A fluorescent hydrogen-bonded organic framework for highly selective sensing of mono-nitrophenol isomers in water†
Received
2nd September 2023
, Accepted 20th November 2023
First published on 20th November 2023
Abstract
Hydrogen-bonded organic frameworks (HOFs) have great potential as sensing platforms for specific molecular recognition due to their designable structures, high solution processability, and easy regeneration. However, the exploration of fluorescent HOFs for chemical sensing in water remains a challenge due to the need to combine excellent photoluminescence performance, high water stability, and specific recognition sites simultaneously. Herein, we report an aggregation-induced emission (AIE) active HOF (termed HOF-TPE-CN) built from a cyano-decorated tetraphenylethylene linker for highly fluorescent sensing of mono-nitrophenol isomers. Owing to the effective AIE effect, HOF-TPE-CN shows a notable enhancement in fluorescence emission, wherein the quantum yield of HOF-TPE-CN was notably increased to 21% compared with that of the TPE-CN ligand in DMF solution (2.89%). Most importantly, this HOF exhibits highly efficient fluorescence quenching toward mono-nitrophenol isomers in water, with a remarkably low detection limit of 0.65 μM. Furthermore, HOF-TPE-CN is more responsive and selective toward mono-nitrophenols compared with a series of homologues. Theoretical calculations combined with X-ray photoelectron spectroscopy revealed that there exist multiple van der Waals and hydrogen-bonding interactions between the trapped nitrophenol molecules and the HOF framework, which form ground-state complexation, resulting in fluorescence quenching by efficient photo-induced electron transfer.
Introduction
Nitrophenols, usually utilized as preservatives and insecticides, can accumulate in organisms through water to cause neurological problems and cancer.1,2 Mono-nitrophenols are representative members of nitrophenols that exist in three isomeric forms, ortho-nitrophenol (ONP), meta-nitrophenol (MNP), and para-nitrophenol (PNP), which can coexist in industrial wastewater due to their water solubility.3 Among them, ONP and PNP are listed as priority pollutants by the US Environmental Protection Agency. At present, fluorescence has become the dominant sensing technology for the detection of mono-nitrophenol owing to its quick response, high sensitivity, and strong practicability.4 Molecular recognition plays a vital role in fluorescence sensing, typically through specific interactions such as hydrogen bonding (H-bonding), metal coordination, and van der Waals interactions between the guest and host molecules.5 By means of their specific molecular recognition, porous materials such as metal–organic frameworks (MOFs)6 and covalent organic frameworks (COFs)7 have received tremendous attention as promising chemical sensors due to their highly designable pore spaces and functionalities for specific and selective recognition.8
Compared with MOFs and COFs, hydrogen-bonded organic frameworks (HOFs), as an emerging type of porous crystalline material that are self-assembled by discrete organic building blocks through H-bonding interactions, have significantly lagged behind in chemical sensing.9 This is because the establishment of permanent porosity and framework stability in HOFs is a long-standing challenge due to the fragile nature of hydrogen bonds.10 In spite of this, the intrinsic characteristics of H-bonds (weak, reversible, and flexible) endow HOF materials with many unique features.11 For example, HOFs possess some inherent merits including high solution processability, easy purification, and facile regeneration and healing by simple recrystallization.12 In addition, HOF materials can be highly crystalline which benefits structural determination and the investigation of structure–property relationships.13 These attractive advantages make HOFs promising candidates for chemical sensing applications.14
Despite these attractive merits, the design of HOF-based fluorescent probes for chemical sensing in water is still at an early stage and faces several challenges.9c,d First, fluorescence intensity changes are the most easily detectable response to chemical sensing. This requires fluorescent materials to have bright fluorescence intensity and high quantum yields. However, most of the reported fluorescent HOFs commonly show poor fluorescence intensity with low quantum yields because of the aggregation-caused quenching effect, which results from π–π stacking within the crystalline frameworks.15 Second, HOFs typically lack strong binding sites as specific recognition sites for target analytes, resulting in poor sensing sensitivity and selectivity. Last but not least, most sensing applications such as nitrophenol sensing should be executed in aqueous solutions, which requires chemical sensors to have high and long-term water stability. To date, only a limited number of HOFs have shown good stability in water.16 Evidently, the design of fluorescent and water-stable HOF-based sensors for highly selective and sensitive sensing of mono-nitrophenols in water is still very challenging.
To improve the fluorescence intensity, one of the most popular strategies is to select AIE-active organic linkers as building units to construct highly fluorescent HOFs. This is because rigidifying AIE-type linkers by solid framework formation would efficiently restrict intramolecular motions and thus raise the quantum yields, as exemplified well by AIE-based MOFs17 and COFs.18 In this regard, tetraphenylethylene (TPE) is well-known for its AIE character, which can serve as a very promising candidate for designing highly fluorescent HOF materials.11d,19 Furthermore, π-electron-rich TPE groups were demonstrated to readily interact with electron-deficient molecules such as mono-nitrophenols through π–π and H-bonding interactions, which can induce a change in luminescence intensity.1e,20 Recent studies have shown that the use of cyano groups as supramolecular synthons can construct water-stable HOFs by multiple CN⋯H–C hydrogen bonds.11a,21 In addition, cyano groups can also serve as specific binding sites to interact with mono-nitrophenol molecules through H-bonding interactions.
With the above considerations in mind, we reasoned that the use of the AIE-type TPE units as tectons and the cyano groups as supramolecular synthons has great potential to realize highly fluorescent and water-stable HOFs for chemical sensing of mono-nitrophenols. Herein, we designed and synthesized a highly fluorescent and stable HOF material (termed HOF-TPE-CN) by using a cyano-based TPE linker (4,4′,4′′,4′′′-(ethene-1,1,2,2-tetrayl) tetrabenzonitrile, TPE-CN). The abundant C–H⋯N and C–H⋯π interactions between the neighbouring TPE-CN linkers enable the framework of HOF-TPE-CN to be highly stable in water and different pH solutions (Fig. 1). Due to the effective AIE effect, the immobilization of functionalized TPE units into rigid HOF-TPE-CN turns on the fluorescence emission, whose quantum yield (21%) is 7.3 times higher than that of the TPE-CN ligand in DMF solution. Most importantly, the high fluorescence intensity of HOF-TPE-CN was found to be largely quenched by exposure of the sample to various nitrophenols in aqueous solutions, wherein the quenching efficiencies are 89.5%, 78%, and 98.7% for ONP, MNP and PNP, respectively. This water-stable fluorescent HOF-TPE-CN thus exhibits excellent selectivity and sensitivity to mono-nitrophenols, wherein the limit of detection of PNP reaches as low as 0.65 μM. It has also been revealed that the analyte molecules can enter the pores of HOF-TPE-CN to form ground-state charge transfer complexes with the framework through multiple intermolecular interactions, as revealed by theoretical calculations combined with X-ray photoelectron spectroscopy. This leads to efficient fluorescence quenching on HOF-TPE-CN because of the possible photo-induced electron transfer (PET) mechanism, as supported by density functional theory (DFT) calculations.
