Core–shell BaPP@BDO-Rh benzimidazole oligomer photocatalyst can effectively promote NADH regeneration and CO2 conversion to fumarate†
Received
21st August 2023
, Accepted 1st November 2023
First published on 5th December 2023
Abstract
The clean energy potential of photoenzyme catalysis has gained significant attention amidst the environmental and energy crisis. BaPP@BDO-Rh, a hydrophilic core–shell catalyst, was developed for photocatalytic NADH regeneration. It incorporates benzimidazole oligomers onto BaSO4's surface and pairs it with [Cp*Rh(bpy)H2O]2+. Its photocatalytic regeneration NADH conversion frequency (TOF) of 113.16 h−1 is the highest reported. Furthermore, when coupled with malate dehydrogenase (MaeB) and fumarate hydrase (FumC), the catalyst effectively converted CO2 to fumarate, producing 79 μM of fumaric acid after a 3 hour photoenzyme reaction. This study introduces for the first time in the field of photoenzyme catalysis a high-performance oligomer photocatalyst and facilitates the high-value utilization of CO2.
Introduction
Drawing inspiration from natural plant photosynthesis, researchers have made significant strides in developing artificial photoenzyme catalytic systems for various applications such as CO2 reduction, pollutant degradation, and biomass conversion.1–3 Photoenzyme catalysis represents a sustainable and environmentally friendly approach to biomanufacturing, as it combines the light-collecting ability of photocatalysts with the high selectivity of enzymes to convert clean and inexhaustible light energy into chemical energy.4–6 Since 1961, the chloroplasts of spinach have been employed for photoenzyme catalysis, and subsequent studies have explored the use of photocatalysts like titanium dioxide and graphite-phase nitrogen carbide.3,7–11 However, the current efficiency of regenerating photocatalytic cofactors and converting light energy remains relatively low.12 Consequently, a key strategy in this field is to design and develop more efficient photocatalysts to enhance cofactor regeneration efficiency.
Photoenzyme catalysis is a biomimetic engineering approach that emulates natural photosynthesis. In natural photosynthesis, large quantities of pigments and proteins are distributed across thylakoid membranes to capture light energy, facilitate electron transfer, and regenerate NADPH (Scheme 1a). Specifically, chloroplasts capture visible light from sunlight, which is then transferred to protein/pigment complexes.13 These complexes convert light energy into electrical energy and subsequently into chemical energy through continuous REDOX reactions. The chemical energy is stored in energy-rich molecules, and NADPH is reduced. NADPH then drives a series of enzymatic reactions that convert CO2 into organic compounds such as starch.14,15
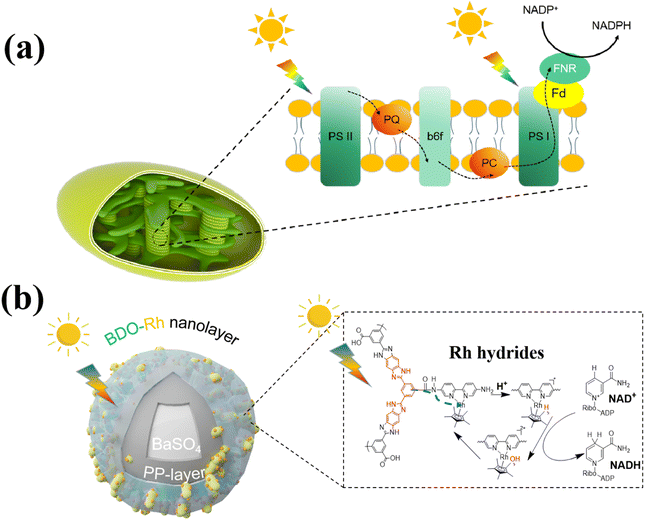 |
| Scheme 1 Synergistic intensification of electron generation, transfer and utilization enabled by (a) thylakoid for NADPH regeneration and (b) BaPP@BDO-Rh for NADH regeneration. | |
Polymeric semiconductors have garnered significant attention as photocatalysts in recent years.16–18 These semiconductors, including oligomers, amorphous polymers, and covalent organic frameworks (COFs), offer extended conjugation for efficient exciton transfer and exceptional stability.19 In particular, the benzodiimidazole oligomer (BDOs20) exhibits unique short-range crystallization properties, promoting the ordered accumulation of fused rings due to the interplay between oligomer chains and side-kyl-aid assembly. This short-range crystallinity reduces carrier transfer resistance, thereby improving photocatalytic performance. Additionally, BDO produced by hydrogels possesses inherent hydrophilicity, ensuring ample absorption of water-soluble molecules and facilitating surface reactions. While BDO has demonstrated excellent photocatalytic activity in water decomposition, its application in photocatalytic cofactor regeneration remains unexplored in the literature.
Excessive emissions of CO2 have led to widespread and significant environmental and social problems.21,22 As a result, there is considerable interest and rapid development in technologies aimed at converting CO2 into high-value chemicals.23–25 Fumarate, a versatile organic compound, finds applications in various industries such as food, medicine, cosmetics, and industrial manufacturing. In the food industry, it serves as an acid regulator, preservative, and antioxidant.26 Fumarate also has extensive uses in the pharmaceutical and cosmetic sectors, where it acts as a solvent, stabilizer, and pH regulator in drug formulations and skincare products.27 Additionally, fumarate is employed as a raw material in the production of polyester resins, paints, plastics, and other chemical compounds.28,29 While traditional chemical synthesis methods face challenges, biological enzyme-mediated CO2-to-fumarate conversion has been reported.30,31 However, the low efficiency and conversion rates of this approach severely limit its industrial application, necessitating the development of more efficient photocatalysts for NADH regeneration to enhance efficiency and reduce costs. In our study, we drew inspiration from the structure and function of thylakoid membranes and prepared nanolayers loaded with benzimidazole oligomers on the surface of barium sulfate, combined with [Cp*Rh(Bpy)H2O]2+ (BaPP@BDO-Rh), for photocatalytic NADH regeneration. The core–shell structure of the BaPP@BDO-Rh catalyst's surface incorporates hydrophilic short-range crystalline BDO to capture light energy, providing a superthin film structure that restricts electron transfer pathways. Additionally, the Rh catalytic center32 is anchored to accept and store electrons, enabling NADH regeneration through synergistic enhancement and utilization of electron generation and transfer (Scheme 1b). The production and transfer of electrons can be controlled by adjusting the thickness of BDO, while the utilization of electrons can be modulated by altering the amount of Rh coordination. To form a photoenzyme catalytic system, the NADH regeneration system is further combined with a CO2-to-fumarate synthesis system catalyzed by malate dehydrogenase and fumarate hydrase.
