appl. Catal. B: Environ., 2014, 147, 359–368 CrossRef CAS.
- W. Wang, Z. Qu, L. Song and Q. Fu, CO2 hydrogenation to methanol over Cu/CeO2 and Cu/ZrO2 catalysts: Tuning methanol selectivity via metal-support interaction, J. Energy Chem., 2020, 40, 22–30 CrossRef.
- T. A. Le, M. S. Kim, S. H. Lee, T. W. Kim and E. D. Park, CO and CO2 methanation over supported Ni catalysts, Catal. Today, 2017, 293–294, 89–96 CrossRef CAS.
- Y. Zhu, J. Miao, M. Long and C. Wu, Feasibilities of producing high-value carbon nanotubes from waste plastics by spray pyrolysis, J. Anal. Appl. Pyrolysis, 2022, 166, 105613 CrossRef CAS.
- S. Zhang, X. Bie, Z. Qian, M. Wu, K. Li and Q. Li,
et al., Synergistic interactions between cellulose and plastics (PET, HDPE, and PS) during CO2 gasification-catalytic reforming on Ni/CeO2 nanorod catalyst, Appl. Energy, 2024, 361, 122975 CrossRef CAS.
- R. Yang, K. Chuang and M. Wey, Effects of Nickel Species on Ni/Al2O3 Catalysts in Carbon Nanotube and Hydrogen Production by Waste Plastic Gasification: Bench- and Pilot-Scale Tests, Energy Fuels, 2015, 29, 8178–8187 CrossRef CAS.
- Q. Liu, N. Cai, B. Peng, C. Wu, R. Hong and D. Jiang,
et al., Investigating the influences of metal-support interaction in NiFe catalysts on the quality of carbon nanomaterials from waste polypropylene, Fuel Process. Technol., 2022, 236, 107428 CrossRef CAS.
-
H. Zhou, Combustible Solid Waste Thermochemical Conversion: a Study of Interactions and Influence Factors, Springer, 2017 Search PubMed.
|
This journal is © The Royal Society of Chemistry 2024 |