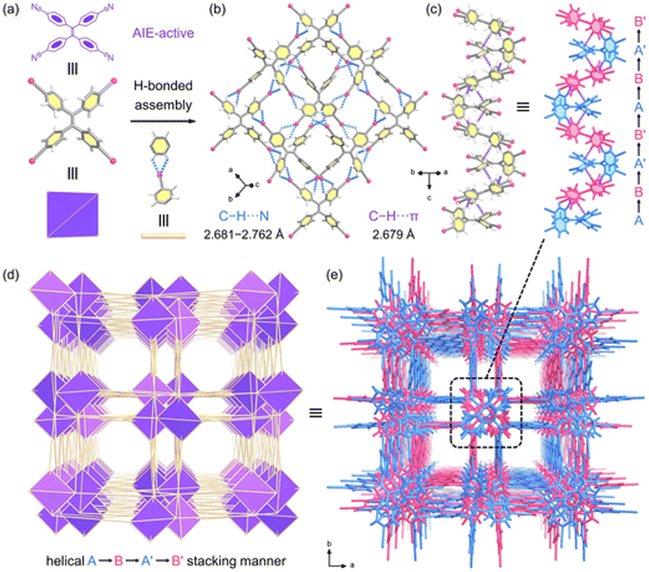 |
| Fig. 1 Crystal structure of HOF-TPE-CN. (a) Construction of sql-type topology networks with the four-connected TPE-CN ligand by forming four pairs of C–H⋯N hydrogen bonds with adjacent molecules. (b) The 2D layer is extended by intermolecular C–N⋯H hydrogen bonds (dotted line) between the adjacent building blocks. (c) C–H⋯π helical strand along the c axis where interlayer adjacent molecules stack in an ABA′B′ pattern. (d) The packing arrangement of TPE-CN generates 1D channels along the c axis with the simulation of a pore size of 4.6 Å. (e) Representation of the porous framework of HOF-TPE-CN. | |
Results and discussion
Structure analysis and stability characterization
The single crystals of HOF-TPE-CN can be obtained by slow vapor diffusion of n-hexane into the acetone solution of the TPE-CN ligand at room temperature for one week (Fig. S1†). During the preparation of this work, the crystal structure of this as-synthesized HOF was independently reported for white-light emission by Tang and Wei's group.22 Single-crystal X-ray crystallographic analysis revealed that HOF-TPE-CN crystallizes in the tetragonal space group I41/acd (Fig. S2†). As shown in Fig. 1a and b, each TPE-CN linker exists in a distorted propeller conformation and connects with four neighbouring linkers via eight pairs of strong intermolecular C–H⋯N hydrogen bonds (N⋯H 2.681–2.762 Å, and the sum of van der Waals (vdW) radii of N and H is 2.75 Å). Each cyano-group forms a pair of C–H⋯N hydrogen bonds with two hydrogen atoms from adjacent benzene rings, which are extended into sql net (Fig. 1c and d). Upon closer inspection of the structure, it can be observed that two neighbouring layers of molecules expand into a three-dimensional structure through multiple C–H⋯π interactions between adjacent benzene rings with a distance of 2.679 Å (Fig. 1c), which is shorter than the sum of vdW radii of C and H (2.90 Å). These short distances indicate relatively strong interactions between two layers, resulting in the robust structure of this HOF. Moreover, there are also weak and additional peripheral C–H⋯π interactions outside the aromatic rings (H⋯π 3.274–3.481 Å, Fig. S3†), which also play a role in the stabilization of the framework. As depicted in Fig. 1c, the interlayer adjacent layers stack in an ABA′B′ pattern and further assemble into 1D left-handed helical chains along the c axis. Thus, the channels have a square-shaped cross-section with a dimension of 4.6 Å after subtracting the van der Waals radius (Fig. 1e). The detailed structural information of HOF-TPE-CN is given in Table S1.†
The phase purity of HOF-TPE-CN was verified by the good agreement between the experimental powder X-ray diffraction (PXRD) pattern and the simulated one from the single-crystal structure (Fig. 2a). Thermogravimetric analysis (TGA, Fig. S4†) showed a steep weight loss below 413 K corresponding to the evaporation of solvent guests. Since mono-nitrophenols mainly exist in aquatic systems, their fluorescence sensing is typically performed in water solution. This requires HOF probes to have high chemical stability for practical applications. Our foregoing structural studies clearly showed that HOF-TPE-CN has multiple H-bonding and C–H⋯π interactions to stabilize the whole framework, which might result in high water stability. As confirmed by PXRD patterns and optical microscopy images, HOF-TPE-CN can retain its crystallinity and structural integrity after soaking in water for 5 days and HCl (pH = 1) and NaOH (pH = 10 and 14) solutions for 1 day (Fig. 2a, b, S5 and S6†). As shown in Fig. S7,† the fluorescence intensity virtually remains almost unchanged under these conditions, which supports their high resistance to water with different pH values. This water-stable feature of HOF-TPE-CN enables us to assess its fluorescence sensing properties toward mono-nitrophenols in water.