Experimental
Reagents and materials
All reagent grade chemicals were purchased from Aladdin and Sigma-Aldrich unless otherwise noted. Biochemical substances and materials for cloning and expression are derived from Thermo Fisher.
The scientific primers and synthetic genes are from GENEWIZ Co., LTD. Materials and equipment proteins used for protein purification are derived from Bio-Rad.
Preparation of BaSO4
Refer to previous literature reports33 and make some modifications. Barium chloride (0.005 M) was dissolved in 1 L deionized water (Use NaOH to adjust pH to 12) stirred at room temperature at 300 rpm. MGDA-3Na was added to the solution and the concentration reached 0.005 M. Slowly add 0.001 M NaOH solution to the above solution and keep the pH at 12. After the addition of NaOH, it was aged for 2 h. Finally, the white solids in the solution were collected by centrifugation, washed twice with water and ethanol, and dried in 70 °C air overnight.
Preparation of BaPP
Refer to the preparation method of previous literature.32 First, more than 1 g of BaSO4 powder was evenly dispersed in a tris buffer (50 mM, pH 8.5) and stirred for approximately 10 minutes to obtain a white suspension. Subsequently, dopamine hydrochloride (2 g L−1) and polyvinylimine (2 g L−1) were added to the aforementioned white BaSO4 medium and stirred at room temperature for 4 h. The resulting solution turned into a dark gray color. It was collected and centrifuged multiple times. Finally, the BaSO4-PDA/PEI composite (referred to as BaPP) powder was dried in a vacuum oven at 40 °C for 24 h.
Preparation of BDO
Refer to previous literature reports20 with minor modifications. 568 mg (2 mmol) benzene-1,2,4,5-trieacetamide (BTA) and 672 mg (3.2 mmol) 1,3,5-tricarboxybenzene (TCB) were added to 45 g polyphosphoric acid (PPA) and mechanically stirred at 150 °C for 72 h. The reaction system is neutralized with sodium hydroxide to remove PPA. After neutralization, the mixture is reheated to 80 °C and then centrifuged with heat. The obtained solid samples were dried overnight in 70 °C air to obtain soaked BDO (BDO precursor). Then, 100 mg of soaked BDO was dissolved in 40 mL water, acidified with sulfuric acid (4 M, 5 mL) and centrifuged to form a bright yellow BDO hydrogel. BDO hydrogels were then extracted for 48 h by Soxhlet and the unreacted precursors were removed. Finally, freeze-dried powder samples were obtained. The solid state 13C NMR spectra of BDO are basically consistent with the ref. 20.
Preparation of BaPP@BDO
The preparation process of BaPP@BDO is basically the same as that of BDO. The difference is that BAPP of different qualities (300 mg, 600 mg, 900 mg and 1200 mg) is added to polyphosphate first, then TCB and BTA are added.
Preparation of BaPP@BDO-Rh
200 mg of BaPP@BDO was dispersed in 20 mL DMF, followed by 500 mg of 1-ethyl-(3-dimethylaminopropyl) carbodiimide hydrochloride and 20 mg of 1-hydroxybenzotriazole at room temperature for 1 h. After completion, 200 mg of 5,5-diamino-2,2-bipyridine was added into the solution, and the reaction was stirred magnetically for 24 h. After centrifugation, the precipitation was washed with ethanol to obtain a solid sample. It is freeze-dried to obtain BaPP@BDO-Bpy.
50 mg BaPP@BDO-Bpy was placed in 40 mL methanol and dispersed by ultrasonic for 5 min. Dichloro (pentamethylcyclopentadienyl) rhodium(III) dimers of different weights (5 mg, 10 mg, 20 mg, 40 mg) were added into the suspension and stirred at room temperature for 24 h. The solution was centrifuged and collected into solid, washed with water and methanol 3 times, and freeze-dried to obtain BaPP@BDO-Rh.
Photoelectrochemical measurement
All photochemical measurements were performed by an electrochemical workstation (CHI660) using a typical three-electrode system (reference electrode, counter electrode and working electrode). Here, Ag/AgCl was selected as the reference electrode, platinum wire was selected as the counter electrode, and the obtained sample was prepared as the working electrode. The working electrode was prepared by dispersing 1 mg sample into 5% Nafion solution (100 μL) and dropping the mixture onto a 1.0 × 1.5 cm2 FTO glass electrode. The electrodes are then dried overnight at room temperature. The electrolyte is 0.5 M sodium sulfate. Xenon lamp with power of 100 W is used as light source.
Photocatalytic regeneration of NADH
A photocatalytic NADH regeneration reactor was performed in a quartz flask with the addition of 3 mL reaction mixture. The reaction system contains 1 g L−1 photocatalyst, 1 mM of NAD+, 1000 mM of TEOA and 100 mM of PBS (pH = 7.5). For BDO and BaPP@ BDO, add [CP*Rh(Bpy)H2O]2+ equal to the concentration of element Rh in BaPP@ BDO-Rh at 0.0183 mM. NADH was detected by nuclear magnetic resonance spectrometer and spectrophotometer.
Expression and purification of enzymes
Protein expression plasmids pET28a-MaeB and pET28a-FumB were constructed by using prolonged overlap extension PCR (POE-PCR) and transferred into E. coli strain DE3 (BL 21).34 Overexpression of MaeB and FumC was performed in Luria–Bertani (LB) medium containing 50 μg mL−1 kanamycin and 0.1 mM isopropyl-β-D-thiogalactopyranoside (IPTG) in flasks shaking at 16 °C for 20 h. Cells were harvested and resuspended in 50 mM PBS buffer (pH 7.5) containing 200 mM NaCl, then lysed by high-pressure homogenization at 4 °C. After centrifugation at 12
000 rpm for 20 min, the His-tagged MaeB and FumC were purified by Ni-NTA resin columns. The protein concentration was determined by the Bradford method. Purified enzymes were added with glycerol at a final concentration of 5% and stored at −20 °C before use.
Enzyme activity assays
One activity unit of MaeB convert 1.0 μmol of NADH to NAD+ in the presence of 10 mM sodium pyruvate, 0.3 mM NADH, 10 mM sodium bicarbonate and 10 mM MgCl2 in 50 mM 1,4-piperazinediethanesulfonic acid KOH buffer per min at pH 7.5 at 37 °C according to the data sheet provided by Thermostable Enzyme Laboratory Co., Ltd. The molecular weight of MaeB was determined to be 86.25 kDa based on the SDS-PAGE of electrophoresis (Fig. S15†).