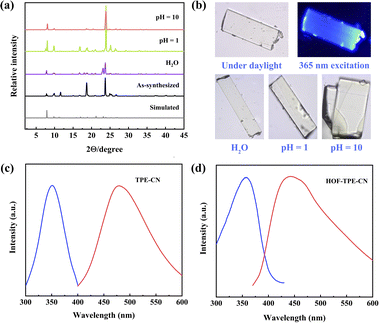 |
| Fig. 2 (a) PXRD patterns of as-synthesized HOF-TPE-CN and after being immersed in different solvents. (b) The optical microscopy image of a single HOF-TPE-CN crystal (top) under a bright field (left) and 365 nm excitation (right). The bright field images of crystals after soaking in water under different pH conditions (bottom). Fluorescence excitation spectra (blue) and emission spectra (red) of TPE-CN (c) and HOF-TPE-CN (d). | |
Fluorescence characteristics of HOF-TPE-CN
Subsequently, we tested the fluorescence behaviours of the TPE-CN ligand (Fig. S8†) and a single crystal of HOF-TPE-CN. According to the optical images, the single HOF-TPE-CN crystal emits blue light upon excitation at 365 nm (Fig. 2b and S9†). The optimum emission wavelength of the solid-state ligand is 478 nm under an excitation of 351 nm, while that of HOF-TPE-CN is located at 447 nm under an excitation of 357 nm (Fig. 2c and d). Compared to the emission spectrum of the TPE-CN ligand, a significant hyperchromic shift of 31 nm occurs after assembling into the HOF crystals. This change in the emission wavelength is mainly related to the confinement effect of the benzene rings by solid framework formation, as well exemplified by some AIE-based MOFs and COFs.23 Most importantly, such a confinement effect of benzene rings in HOF-TPE-CN also results in a significant enhancement in fluorescence emission, wherein the quantum yield of HOF-TPE-CN was notably increased to 21% compared with that of the TPE-CN ligand in DMF solution (2.89%). Such quantum yield of HOF-TPE-CN is higher than or comparable to that of some representative TPE-based HOFs, such as 2D-90 (10.61%),11d CBPE-1a (21%),24a and TP2VPE (16.44%).24b This observed 7.3 times enhancement in the emission of HOF-TPE-CN can be attributed to the typical characteristics of the AIE effect (Fig. S10†), which would be beneficial to improve the selectivity and sensitivity of chemical sensing of mono-nitrophenols.
Fluorescence sensing of nitrophenols
The structural analysis revealed that the framework of HOF-TPE-CN possesses a pore size comparable with that of mono-nitrophenol molecules (Fig. S11†), which enables the guest molecules to enter the pores. On the other hand, the electron-rich features of cyano groups around the pore channels may serve as specific binding sites to interact with electron-deficient mono-nitrophenol molecules through H-bonding interactions, thus resulting in the selective sensing of mono-nitrophenol. As shown in Fig. 3, the fluorescence intensity of the HOF-TPE-CN suspension was quenched gradually with the sequential addition of mono-nitrophenol solution. A linear response between the fluorescence intensity of HOF-TPE-CN at 425 nm and the concentrations of nitrophenols fits well with R2 = 0.99 at low concentrations in the range of 0–50 μM for ONP, 0–100 μM for MNP, and 0–100 μM for PNP, respectively. The fluorescence quenching efficiency can be quantitatively explained using the Stern–Volmer equation. From the insets of Fig. 3, it was found that HOF-TPE-CN has the highest quenching constant (Ksv) values of 1.34 × 104 M−1 toward PNP and 7 × 103 M−1, 3.5 × 103 M−1 toward ONP and MNP, respectively. These Ksv values are comparable to those of many promising sensing materials reported (Table S2†), such as BUT-12 (4.2 × 104)4 and Pythz-COF (4.4 × 104).26 Based on the Ksv values and the standard deviations, the limit of detection (LOD) of HOF-TPE-CN toward ONP, MNP, and PNP were calculated to be 1.1 μM, 2.39 μM, and 0.65 μM, respectively (see the details in the ESI†), which are lower than most of the promising crystalline sensing materials, including Th-TCBPE (0.7 μM),25 Pyaph-COF (42 μM),26 and CHOF-1 (8.78 μM).27 It is worthy of note that most of the related sensing reports were performed in organic solvents (Table S2†). These results indicate the remarkably high sensitivity of HOF-TPE-CN.
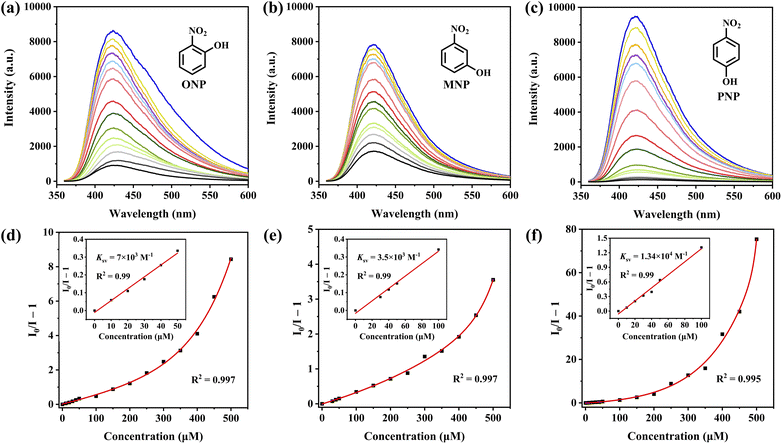 |
| Fig. 3 Fluorescence spectra of HOF-TPE-CN dispersed in water (1 mg mL−1) with increasing concentrations (0–500 μM) of (a) ONP; (b) MNP; (c) PNP. The exponentially fitted Stern–Volmer plots of (I0/I − 1) versus the concentrations of (d) ONP; (e) MNP; and (f) PNP. Inset: the linear fitting S–V plots at low concentrations of ONP, MNP, and PNP, respectively. | |
As shown in Fig. S13,† HOF-TPE-CN maintains its original crystallinity upon exposure to mono-nitrophenols in water as confirmed by PXRD. The selective detection of mono-nitrophenols in water is highly desirable for practical applications. Therefore, the selectivity of the fluorescence probe platforms was investigated regarding other soluble nitro-aromatics in water. As shown in Fig. 4, when the HOF sensor was exposed to 500 μM of other similar nitroaromatics (e.g., DNCB, DNBA, DNT, NT, NCB, DNB, NBDB, and DNP, Fig. S14 and S15†), this HOF material only showed a slight fluorescence quenching, which was no more than 22%. In contrast, the addition of ONP, MNP, and PNP produces an obvious quenching phenomenon, with a maximum quenching efficiency of up to 98.7%. These results reveal the high quenching selectivity of HOF-TPE-CN toward mono-nitrophenols. On the other hand, rapid response is also one of the essential properties of fluorescence detection platforms. When a certain number of analytes (ONP, MNP, and PNP) were added to the system, the fluorescence emission was quenched rapidly and reached a steady state within 1 min, and the fluorescence intensity remained unchanged within 5 min (Fig. S16†). Therefore, these results demonstrate the potential of using HOF-TPE-CN for the detection of mono-nitrophenol in practical water samples with high sensitivity and selectivity.
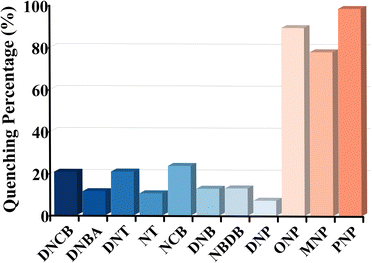 |
| Fig. 4 The quenching percentage of HOF-TPE-CN (1 mg mL−1) toward different nitroaromatic analytes at a concentration of 500 μM. | |
The excellent selectivity and sensitivity of HOF-TPE-CN toward mono-nitrophenols prompted us to examine its sensing ability in real water samples. As shown in Fig. S12,† a similar turn-off phenomenon and quenching efficiency were observed even in real water samples. To further evaluate the repeatability of HOF-TPE-CN for sensing mono-nitrophenols, we alternately immersed HOF-TPE-CN into 0 and 50 μM nitrophenol solutions and recorded their emission spectra. As depicted in Fig. S17,† the fluorescence of HOF-TPE-CN nearly recovered to its initial intensity upon removal of residual nitrophenols from the solution, and the fluorescence quenching remained almost consistent in four cycles. Therefore, HOF-TPE-CN exhibits a good repeatability for the chemical sensing of mono-nitrophenols.
Sensing mechanism
We have shown that HOF-TPE-CN exhibits superior sensing performance toward mono-nitrophenols in water, which might be attributed to some specific interactions between nitrophenols and the framework. To confirm this assumption, we first performed configurational-bias Monte Carlo (CBMC) simulations on HOF-TPE-CN to elucidate the interactions between the nitrophenol molecules and the framework (see the ESI† for the simulation details).28 Considering that the quenching effect of PNP is the most obvious, we simulated the interactions between PNP and HOF-TPE-CN in Materials Studio. As depicted in Fig. 5a–c and S18,† the PNP molecule diagonally occupies the pores of HOF-TPE-CN, which forms multiple supramolecular interactions with adjacent TPE-CN linkers. The H atom of the hydroxyl group can form an H-bonding interaction with the N atom of the cyano-group (3.4 Å). The phenyl ring of the PNP molecule interacts concurrently with the phenyl rings of two adjacent TPE linkers through a π–π (4.1 Å) and a C–H⋯π (3.8 Å) interaction, respectively. As a result, it provides a wide range of analyte–sensor interactions and facilitates efficient fluorescence quenching.
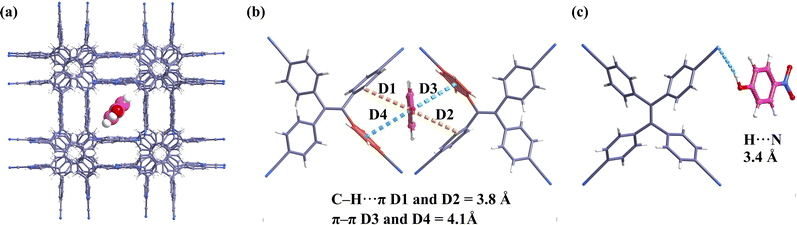 |
| Fig. 5 CBMC molecular simulation: (a) PNP within the HOF channel at 1 bar. (b and c) The binding site of PNP within the framework. The close C–H⋯π and π–π contacts and C–H⋯N hydrogen bonding interaction with the cyano-group in HOF-TPE-CN. | |
Next, X-ray photoelectron spectroscopy (XPS) tests were carried out to further evaluate the interactions between the analytes and the substrate at the experimental level. We analysed the changes in the elemental composition and chemical binding state of the system before and after interacting with nitrophenols and the obtained results are shown in Fig. 6. In the detailed spectra of carbon C 1s of HOF-TPE-CN, the C 1s line is distributed into 4 singlet spectral lines (Fig. 6a). The two most intense signals at binding energies of 282.94 eV and 284.42 eV can be attributed to the C–C bonds of benzene rings and the C
N bonds of cyano-group,29 respectively. The nitrogen N 1s lines are mainly formed by a strong main peak at a binding energy of 397.20 eV, which corresponds to the C
N group.29 After the addition of PNP, HOF-TPE-CN@PNP shows two most intense C 1s peaks at 283.6 eV and 284.99 eV (Fig. 6b), both of which have a positive shift compared with HOF-TPE-CN. Similarly, the single N 1s spectral line of C
N was also found to show a positive shift. All these shifts on C 1s and N 1s peaks for HOF-TPE-CN@PNP suggest that there indeed exist some interactions between the cyano-/phenyl-groups and PNP molecule.30 This is well consistent with the results of the above CBMC simulations. Furthermore, we note that the N 1s singlet spectral lines display a new peak at a binding energy of 398.77 eV (Fig. 6b), which is derived from the –NO2 group of the PNP molecule according to the previous literature.31 This also indicates that the PNP molecule can stay inside the pores due to the host–guest interactions. Therefore, all these XPS results combined with CBMC simulations reveal that the cyano-group and TPE units can provide specific binding sites for the PNP molecule, thus leading to the responsive and selective sensing of PNP.
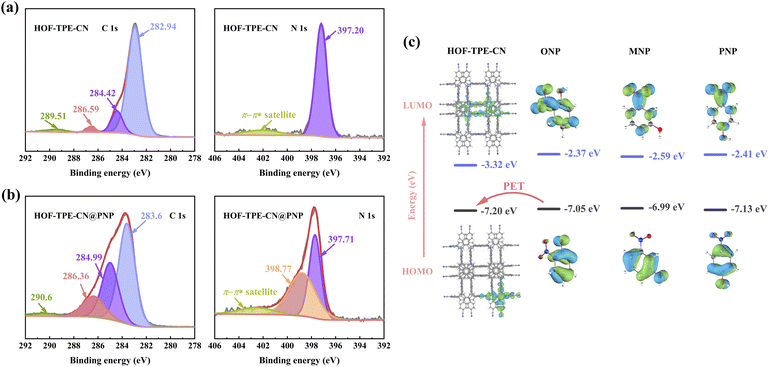 |
| Fig. 6 XPS spectra of HOF-TPE-CN before (a) and after adding PNP (b). Deconvoluted C 1s (left) and N 1s (right) high-resolution core-level XPS spectra. The solid lines with gray and red color represent the experimentally measured data and curve fitting, respectively. π–π* shakeup transitions are related to N 1s photoelectrons. A small percentage of photoelectrons emitted from the N 1s core levels can facilitate the excitation of electrons from π to π* orbitals. The emergence of these π–π* shakeup transitions is a common feature of materials involving π bonds. (c) The orbital surfaces and HOMO and LUMO energy level diagrams for the fragment of HOF-TPE-CN and nitrophenol molecules. | |
To reveal the quenching mechanism of the chemo-sensing system, time-resolved fluorescence spectroscopy was employed to evaluate the quenching dynamics. As shown in Fig. S19,† time-resolved fluorescence spectroscopy indicated that there is no obvious alteration of the fluorescence lifetime of HOF-TPE-CN (τ = 1.43 ns) upon the addition of ONP, MNP, and PNP, irrespective of the mono-nitrophenol concentration, which suggests that the behaviour of the fluorescence quenching process is static.32 Static quenching is a non-diffusional process and derives from the production of non-fluorescent ground-state charge transfer complexes through the combination of a quencher and fluorophore. The appearance of a new peak at approximately 400 nm in the UV-vis absorption spectrum of HOF-TPE-CN upon the gradual addition of PNP indicates the formation of stable ground-state charge transfer complexes (Fig. S20 and S21†).