FumC activity was defined as adding 5 mM fumararte and an appropriate amount of enzyme solution to 100 mM PBS buffer for 10 min to detect fumararte concentration. The concentration of fumararte is obtained by absorbance at 250 nm. The molecular weight of FumC was 49.52 kDa as determined by SDS-page electrophoresis (Fig. S15†).
Enzyme-photo-coupled catalytic synthesis of fumarate
The 3 mL photoenzyme reaction system contains 1 g L−1 photocatalyst, 1 mM of NAD+, sodium pyruvate (5.0 mM), sodium bicarbonate (100 mM), magnesium chloride (5.0 mM), MaeB (1 U) and FumC (1 U),1000 mM of TEOA and 100 mM of PBS (pH = 7.5). The sample solution was illuminated by a 100 W xenon lamp at room temperature as a visible light source. Fumarate was detected by ion chromatography and NMR.
Characterizations
The morphology of the materials was analyzed by transmission electron microscopy (TEM, JEOL JEM F200 and HT7700) and scanning electron microscopy (SEM, Hitachi Regulus 8100). X-ray powder diffraction (XRD) patterns was performed by an X-ray powder diffractometer (Shimadzu, XDR-6000). X-ray photoelectron spectroscopy (XPS) was obtained using an X-ray photoelectron spectrometer (Axis Supra). Fourier transform infrared spectrum was collected by Thermo Nicolet-6700 spectrometer. UV-vis absorption spectrum was measured with an ultraviolet spectrophotometer (Shimazu, UV-2501PC). The photoluminescence (PL) spectra were obtained using an X-ray fluorescence spectrometer (Shimazu, XRF-1800).
Results and discussion
Preparation and characterizations of photocatalysts
BaPP@BDO-Rh is typically prepared through a synthesis process involving the growth of BaPP@BDO on the surface of polydopamine/polyimide (PDA/PEI) modified BaSO4, followed by the addition of a Rh-cocatalyst. The schematic diagram of the preparation process is illustrated in Fig. 1a. Initially, white BaSO4 particles with a diameter of 200 nm are modified with PEI/PDA to obtain grayish white BaPP (Fig. S2 and S3†). Subsequently, 1,3,5-tricarboxylbenzene (TCB) is used to graft TCB onto BaPP through a condensation reaction between the amino and carboxyl groups. The addition of benzene-1,2,4,5-troamine (BTA) to TCB leads to a condensation reaction, resulting in the formation of a bright yellow BaPP@BDO hydrogel. Finally, the carboxyl group of BaPP@BDO reacts with the amino group of 2,2′-bipyridine-5,5′-diamine (Bpy), and (Cp*RhCl2)2 coordinates with Bpy to form the bright yellow BaPP@BDO-Rh hydrogel. The BaPP@BDO-Rh structure exhibits a spherical core–shell morphology, with the BDO-Rh layer having a thickness of approximately 16 nm (Fig. 1b–d), and the PEI/PDA (PP) layer measuring around 4.2 nm (Fig. S4a†). The zeta potential changes of BaSO4 (−23.23 mv) and BaPP (9.23 mv) further indicate the existence of PP layer (Fig. S4b†). Elemental mapping of BaPP@BDO-Rh, as shown in Fig. 1e–g and S5,† indicates that Ba and O originate from BaSO4, while C, N, and Rh originate from the BDO-Rh nanolayer. Additionally, the material displays excellent hydrophilicity due to the presence of exposed carboxyl groups on the surface (Fig. S6†). The stable dispersion of 1 mg L−1 BaPP@BDO-Rh in deion (Fig. 5a) depends on the abundance of COO– in BDO precursors. The modifications of PEI and PDA play a crucial role in the formation of the core–shell structure BaPP@BDO (Fig. S7†). It should be noted that BDO and barium sulfate without amination are unable to form the desired core–shell structure.
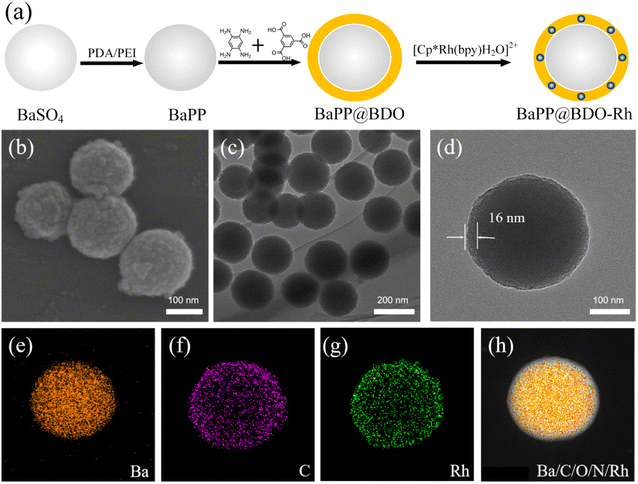 |
| Fig. 1 (a) Schematic preparation of BaPP@BDO-Rh photocatalyst. (b) SEM images of BaPP@BDO-Rh. (c and d) TEM image of BaPP@BDO-Rh and corresponding element mappings of (e) Ba, (f) C, (g) Rh and (h) Ba/C/O/N/Rh. | |
In order to more clearly analyze the structure and composition of BaPP@BDO-Rh, Fourier transform infrared spectroscopy (FTIR), X-ray powder diffraction (XRD) and X-ray photoelectron spectroscopy (XPS) were used. The peak at 1567 cm−1 in Fig. 2a is the C
N stretching vibration of benzodiimidazole. In addition, O–H bending (1321 cm−1) and C
O stretching (1695 cm−1) were observed in the infrared spectra of BDO, BaPP@BDO-MC and BaPP@BDO-MC-Rh, indicating the presence of abundant carboxyl groups. This corresponds to their hydrophilicity (Fig. S6†). The C–NH of 1153 cm−1 is a peptide bond formed by the reaction of the carboxyl group on BaPP@BDO with the amino group of Bpy. BaPP@BDO and BaPP@BDO-Rh inherit the crystalline character of BaSO4, although BDO crystallizes over a short distance due to the antagonism between the fused benzodiimdazole rings (Fig. 2b). XPS is an important surface analysis technology, which can know the type of elements, chemical state and other information. As shown in Fig. 2c&e, the presence of Rh can be confirmed from the XPS energy spectrum of BaPP@BDO-Rh. The N 1s peak (Fig. 2c&d) in BaPP indicates the presence of the PEI/PDA layer, which corresponds to Fig. S4.† Among them, BaPP's high resolution N 1s XPS spectrum in 400.5 eV, 400.2 eV and 399.0 eV three peaks, respectively belong to the PEI/PDA C–NH2, C–N3 and C–N
C tensile vibration. The peak of BaPP@BDO at 397.4 eV is the tensile vibration of C–NH–C in BDO (Fig. 2d). Because the BDO layer covers the surface of BaSO4 and has a thickness of 16 nm, it exceeds the XPS penetration depth (3–10 nm).35 So there are no peaks for S and Ba in BaPP@BDO (Fig. 2c&f). To sum up,BaPP@BDO-Rh is successfully synthesized.