The formation of strong ground-state complexation (GSC) may commonly lead to an efficient photo-induced electron transfer (PET),33 thus enabling effective fluorescence quenching. As we know, PET can be investigated by the method of comparing the highest occupied molecular orbital (HOMO) and lowest unoccupied molecular orbital (LUMO) energy states of substrate and analyte molecules.4a We thus performed DFT calculations to determine the HOMO and LUMO energy levels of the fragment of HOF-TPE-CN and nitrophenol molecules. As shown in Fig. 6c, the HOMO energy level of the analyte molecules (acceptor) was found to be located within the range of the HOMO and LUMO energy levels of HOF-TPE-CN, which suggests that the acceptor-PET can occur since the HOMO energy level of the acceptor is higher than that of HOF-TPE-CN.34,35 As a result, such acceptor-PET may be the possible reason for the effective fluorescence quenching for chemical sensing of mono-nitrophenols.
Conclusions
In summary, we have successfully designed and synthesized a water-stable and AIE-active HOF (HOF-TPE-CN) for highly selective and sensitive detection of ONP, MNP, and PNP in water. Due to the efficient AIE effect, the crystalline HOF-TPE-CN material showed significantly enhanced fluorescence emission, wherein the quantum yield of HOF-TPE-CN was notably increased to 21% compared with that of the TPE-CN ligand (2.89%). Furthermore, theoretical calculations combined with X-ray photoelectron spectroscopy revealed that the electron-rich cyano and phenyl groups can serve as specific binding sites to interact with electron-deficient mono-nitrophenol molecules through multiple van der Waals and hydrogen-bonding interactions. As a result, this HOF sensor exhibits highly sensitive and selective sensing of mono-nitrophenols, as evidenced by its remarkably low limit of detection of 0.65 μM for PNP. This sensing performance is even comparable to those of some of the best-performing crystalline materials reported so far. The mechanism for sensing PNP was investigated using various analytical techniques including UV-vis, XPS, fluorescence lifetime, and theoretical calculations, wherein the interactions between the framework and analyte molecules enable them to form ground-state complexation for efficient fluorescence quenching through possible photo-induced electron transfer. This work provides some guidance on how to design highly fluorescent and water-stable HOF sensors for chemical sensing applications.
Experimental section
Materials
All reagents used in the experiments were purchased from commercial companies without further purification. Among them, 4,4′,4′′,4′′′-(ethene-1,1,2,2-tetrayl) tetrabenzonitrile was purchased from Extension Company (China).
Characterization
Powder X-ray diffraction (PXRD) patterns were recorded on a PANalytical X'Pert Pro X-ray diffractometer with Cu-Kα radiation (λ = 1.542 Å) in the range of 2°–45° (2θ) at a rate of 5° min−1. Steady-state photoluminescence and excitation spectra were obtained with a Hitachi F-4600 fluorescence spectrometer equipped with a xenon lamp under ambient conditions. The fluorescence micrographs were taken on an Olympus IX71 inverted fluorescence microscope. The single crystal emitting light was focused and collected using a PG2000-Pro fiber optic spectrometer. UV-vis absorption spectroscopy spectra were collected on a UV-2600 spectrophotometer (Shimadzu Corp, Japan). X-ray photoelectron spectroscopy (XPS) was conducted on a Thermo Kalpha. Photoluminescence quantum efficiency was examined using an Edinburgh FLS1000 photoluminescence spectrometer at ambient temperature. Fluorescence lifetime was evaluated on an Edinburgh FLSP920 fluorescence spectrophotometer and an external laser was used as a light source.
Single crystal X-ray diffraction
Single-crystal X-ray diffraction data were collected on an Agilent Supernova CCD diffractometer equipped with graphite-monochromatic enhanced Mo-Kα radiation (λ = 0.71073 Å). The datasets were corrected by empirical absorption correction using spherical harmonics, implemented in the SCALE3 ABSPACK scaling algorithm. The structure was solved by direct methods using SIR92 and refined by full-matrix least-squares methods with the SHELX-2014 program package. The crystal data are summarized in Table S1.†
Synthesis of HOF-TPE-CN
The single crystals of HOF-TPE-CN were grown by slow vapor diffusion of n-hexane into an acetone solution (5 mL) of TPE-CN (60 mg) at room temperature for one week. Orange crystals were obtained by filtration and washed with n-hexane.
Fluorescence sensing experiments
A HOF suspension in water with a concentration of 1 mg mL−1 was used for the fluorescence sensing experiments. In addition, the HOF was ground ultrasonically before sensing to ensure uniform dispersion in water. The nitrophenol solution was added for at least one minute before testing to ensure fluorescence stability.
Conflicts of interest
There are no conflicts to declare.
Acknowledgements
This research was supported by the National Natural Science Foundation of China (52073251), the Zhejiang Provincial Natural Science Foundation of China (No. LR22E030003) and the Science Technology Department of Zhejiang Province (2023C01098).
Notes and references
-
(a) J. H. Song and D. W. Kang, Coord. Chem. Rev., 2023, 492, 215279 CrossRef CAS;
(b) A. Karmakar, E. Velasco and J. Li, Natl. Sci. Rev., 2022, 9, nwac091 CrossRef CAS PubMed;
(c) S. Kamal, M. Khalid, M. S. Khan and M. Shahid, Coord. Chem. Rev., 2023, 474, 214859 CrossRef CAS;
(d) Z. Hu, B. J. Deibert and J. Li, Chem. Soc. Rev., 2014, 43, 5815–5840 RSC;
(e) M. Asad, M. Imran Anwar, A. Abbas, A. Younas, S. Hussain, R. Gao, L. Li, M. Shahid and S. Khan, Coord. Chem. Rev., 2022, 463, 214539 CrossRef CAS.
-
(a) A. Lan, K. Li, H. Wu, D. H. Olson, T. J. Emge, W. Ki, M. Hong and J. Li, Angew. Chem., Int. Ed., 2009, 48, 2334–2338 CrossRef CAS;
(b) E. S. Forzani, D. Lu, M. J. Leright, A. D. Aguilar, F. Tsow, R. A. Iglesias, Q. Zhang, J. Lu, J. Li and N. Tao, J. Am. Chem. Soc., 2009, 131, 1390–1391 CrossRef CAS PubMed;
(c) S. Dalapati, S. Jin, J. Gao, Y. Xu, A. Nagai and D. Jiang, J. Am. Chem. Soc., 2013, 135, 17310–17313 CrossRef CAS PubMed;
(d) H. Xu, F. Zhong, F. Chen, T. Luan, P. Li, S. Xu and J. Gao, J. Mater. Chem. C, 2022, 10, 7469–7475 RSC;
(e) M. Rieger, M. Wittek, P. Scherer, S. Löbbecke and K. Müller-Buschbaum, Adv. Funct. Mater., 2018, 28, 1704250 CrossRef.
- L. Zhu, X. Mei, Z. Peng, J. Yang and Y. Li, J. Hazard. Mater., 2022, 435, 129012 CrossRef CAS.
-
(a) B. Wang, X. Lv, D. Feng, L. Xie, J. Zhang, M. Li, Y. Xie, J. Li and H. Zhou, J. Am. Chem. Soc., 2016, 138, 6204–6216 CrossRef CAS PubMed;
(b) M. Liu, M. Y. Wang, R. Huo, C. Wang, Y. H. Xing, F. Y. Bai and F. Xu, J. Mater. Chem. C, 2022, 10, 11676–11685 RSC.
-
(a) X. Z. Song, S. Y. Song, S. N. Zhao, Z. M. Hao, M. Zhu, X. Meng, L. L. Wu and H. J. Zhang, Adv. Funct. Mater., 2014, 24, 4034–4041 CrossRef CAS;
(b) L. Chen, Z. Yuan, H. Zhang, Y. Ye, Y. Yang, F. Xiang, K. Cai, S. Xiang, B. Chen and Z. Zhang, Angew. Chem., Int. Ed., 2022, 61, e202213959 CrossRef CAS;
(c) C. Liu, X. Chen, H. Chen, Z. Niu, H. Hirao, P. Braunstein and J. Lang, J. Am. Chem. Soc., 2020, 142, 6690–6697 CrossRef CAS;
(d) X. Gu, E. Wu, J. Wang, H. Wen, B. Chen, B. Li and G. Qian, Sci. Adv., 2023, 9, h135 Search PubMed;
(e) D. Liu, J. Pei, X. Zhang, X. Gu, H. Wen, B. Chen, G. Qian and B. Li, Angew. Chem., Int. Ed., 2023, 62, e202218590 CrossRef CAS PubMed;
(f) K. Shao, H. Wen, C. Liang, X. Xiao, X. Gu, B. Chen, G. Qian and B. Li, Angew. Chem., Int. Ed., 2022, 61, e202211523 CrossRef CAS.
-
(a) W. P. Lustig, S. Mukherjee, N. D. Rudd, A. V. Desai, J. Li and S. K. Ghosh, Chem. Soc. Rev., 2017, 46, 3242–3285 RSC;
(b) S. Wu, H. Min, W. Shi and P. Cheng, Adv. Mater., 2019, 32, 1805871 CrossRef;
(c) Y. Cui, J. Zhang, H. He and G. Qian, Chem. Soc. Rev., 2018, 47, 5740–5785 RSC;
(d) S. Xing and C. Janiak, Chem. Commun., 2020, 56, 1229–1236 Search PubMed.
-
(a) X. Liu, D. Huang, C. Lai, G. Zeng, L. Qin, H. Wang, H. Yi, B. Li, S. Liu, M. Zhang, R. Deng, Y. Fu, L. Li, W. Xue and S. Chen, Chem. Soc. Rev., 2019, 48, 5266–5302 RSC;
(b) X. Wu, X. Han, Q. Xu, Y. Liu, C. Yuan, S. Yang, Y. Liu, J. Jiang and Y. Cui, J. Am. Chem. Soc., 2019, 141, 7081–7089 CrossRef CAS PubMed;
(c) Z. Li, N. Huang, K. H. Lee, Y. Feng, S. Tao, Q. Jiang, Y. Nagao, S. Irle and D. Jiang, J. Am. Chem. Soc., 2018, 140, 12374–12377 CrossRef CAS;
(d) D. Yan, Z. Wang, P. Cheng, Y. Chen and Z. Zhang, Angew. Chem., Int. Ed., 2021, 60, 6055–6060 CrossRef CAS PubMed.
-
(a) H. Wang, X. Dong, J. Lin, S. J. Teat, S. Jensen, J. Cure, E. V. Alexandrov, Q. Xia, K. Tan, Q. Wang, D. H. Olson, D. M. Proserpio, Y. J. Chabal, T. Thonhauser, J. Sun, Y. Han and J. Li, Nat. Commun., 2018, 9, 1745 CrossRef PubMed;
(b) D. Zhao, D. J. Timmons, D. Yuan and H. Zhou, Acc. Chem. Res., 2011, 44, 123–133 CrossRef CAS PubMed;
(c) S. Xing, J. Liang, P. Brandt, F. Schäfer, A. Nuhnen, T. Heinen, I. Boldog, J. Möllmer, M. Lange, O. Weingart and C. Janiak, Angew. Chem., Int. Ed., 2021, 60, 17998–18005 CrossRef CAS;
(d) C. Wang, G. Zeng, Z. You, Y. Xing, F. Bai and L. Sun, Inorg. Chem., 2022, 61, 10066–10078 CrossRef CAS.