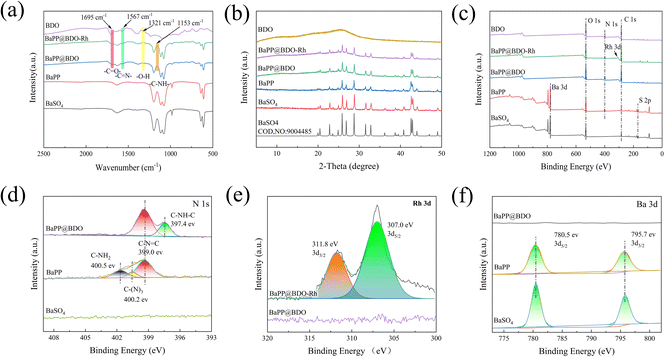 |
| Fig. 2 (a) FTIR spectra, (b) XRD patterns and (c) full-scan XPS spectra of BaSO4, BaPP, BaPP@BDO, BaPP@BDO-Rh and BDO. High-resolution XPS (d) N 1s, (e) Rh 3d and (f) Ba 3d spectra of BaSO4, BaPP and BaPP@BDO. | |
Optical electronic properties of photocatalysts
The UV-vis diffuse reflectance spectrum revealed that the light absorption range of BaPP@BDO-Rh increased from 480 nm to 405 nm and 409 nm compared to BDO-Rh alone. This enhancement in light absorption can be attributed to the conjugated structure present in BDO and Bpy (Fig. 3a). The calculated bandgap of BaPP@BDO-Rh, obtained from the Tauc diagram, was found to be narrower than that of BaPP@BDO. This indicates that both samples exhibit favorable light absorption properties (Fig. 3b). The conduction bands (CB) of these samples were determined using the Mott–Schottky curve, and it was observed that they all exhibited N-type semiconductor behavior (Fig. 3c). From the information presented in Fig. 3b and c, the positions of their conduction bands (−0.95 eV, −1.13 eV, and −1.06 eV) and valence bands (1.75 eV, 1.70 eV, and 1.53 eV) can be determined. Based on their redox properties, as depicted in Fig. 3d, it can be inferred that they have the ability to oxidize TEOA and reduce NADH.
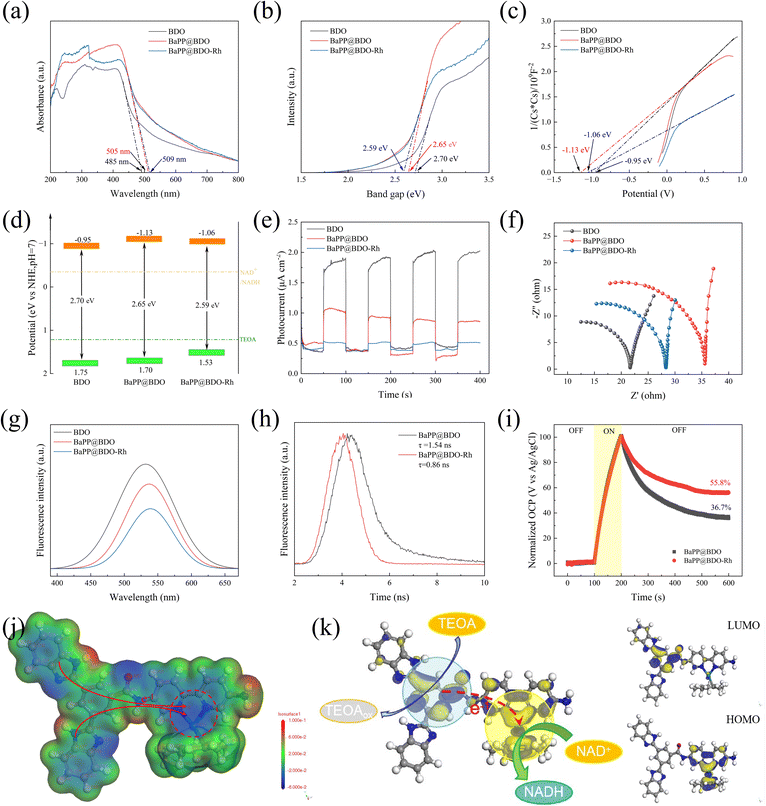 |
| Fig. 3 (a) UV-vis diffuse reflectance spectra, (b) Tauc plot spectra, (c) Mott–Schottky valence band spectra and (d) schematic band structure of BDO, BaPP@BDO and BaPP@BDO-Rh. (e) Photocurrent responses, (f) EIS Nyquist plots and (g) steady state PL spectra of BDO, BaPP@BDO and BaPP@BDO-Rh. (h) Time-resolved PL spectra and (i) open circuit potential of BaPP@BDO and BaPP@BDO-Rh. (j) Theoretical modeling of molecular electrostatic potential (ESP) and (k) HOMO/LUMO energy of BaPP@BDO-Rh. | |
When light interacts with a semiconductor material, it leads to the separation of electrons and holes, resulting in the generation of an electric current. The magnitude of the photocurrent reflects the efficiency of separating photogenerated electrons and holes. In the case of BaPP@BDO-Rh, the photocurrent is 4.0 times and 11.5 times higher compared to BaPP@BDO and BDO, respectively (Fig. 3e). This indicates that BaPP@BDO-Rh exhibits a higher efficiency in separating photogenerated electrons from photogenerated holes. The efficiency of electron transfer can be assessed by examining the steady state photoluminescence (PL) spectra. In this context, the PL peak intensity of BaPP@BDO-Rh is the weakest, indicating that the electron–hole pairs generated in BaPP@BDO-Rh are less prone to recombine (Fig. 3g). Moreover, the smaller internal resistance of BaPP@BDO-Rh facilitates the efficient utilization of all photo-excited free electrons by NADH instead of their release as fluorescence (Fig. 3f). These low resistance properties can be attributed to local dipole changes and the nanometer scale crystallinity in BDO, which create efficient pathways for charge conduction over short distances. Time-resolved PL spectroscopy is another characterization method used to evaluate the separation efficiency of photogenerated charges and holes in photocatalyst semiconductors. BaPP@BDO-Rh (0.85 ns) exhibits a shorter electron lifetime compared to BaPP@BDO (1.54 ns) because the photogenerated electrons in the BDO layer are effectively anchored in Rh, thereby inhibiting the recombination of photogenerated electrons and holes (Fig. 3h). Additionally, the electron transfer efficiency36 can be analyzed by measuring the open circuit voltage (Fig. 3i). After 100 s of light irradiation, the photogenerated electrons and holes become separated, resulting in an increase in the open circuit voltage. When the light is turned off, some electrons and holes recombine, leading to a decrease in the open circuit voltage. However, even after 400 seconds (from 200–600 s), the electron utilization efficiency of BaPP@BDO-Rh remains 52% higher than that of BaPP@BDO, with a value of 55.8% compared to 36.7%. In conclusion, the Rh-loaded core–shell structure hydrogel-type photocatalyst, BaPP@BDO-Rh, effectively suppresses the recombination of photogenerated electrons and holes, thereby enhancing the utilization efficiency of photogenerated electrons. This improved efficiency is advantageous for efficient photocatalysis.