-
(a) R. Lin, Y. He, P. Li, H. Wang, W. Zhou and B. Chen, Chem. Soc. Rev., 2019, 48, 1362–1389 RSC;
(b) B. Wang, R. Lin, Z. Zhang, S. Xiang and B. Chen, J. Am. Chem. Soc., 2020, 142, 14399–14416 CrossRef CAS PubMed;
(c) M. R. di Nunzio, I. Hisaki and A. Douhal, J. Photochem. Photobiol.,
C, 2021, 47, 100418 CrossRef CAS;
(d) S. Cai, Z. An and W. Huang, Adv. Funct. Mater., 2022, 32, 2207145 CrossRef CAS.
-
(a) X. Song, Y. Wang, C. Wang, D. Wang, G. Zhuang, K. O. Kirlikovali, P. Li and O. K. Farha, J. Am. Chem. Soc., 2022, 144, 10663–10687 CrossRef CAS PubMed;
(b) Y. He, S. Xiang and B. Chen, J. Am. Chem. Soc., 2011, 133, 14570–14573 CrossRef CAS PubMed;
(c) W. Yang, A. Greenaway, X. Lin, R. Matsuda, A. J. Blake, C. Wilson, W. Lewis, P. Hubberstey, S. Kitagawa, N. R. Champness and M. Schröder, J. Am. Chem. Soc., 2010, 132, 14457–14469 CrossRef CAS.
-
(a) Y. Yang, L. Li, R. Lin, Y. Ye, Z. Yao, L. Yang, F. Xiang, S. Chen, Z. Zhang, S. Xiang and B. Chen, Nat. Chem., 2021, 13, 933–939 CrossRef CAS PubMed;
(b) Y. Li, E. V. Alexandrov, Q. Yin, L. Li, Z. Fang, W. Yuan, D. M. Proserpio and T. Liu, J. Am. Chem. Soc., 2020, 142, 7218–7224 CrossRef CAS PubMed;
(c) L. Li, H. Ma, J. Zhang, E. Zhao, J. Hao, H. Huang, H. Li, P. Li, X. Gu and B. Z. Tang, J. Am. Chem. Soc., 2021, 143, 3856–3864 CrossRef CAS PubMed;
(d) Q. Huang, W. Li, Z. Mao, H. Zhang, Y. Li, D. Ma, H. Wu, J. Zhao, Z. Yang, Y. Zhang, L. Gong, M. P. Aldred and Z. Chi, Chem, 2021, 7, 1321–1332 CrossRef CAS;
(e) B. Yu, S. Geng, H. Wang, W. Zhou, Z. Zhang, B. Chen and J. Jiang, Angew. Chem., Int. Ed., 2021, 60, 25942–25948 CrossRef CAS PubMed;
(f) Q. Huang, W. Li, Z. Mao, L. Qu, Y. Li, H. Zhang, T. Yu, Z. Yang, J. Zhao, Y. Zhang, M. P. Aldred and Z. Chi, Nat. Commun., 2019, 10, 3074 CrossRef.
-
(a) J. Gao, Y. Cai, X. Qian, P. Liu, H. Wu, W. Zhou, D. X. Liu, L. Li, R. B. Lin and B. Chen, Angew. Chem., Int. Ed., 2021, 60, 20400–20406 CrossRef CAS PubMed;
(b) I. Hisaki, C. Xin, K. Takahashi and T. Nakamura, Angew. Chem., Int. Ed., 2019, 58, 11160 CrossRef CAS PubMed;
(c) F. Hu, C. Liu, M. Wu, J. Pang, F. Jiang, D. Yuan and M. Hong, Angew. Chem., Int. Ed., 2017, 56, 2101–2104 CrossRef CAS.
-
(a) P. Cui, E. Svensson Grape, P. R. Spackman, Y. Wu, R. Clowes, G. M. Day, A. K. Inge, M. A. Little and A. I. Cooper, J. Am. Chem. Soc., 2020, 142, 12743–12750 CrossRef CAS PubMed;
(b) A. Pulido, L. Chen, T. Kaczorowski, D. Holden, M. A. Little, S. Y. Chong, B. J. Slater, D. P. McMahon, B. Bonillo, C. J. Stackhouse, A. Stephenson, C. M. Kane, R. Clowes, T. Hasell, A. I. Cooper and G. M. Day, Nature, 2017, 543, 657–664 CrossRef CAS.
-
(a) B. Wang, R. He, L. Xie, Z. Lin, X. Zhang, J. Wang, H. Huang, Z. Zhang, K. S. Schanze, J. Zhang, S. Xiang and B. Chen, J. Am. Chem. Soc., 2020, 142, 12478–12485 CrossRef CAS PubMed;
(b) X. Xu and B. Yan, Adv. Mater., 2023, 35, 2303410 CrossRef CAS;
(c) G. Xia, Z. Jiang, S. Shen, K. Liang, Q. Shao, Z. Cong and H. Wang, Adv. Opt. Mater., 2019, 7, 1801549 CrossRef;
(d) Z. Lin, J. Qin, X. Zhan, K. Wu, G. Cao and B. Chen, ACS Appl. Mater. Interfaces, 2022, 14, 21098–21105 CrossRef CAS PubMed;
(e) I. Hisaki, Y. Suzuki, E. Gomez, Q. Ji, N. Tohnai, T. Nakamura and A. Douhal, J. Am. Chem. Soc., 2019, 141, 2111–2121 CrossRef CAS.
- I. Hisaki, Y. Suzuki, E. Gomez, B. Cohen, N. Tohnai and A. Douhal, Angew. Chem., Int. Ed., 2018, 130, 12832–12837 CrossRef.
-
(a) X. Zhang, L. Li, J. Wang, H. Wen, R. Krishna, H. Wu, W. Zhou, Z. Chen, B. Li, G. Qian and B. Chen, J. Am. Chem. Soc., 2020, 142, 633–640 CrossRef CAS;
(b) Q. Yin, P. Zhao, R. Sa, G. Chen, J. Lü, T. Liu and R. Cao, Angew. Chem., Int. Ed., 2018, 57, 7691–7696 CrossRef CAS PubMed.
-
(a) N. B. Shustova, B. D. McCarthy and M. Dincă, J. Am. Chem. Soc., 2011, 133, 20126–20129 CrossRef CAS;
(b) Y. Guo, X. Feng, T. Han, S. Wang, Z. Lin, Y. Dong and B. Wang, J. Am. Chem. Soc., 2014, 136, 15485–15488 CrossRef CAS;
(c) Z. Wei, Z. Gu, R. K. Arvapally, Y. Chen, R. N. McDougald, J. F. Ivy, A. A. Yakovenko, D. Feng, M. A. Omary and H. Zhou, J. Am. Chem. Soc., 2014, 136, 8269–8276 CrossRef CAS PubMed;
(d) M. Zhang, G. Feng, Z. Song, Y. Zhou, H. Chao, D. Yuan, T. T. Y. Tan, Z. Guo, Z. Hu, B. Z. Tang, B. Liu and D. Zhao, J. Am. Chem. Soc., 2014, 136, 7241–7244 CrossRef CAS.