In addition, the local electrostatic potential (ESP) and HOMO/LUMO energy levels of BaPP@BDO-Rh molecules were investigated by DFT calculations to explain the electron transfer behavior on the photocatalyst skeleton. On the one hand, the two nitrogen atoms (−4.01 au and −4.08 au) and Rh atoms (−0.019 au) in the bipyridine region of the catalyst molecular framework have large electronegativity, forming a charge accumulation region (blue region, Fig. 3j). The Rh complex is closely bound to two nitrogen atoms of the bipyridine structure by a coordination bond. This region accumulate a large amount of charge, which is easily transferred to the Rh complex, achieving the conversion of NAD+ to NADH. NADH regeneration requires two electrons and a proton.37 On the other hand, the catalyst molecules form a clear spatial separation between the LUMO region and the HOMO region, forming a D–A configuration. Construction of a donor–acceptor (D–A) configuration induces an internal electric field that promotes free hole and electron production, as well as photo-generated carrier separation. Electron binding in the HOMO region is reported to be more relaxed, with the properties of an electron donor.38,39 Electrons in the HOMO region are more easily transferred for NADH regeneration. At the same time, Rh is located in the HOMO region, which is consistent with the traditional understanding that Rh is considered to be the active site for photocatalytic NADH regeneration.36,40–42 The LUMO region has a strong affinity for electrons and has electron acceptor properties.43,44 The LUMO region readily oxidizes electron donors (triethanolamine, water, glucose, etc.), filling the holes in the catalyst and continuously converting NAD+ to NADH.
Optimization of electron generation, transfer and utilization for NADH regeneration
To evaluate the performance of a photocatalyst inspired by natural thylakoids for NADH regeneration, we conducted experiments using BDO, BaPP@BDO, and BaPP@BDO-Rh. The results are shown in Fig. 4a, b and S8.† BaPP@BDO-Rh significantly increased NADH regeneration rate and turnover frequency (TOF) compared with BaPP@BDO with free Rh and BDO with free Rh. This improvement can be attributed to the larger specific surface area of the core–shell structure of BaPP@BDO, which exposes more active sites for capturing light energy (Fig. S9†). Additionally, the ultra-thin shell structure of BaPP@BDO restricts the transport path of photogenerated electrons in a two-dimensional space, thereby enhancing electron transport efficiency. This finding aligns with previous studies that have shown improved efficiency of NADH regeneration with other core–shell photocatalysts.17,32,40 Notably, the NADH regeneration efficiency of BDO-Rh was significantly higher than that of BDO with free Rh. BaPP@BDO-Rh exhibited a maximum TOF of 131.16 h−1 (Fig. 4b). To the best of our knowledge, this is the highest reported level thus far (Table. S2†). The TOF of the optimal BaPP@BDO-Rh (16 nm and 0.188 wt%) is 9.8 times higher than that of BDO and 2.6 times higher than that of BaPP@BDO (Fig. 4a). This phenomenon can be attributed to the Rh catalyst anchored in the BDO framework, which acts as an active site for NADH regeneration. The electron transport distance is shortened, leading to improved electron transport efficiency. Similar phenomena have been reported in previous studies.17,32
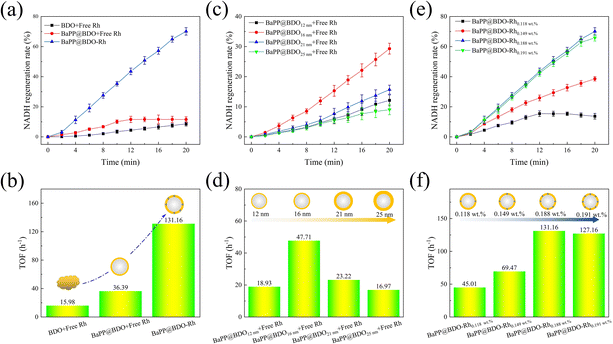 |
| Fig. 4 (a) Yield of NADH as a fucntion of reaction time, (b) the corresponding TOF of BDO with free Rh, BaPP@BDO with free Rh and BaPP@BDO-Rh. (c) Yield of NADH as a fucntion of reaction time, (d) the corresponding TOF of BaPP@BDO12nm with free Rh, BaPP@BDO16nm with free Rh, BaPP@BDO21nm with free Rh and BaPP@BDO15nm with free Rh. (e) Yield of NADH as a fucntion of reaction time, (f) the corresponding TOF of BaPP@BDO-Rh0.115 wt%, BaPP@BDO-Rh0.145 wt%, BaPP@BDO-Rh 0.188 wt%, BaPP@BDO-Rh 0.238 wt%. The TOF for the regeneration of the NADH was calculated based on the reaction time from 4 min to 12 min. Reaction conditions: [photocatalyst] = 1 g L−1; [TEOA/PBS] = 1000/100 mM, pH = 7.5; [NAD+] = 1 mM; [free Rh] = 0.0183 mM; volume = 3 mL. | |
The thickness of the shell has a significant effect on photocatalytic NADH regeneration.41 By controlling the amount of barium sulfate in the synthesis system, we prepared BaPP@BDO catalysts with different thicknesses (Fig. S10†). As shown in Fig. 4c and d, after 12 minutes of light treatment, BaPP@BDO16nm with free Rh exhibited the highest NADH regeneration rate of 15.3% and a TOF of 47.71 h−1. The electron generation and transfer capacity of photocatalysts with different thicknesses initially increased and then decreased, as indicated by changes in resistance and photocurrent (Fig. S10†). Therefore, the synergistic enhancement of photogenerated electron production and transfer ability should be considered as the reason for the improved photocatalytic NADH regeneration efficiency of BaPP@BDO16nm.
Rhodium (Rh) plays a crucial role as a catalytic active site in photocatalytic NADH regeneration. We prepared BDO-Rh catalysts with varying amounts of Rh coordination based on BaPP@BDO16nm. It was observed that the NADH regeneration efficiency of BaPP@BDO-Rh increased with increasing Rh loading. The load of Rh refers to the load on the BDO nanolayer, and the mass of the BDO nanolayer is obtained by thermogravimetric analysis (Fig. S11†). After 12 minutes of irradiation, the NADH regeneration rate reached a maximum of 43.5% for BaPP@BDO-Rh with 0.188 wt% Rh, with a corresponding TOF of 131.16 h−1 (Fig. 4e&f). However, further increasing the Rh loading did not significantly improve the NADH regeneration efficiency, likely due to the saturation of Rh coordination on pyridine. Furthermore, the stability of BaPP@BDO-Rh was evaluated, and it was found that after 6 cycles of use, it still maintained 65% activity (Fig. S12†). Further studies found that the decrease of Rh content was the main reason for the decrease of BaPP@BDO-Rh activity. Rh and bipyridine are connected by coordination interactions, which are weak and unstable. This has become a common core problem facing the entire field of photoenzyme catalysis.
In conventional photocatalytic NADH regeneration systems, triethanolamine (TEOA) is commonly used as an electron donor or sacrificial agent due to its excellent properties.35 However, TEOA is primarily produced through the reaction of toxic ethylene oxide with ammonia, making it unsafe and environmentally unsustainable.42 Additionally, the price of TEOA is relatively expensive (around $1000 per ton for 85% TEOA in the Chinese market). In contrast, glucose can serve as a biologically derived, safe, sustainable, and relatively inexpensive (approximately $500 per ton for 98% glucose in the Chinese market) electron donor.43,44 We have successfully achieved photocatalytic NADH regeneration using glucose as an electron donor. Moreover, BaPP@BDO-Rh (Rh concentration 0.0183 mM) yielded 19.2% NADH after 20 minutes (Fig. S13a†).
Although this value is lower than that achieved with TEOA (70.1% in 20 minutes), the glucose-based regeneration system still holds great potential due to the availability, renewability, and affordability of glucose. HPLC analysis revealed that the main byproducts of glucose oxidation were gluconic acid and formic acid (Fig. S13b†).
We also discovered that BaPP@BDO-Re exhibits the ability to reduce CO2 after substituting Rh with rhenium (Re). When exposed to light, the electrons generated by BDO are transferred to rhenium in the coordination sphere, driving the reduction of CO2 (see Fig. S15a†). In the process of photocatalytic carbon dioxide reduction using Re complex as a co-catalyst, the production of CO in BaPP@BDO-Re (with 4.4% Re content in the coordination sphere) is significantly enhanced compared to BDO and BaPP@BDO (Fig. S14b†). The corresponding rate of CO generation is 92.3 μmol h−1 g−1 for BaPP@BDO-Re, which is 14.9 times higher than that of BDO and 4.7 times higher than that of BaPP@BDO (Fig. S14c†). This further confirms the remarkable photocatalytic activity of the synthesized hydrophilic benzimidazole oligomers photocatalysts.
CO2 is converted to fumarate by a photoenzyme-coupled catalytic system
Fumarate, an unsaturated dicarboxylic acid, has gained attention in the production of environmentally friendly polyester resins, particularly in the synthesis of poly(butene succinate) (PBS) with low environmental impact.29,45,46 In our design, pyruvate and CO2 were utilized as raw materials to sequentially synthesize malate and fumarate through the enzymatic reactions catalyzed by malate dehydrogenase (MaeB) and fumarate hydrase (FumC) (Fig. 5a). To sustain the MaeB reaction, photocatalytic NADH regeneration using BaPP@BDO-Rh was employed. The details of all the reactions are provided in Table S3,† and the standard Gibbs free energy change (ΔG′°) for each enzymatic reaction is listed in Fig. 5b. Despite being thermodynamically unfavorable, with a calculated ΔG′° of 3.89 kJ mol−1 for the overall reaction, the feasibility of this process has been demonstrated.30,31
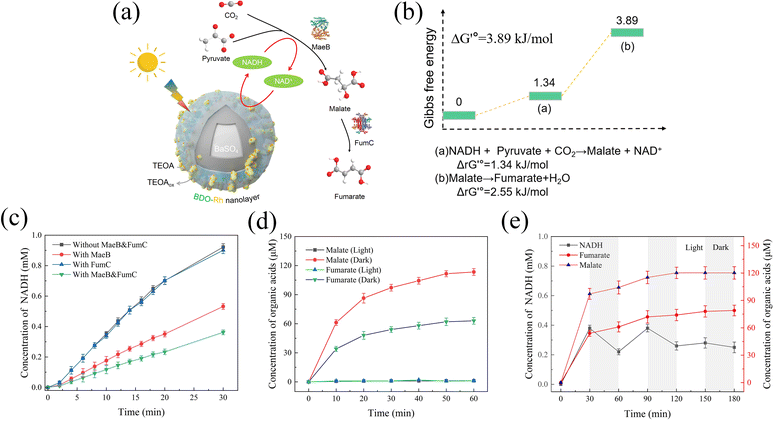 |
| Fig. 5 (a) Scheme of enzyme-photo-coupled catalytic production of organic acid. (b) Standard Gibbs free energy change of each reaction step and the overall process at pH 7.5 and an ionic strength of 0.25 M. (c) Concentration variations of NADH through photocatalysis and MaeB&FumC-photo coupled catalysis. (d) Enzymatic conversion of fumarate in the dark and under light irradiation. (e) Concentration of NADH, malate and fumarate through MaeB & FumC-photo coupled catalysis in the dark and under light irradiation. | |
Firstly, successful expression of malate dehydrogenase (MaeB) from E. coli and fumarate hydrase (FumC) from R. capsulata was achieved (Fig. S15†). The activities of MaeB and FumC were measured to be 1.76 U mg−1 and 6.29 U mg−1, respectively (Table. S4†). Photoenzyme catalysis experiments were conducted using BaPP@BDO-Rh, MaeB, and FumC prepared in this study. As shown in Fig. 5c, NADH is consumed by MaeB to synthesize malic acid. The presence of FumC drives the reaction forward, leading to further consumption of NADH. Malate (114 μM) and fumarate (63 μM) were detected by ion chromatography after 1 hour of illumination under optimal conditions, but were absent under dark conditions (Fig. 5d and S16–S18†). The accumulation of fumarate as an intermediate indicates that the FumC-catalyzed reaction is the rate-limiting step, possibly associated with ΔG′° (Fig. 5b).47,48 Notably, the presence of fumarate and NADH was confirmed by 1H NMR, providing further evidence for the feasibility of this photoenzyme system (Fig. S16†). The relationship between malate and fumarate formation and NADH consumption was analyzed through light–dark cycles. As depicted in Fig. 5e, the formation of NADH, malate, and fumarate exhibits synchronized trends. Even in the dark, malate dehydrogenase can still utilize the photogenerated NADH to achieve the uncoupling of light and dark reactions. The enhanced efficiency of photoenzyme catalysis is closely related to the remarkable NADH regeneration capability of BaPP@BDO-Rh.