-
(a) S. Dalapati, E. Jin, M. Addicoat, T. Heine and D. Jiang, J. Am. Chem. Soc., 2016, 138, 5797–5800 CrossRef CAS PubMed;
(b) H. Ding, J. Li, G. Xie, G. Lin, R. Chen, Z. Peng, C. Yang, B. Wang, J. Sun and C. Wang, Nat. Commun., 2018, 9, 5234 CrossRef CAS PubMed.
-
(a) Y. Hong, J. W. Y. Lam and B. Z. Tang, Chem. Soc. Rev., 2011, 40, 5361–5388 RSC;
(b) J. Mei, N. L. C. Leung, R. T. K. Kwok, J. W. Y. Lam and B. Z. Tang, Chem. Rev., 2015, 115, 11718–11940 CrossRef CAS PubMed.
- M. Faheem, S. Aziz, X. Jing, T. Ma, J. Du, F. Sun, Y. Tian and G. Zhu, J. Mater. Chem. A, 2019, 7, 27148–27155 RSC.
-
(a) M. C. Das, S. C. Pal and B. Chen, Joule, 2022, 6, 22–27 CrossRef;
(b) Z. Yuan, X. Jiang, L. Chen, J. Chen, L. Li, Y. Yang, Y. Li, F. Xiang, S. Xiang, B. Chen and Z. Zhang, CCS Chem., 2023, 1–9 Search PubMed.
- Y. Shi, S. Wang, W. Tao, J. Guo, S. Xie, Y. Ding, G. Xu, C. Chen, X. Sun, Z. Zhang, Z. He, P. Wei and B. Z. Tang, Nat. Commun., 2022, 13, 1882 CrossRef CAS.
- Z. Li, F. Jiang, M. Yu, S. Li, L. Chen and M. Hong, Nat. Commun., 2022, 13, 2142 CrossRef CAS PubMed.
-
(a) Y. Suzuki, N. Tohnai and I. Hisaki, Chem.–Eur. J., 2020, 26, 17056–17062 CrossRef CAS PubMed;
(b) J. Xiong, K. Wang, Z. Yao, B. Zou, J. Xu and X. Bu, ACS Appl. Mater. Interfaces, 2018, 10, 5819–5827 CrossRef CAS PubMed.
- Q. L. Guan, F. Xu, Y. Xiao, Z. X. You, F. Y. Bai and Y. H. Xing, Adv. Mater. Interfaces, 2022, 9, 2201547 CrossRef CAS.
- S. Jiang, L. Meng, M. Lv, F. Bai, W. Tian and Y. Xing, Microporous Mesoporous Mater., 2023, 348, 112408 CrossRef CAS.
- C. Zhang, F. Sun and Y. He, ACS Appl. Mater. Interfaces, 2023, 15, 9970–9977 CrossRef CAS PubMed.
-
(a) T. Luo, P. Das, D. L. White, C. Liu, A. Star and N. L. Rosi, J. Am. Chem. Soc., 2020, 142, 2897–2904 CrossRef CAS;
(b) H. M. Cezar, S. Canuto and K. Coutinho, J. Chem. Inf. Model., 2020, 60, 3472–3488 CrossRef CAS PubMed;
(c) Z. Hu, W. P. Lustig, J. Zhang, C. Zheng, H. Wang, S. J. Teat, Q. Gong, N. D. Rudd and J. Li, J. Am. Chem. Soc., 2015, 137, 16209–16215 CrossRef CAS.
- A. Cano, L. Reguera, M. Avila, D. Velasco Arias and E. Reguera, Eur. J. Inorg. Chem., 2020, 2020, 137–145 CrossRef CAS.
-
(a) H. Yin, K. Tan, S. Jensen, S. J. Teat, S. Ullah, X. Hei, E. Velasco, K. Oyekan, N. Meyer, X. Wang, T. Thonhauser, X. Yin and J. Li, Chem. Sci., 2021, 12, 14189–14197 RSC;
(b) N. K. R. Bogireddy, R. Cruz Silva, M. A. Valenzuela and V. Agarwal, J. Hazard. Mater., 2020, 386, 121643 CrossRef CAS PubMed.
-
(a) S. Q. Lud, M. Steenackers, R. Jordan, P. Bruno, D. M. Gruen, P. Feulner, J. A. Garrido and M. Stutzmann, J. Am. Chem. Soc., 2006, 128, 16884–16891 CrossRef CAS PubMed;
(b) M. Wasilewska, A. W. Marczewski, A. Deryło-Marczewska and D. Sternik, J. Environ. Chem. Eng., 2021, 9, 105459 CrossRef CAS;
(c) Y. Tang, H. Huang, B. Peng, Y. Chang, Y. Li and C. Zhong, J. Mater. Chem. A, 2020, 8, 16542–16550 RSC.
- A. Sharma, D. Kim, J. Park, S. Rakshit, J. Seong, G. H. Jeong, O. Kwon and M. S. Lah, Commun. Chem., 2019, 2, 39 CrossRef.
- D. Escudero, Acc. Chem. Res., 2016, 49, 1816–1824 CrossRef CAS PubMed.
- W. Sun, M. Li, J. Fan and X. Peng, Acc. Chem. Res., 2019, 52, 2818–2831 CrossRef CAS PubMed.
-
(a) X. Yu, A. A. Ryadun, D. I. Pavlov, T. Y. Guselnikova, A. S. Potapov and V. P. Fedin, Angew. Chem., Int. Ed., 2023, 62, e202306680 CrossRef CAS;
(b) Q. Huang, K. I. Otake and S. Kitagawa, Angew. Chem., Int. Ed., 2023, 62, e202310225 CrossRef CAS.
Footnotes |
† Electronic supplementary information (ESI) available: Additional graphics (Fig. S1–S21), tables (Tables S1 and S2) and calculation methods. See DOI: https://doi.org/10.1039/d3ta05309a |
‡ These authors have contributed equally to this work. |
|
This journal is © The Royal Society of Chemistry 2024 |