Conclusion
In conclusion, we successfully synthesized a highly efficient photocatalyst, BaPP@BDO-Rh, for NADH regeneration. The unique features of its core–shell structure, hydrophilicity, and short crystallization length create a conducive environment for charge carrier movement and enhance the internal electric field, resulting in improved photocatalytic NADH regeneration efficiency. Under optimized conditions, BaPP@BDO-Rh achieved a remarkable 43.5% NADH production within just 12 minutes, with a TOF of 131.16 h−1, the highest reported so far. Moreover, BaPP@BDO-Rh demonstrated synergistic effects with malate dehydrogenase and fumarate hydrase, forming a photoenzyme catalytic system. This system facilitated the synthesis of 79 μM fumarate from CO2 within 3 hour. Notably, this is the first report of a high-performance oligomer photocatalyst in the field of photoenzyme catalysis, opening up new avenues in the realm of sustainable, efficient and high value use of carbon dioxide.
Author contributions
Guohua Li: conceptualization, methodology, formal analysis, investigation, writing – original draft, writing – review & editing, visualization. Zhiwen Lin: methodology, validation, formal analysis, investigation, writing – review & editing, visualization. Xiaodi Li: validation, investigation. Yahui Zhang and Wenlong Zhu: validation, investigation. Yusheng Shao, Qiang Xue and Qunfeng Fan: writing – review & editing. Hui Cao: conceptualization, methodology, resources, writing – review & editing, supervision, project administration, funding acquisition. Tianwei Tan: conceptualization, resources, writing – review & editing, supervision, project, administration, funding acquisition.
Conflicts of interest
The authors declare no conflict of interest.
Acknowledgements
This work was supported by the National Key R&D Program of China [grant numbers 2022YFC2105905] and National Nature Science Foundation of China [grant numbers 22208020]. Thanks to Wei Zhang, Yu Kang, Xin Chang, Jie Cui and other teachers of Beijing University of Chemical Technology for their support in the experimental technology.
Notes and references
- X. Deng, X. Zheng, F. Jia, C. Cao, H. Song, Y. Jiang, Y. Liu, G. Liu, S. Li and L. Wang, Appl. Catal., B, 2023, 330, 122622 CrossRef CAS.
- G. Lin, Y. Zhang, Y. Hua, C. Zhang, C. Jia, D. Ju, C. Yu, P. Li and J. Liu, Angew Chem. Int. Ed. Engl., 2022, 61, e202206283 CrossRef CAS PubMed.
- F. Li, X. Wei, L. Zhang, C. Liu, C. You and Z. Zhu, Angew Chem. Int. Ed. Engl., 2022, 61, e202111054 CrossRef CAS PubMed.
- S. Li, J. Shi, S. Liu, W. Li, Y. Chen, H. Shan, Y. Cheng, H. Wu and Z. Jiang, Chin. J. Catal., 2023, 44, 96–110 CrossRef CAS.
- W. Harrison, X. Huang and H. Zhao, Acc. Chem. Res., 2022, 55, 1087–1096 CrossRef CAS.
- L. Yang, D. Fan, Z. Li, Y. Cheng, X. Yang and T. Zhang, Adv. Sustainable Syst., 2022, 6, 2100477 CrossRef CAS.
- D. I. Arnon, A. Paneque and A. Mitsui, Science, 1961, 134, 1425–1437 CrossRef.
- D. Godde and A. Trebst, Arch. Microbiol., 1980, 127, 245–252 CrossRef CAS.
- Y. Wu, J. Ward-Bond, D. Li, S. Zhang, J. Shi and Z. Jiang, ACS Catal., 2018, 8, 5664–5674 CrossRef CAS.
- S. Zhang, J. Shi, Y. Sun, Y. Wu, Y. Zhang, Z. Cai, Y. Chen, C. You, P. Han and Z. Jiang, ACS Catal., 2019, 9, 3913–3925 CrossRef CAS.
- J. Meng, Y. Tian, C. Li, X. Lin, Z. Wang, L. Sun, Y. Zhou, J. Li, N. Yang, Y. Zong, F. Li, Y. Cao and H. Song, Catal. Sci. Technol., 2019, 9, 1911–1921 RSC.
- S. Zhang, S. Liu, Y. Sun, S. Li, J. Shi and Z. Jiang, Chem. Soc. Rev., 2021, 50, 13449–13466 RSC.
- R. Kathpalia and A. K. Verma, Mater. Today: Proc., 2021, 45, 3825–3832 CAS.
- M. Li, V. Svoboda, G. Davis, D. Kramer, H.-H. Kunz and H. Kirchhoff, Nat. Plants, 2021, 7, 979–988 CrossRef CAS.
- M. Rantala, S. Rantala and E.-M. Aro, Photochem. Photobiol. Sci., 2020, 19, 604–619 CrossRef CAS PubMed.
- X. Wu, S. Wang, J. Fang, H. Chen, H. Liu and R. Li, ACS Appl. Mater. Interfaces, 2022, 14, 38895–38904 CrossRef CAS.
- H. Zhao, L. Wang, G. Liu, Y. Liu, S. Zhang, L. Wang, X. Zheng, L. Zhou, J. Gao, J. Shi and Y. Jiang, ACS Catal., 2023, 13, 6619–6629 CrossRef CAS.
- Z. Cheng, Y. He, C. Yang, N. Meng and Y. Liao, Chin. Chem. Lett., 2022, 34, 107440 CrossRef.
- H. Yang, F. Li, S. Zhan, Y. Liu, W. Li, Q. Meng, A. Kravchenko, T. Liu, Y. Yang, Y. Fang, L. Wang, J. Guan, I. Furó, M. S. G. Ahlquist and L. Sun, Nat. Catal., 2022, 5, 414–429 CrossRef CAS.
- J. Xu, W. Li, W. Liu, J. Jing, K. Zhang, L. Liu, J. Yang, E. Zhu, J. Li and Y. Zhu, Angew Chem. Int. Ed. Engl., 2022, 61, e202212243 CrossRef CAS.
- P. Gao, L. Zhong, B. Han, M. He and Y. Sun, Angew. Chem., Int. Ed., 2022, 61, e202210095 CrossRef CAS PubMed.
- J. QU, W. CHEN, J. ZENG, Y. SUN, Q. LIAO, K. GUO, A. QIN, H. PEI, F. TENG and Y. LIU, Bull. Chin. Acad. Sci., 2022, 37, 444–458 Search PubMed.
- C. Li, R. Wang, J. Wang, L. Liu, H. Li, H. Zheng and J. Ni, Angew Chem. Int. Ed. Engl., 2022, e202215013, DOI:10.1002/anie.202215013.
- J. Yin, Z. Yin, J. Jin, M. Sun, B. Huang, H. Lin, Z. Ma, M. Muzzio, M. Shen, C. Yu, H. Zhang, Y. Peng, P. Xi, C. H. Yan and S. Sun, J. Am. Chem. Soc., 2021, 143, 15335–15343 CrossRef CAS.
- T. Zheng, M. Zhang, L. Wu, S. Guo, X. Liu, J. Zhao, W. Xue, J. Li, C. Liu and X. Li, Nat. Catal., 2022, 5, 388–396 CrossRef CAS.
- P. Wei, H. Ma, H. Fu, Z. Xu and X. Qu, Chemosphere, 2022, 301, 134659 CrossRef CAS.
- L. Zhao, I. M. Khan, B. Wang, L. Yue, Y. Zhang, Z. Wang and W. Xia, Int. J. Food Sci. Technol., 2022, 57, 2872–2878 CrossRef CAS.
- X.-W. Wei, C. Chen, T.-Y. Wu, L.-H. Cai and H.-M. Ye, Molecules, 2022, 27, 7086 CrossRef CAS PubMed.
- Y. Ding, S. Li, J. Wang, Y. Liu, L. Dong, X. Du, D. Huang, T. Ai and J. Ji, J. Appl. Polym. Sci., 2022, 139, e52509 CrossRef CAS.
- M. Takeuchia and Y. Amao, Sustainable Energy Fuels, 2023, 7, 355–359 RSC.
- M. Takeuchi and Y. Amao, React. Chem. Eng., 2022, 7, 1931–1935 RSC.
- S. Li, Y. Cheng, Y. Chen, J. Li, Y. Sun, J. Shi and Z. Jiang, Appl. Catal., B, 2022, 317, 121772 CrossRef CAS.
- J. Li, Y. Zhou, J. Wang, N. Wang, J. Bi, X. Li, K. Chen and H. Hao, Molecules, 2023, 28, 726 CrossRef CAS.
- F. Li, X. Wei, L. Zhang, C. Liu, C. You and Z. Zhu, Angew. Chem., Int. Ed., 2021, 61, e202111054 CrossRef PubMed.
- J. Han, B. Göksel, S. Mohajernia, M. S. Killian, J. Vleugels, A. Braem and S. Castagne, Appl. Surf. Sci., 2023, 156407, DOI:10.1016/j.apsusc.2023.156407.
- Y. Cheng, J. Shi, Y. Wu, X. Wang, Y. Sun, Z. Cai, Y. Chen and Z. Jiang, Research, 2021, 2021, 8175709 CrossRef CAS PubMed.
- B. Huang, X. Tang, Y. Hong, L. Li, T. Hu, K. Yuan and Y. Chen, Angew. Chem., 2023, 135, e202306667 CrossRef.
- Z. Gao, L. Shi, F. Yan, Y. Han, W. Yuan and W. Tian, Angew. Chem., 2023, 135, e202302274 CrossRef.
- C. Wang, L. Ma, S. Wang and G. Zhao, J. Phys. Chem. Lett., 2021, 12, 12129–12134 CrossRef CAS PubMed.
- Y. Tan, J. Ma, F. Zhang, S. Wang, F. Lan, H. Liu and R. Li, ACS Sustain. Chem. Eng., 2022, 10, 12065–12071 CrossRef CAS.
- Y. Sun, J. Shi, Z. Wang, H. Wang, S. Zhang, Y. Wu, H. Wang, S. Li and Z. Jiang, J. Am. Chem. Soc., 2022, 144, 4168–4177 CrossRef CAS PubMed.
- H. Ishaq, R. Foxall and C. Crawford, J. CO2 Util., 2022, 64, 102154 CrossRef CAS.
- X. Bai, Q. Hou, H. Qian, Y. Nie, T. Xia, R. Lai and G. Yu, Appl. Catal., B, 2022, 303, 120895 CrossRef CAS.
- J. Wang, H. Zhao, B. Zhu, S. Larter, S. Cao, J. Yu, M. G. Kibria and J. Hu, ACS Catal., 2021, 11, 12170–12178 CrossRef CAS.
- J. Liu, S. Wang, Y. Peng, J. Zhu, W. Zhao and X. Liu, Prog. Polym. Sci., 2021, 113, 101353 CrossRef CAS.
- V. Hevilla, A. Sonseca, C. Echeverría, A. Muñoz-Bonilla and M. Fernández-García, Macromol. Biosci., 2021, 21, 2100156 CrossRef CAS PubMed.
- S. Tang, D. Liao, X. Li, Y. Lin, S. Han and S. Zheng, ACS Synth. Biol., 2021, 10, 2417–2433 CrossRef CAS.
- Y. Sun, W. Li, Z. Wang, J. Shi and Z. Jiang, Curr. Opin. Biotechnol., 2022, 73, 67–73 CrossRef CAS.
|
This journal is © The Royal Society of Chemistry 2024 |