DOI:
10.1039/D4SU00149D
(Paper)
RSC Sustain., 2024,
2, 2005-2014
Synthesis and characterization of magnetic carbonaceous materials from bamboo waste and investigation of their adsorption capability for cesium ions
Received
26th March 2024
, Accepted 25th April 2024
First published on 26th April 2024
Abstract
In this work, we prepared a series of magnetic carbonaceous materials (CB200F, CB400F, CB600F, CB800F, and CB1000) from waste bamboo (RB) for the removal of cesium ions from water. Physicochemical properties such as pHpzc, specific surface area, and surface functional groups of the prepared adsorbents were evaluated and their cesium ion adsorption capability was also assessed. Consequently, the amount of cesium ions adsorbed was in the following order: CB1000F < RB < CB200F < CB800F < CB600F < CB400F. Additionally, the solid–liquid separation ability of the prepared adsorbent after adsorption was evaluated. The results showed that CB400F, CB600F, and CB800F could be easily separated from the sample solution with the action of the magnetic field under our experimental conditions. Moreover, the effect of parameters, including contact time, adsorption temperature, and pH, on the adsorption of cesium ions was studied. The adsorption of cesium ions was rapid, and the equilibrium was reached within 10 min for CB400F. The adsorption capacity decreased with increasing adsorption temperatures (45 °C < 25 °C < 7 °C), and the optimal pH for removing cesium ions was approximately 10. Finally, the selectivity of cesium ion adsorption using CB400F was assessed; anions including chloride ions, sulfate ions, and nitrate ions did not interfere with the adsorption of cesium ions by CB400F. These results provide valuable information regarding the adsorption of cesium ions using the CB400F from aqueous media. The suggested treatment in this study significantly contributes to the development of recycling technology for biomass waste and/or purification of wastewater containing cesium ions.
Sustainability spotlight
In 2015, all member states of the United Nations embraced the sustainable development goals as a component of the 2030 agenda for sustainable development. This signifies that diverse initiatives have been implemented to establish a comprehensive 15 year strategy to attain these objectives. Particularly, goal 6 and goal 12 aim to develop efficient recycling technologies for establishing a sustainable society and reducing waste globally. Therefore, we focused on both the recycling technology of waste biomass bamboo (raw bamboo, RB) and wastewater purification to prevent clogging in adsorption treatment with adsorbent to establish a sustainable society and reduce global waste.
|
1 Introduction
In Japan, the Fukushima Daiichi Nuclear Power Plant accident occurred in 2011 and it led to widespread radiation release. Thus, it aroused the attention of many researchers towards finding a swift and sustainable technology for the decontamination of the radioactivity in the surrounding environment.1–3 The accident was one of the worst nuclear accidents in nuclear history due to the release of certain nuclides, notably cesium, such as 137Cs and 134Cs, into the water environment. The 137Cs level in Japan was approximately 1–2 × 10−3 Bq L−1 before 2011.4 However, after 2011 in Japan, the levels of 137Cs and 134Cs increased to approximately 1.5 × 1013 Bq L−1 and approximately 1.8 × 1013 Bq L−1.5 These isotopes are beta and gamma emission nuclides with a half-life of approximately 2 and 30 years, respectively. Cesium nuclides have the potential to enter and accumulate within the human body through the consumption of contaminated food and/or drinking water.6–9 Consequently, these can contribute to the development of various diseases including thyroid cancer.10 In addition, cesium nuclides can crush the biological cells of many organs and affect severe genetic disorders.11–13 The release of cesium nuclides into the water environment also resulted in soil contamination and restricted the advancement of agriculture and animal tillage.14–18 Therefore, according to the regulations of the International Atomic Energy Agency, low-rate radioactive wastewaters are defined as solutions with total radioactivity below 4.0 × 106 Bq L−1.19
Various treatments have been proposed to prevent the spread of radionuclides in the biosphere, especially in water environments, such as membrane separation, evaporation, adsorption, reverse osmosis, ion exchange, precipitation, and filtration.19 Among these treatments, adsorption is an easy, inexpensive, and more effective method, which is generally selected to adsorb radioactive waste from water environments.20–23
Furthermore, in 2015, all member states of the United Nations embraced the sustainable development goals as a component of the 2030 agenda for sustainable development. This signifies that diverse initiatives have been implemented to establish a comprehensive 15 year strategy to attain these objectives. Particularly, Goal 6 (clean water and sanitation) and Goal 12 (responsible consumption and production) aim to develop efficient recycling technologies for establishing a sustainable society and reducing waste globally.24 One of these technologies changes waste biomass into valuable material, such as an adsorbent. In previous literature, activated charcoal, including various modified activated carbons, was prepared from waste biomass, and its characteristics and adsorption capability of cesium ions from the aqueous phase were evaluated.25–28
Bamboo is abundant in nature and is a renewable waste biomass, mostly found in Southeast Asian countries, including Thailand. A previous study reported that the physicochemical treatment of bamboo materials can enhance the adsorption capability of the adsorbate.29,30 Additionally, the calcination of bamboo has gained attention as an adsorbent due to its enrichment with a microporous structure and higher surface area.31 In a separate study, another researcher reported a simple separation method toward the use of magnetically activated carbon for preventing clogging in the water purification process.32,33 Therefore, we focused on both the recycling technology of waste biomass bamboo (raw bamboo, RB) and wastewater purification to prevent clogging during the adsorption treatment with the adsorbent to establish a sustainable society and reduce global waste.
Based on the current understanding, there is a lack of research on the utilization of magnetically-activated charcoal derived from waste biomass bamboo as an adsorbent for removing cesium ions, despite its significant potential. Therefore, the magnetically activated charcoal was prepared from RB, and its characteristics including pHpzc, specific surface area, and surface functional groups were investigated. Additionally, the adsorption capability of cesium ions from aqueous solution under several experimental conditions such as contact time, adsorption temperature, solution pH, and the effect of coexistences using the prepared adsorbent was also addressed in detail. Finally, the obtained results in this study contribute to the development of recycling technology for biomass waste and/or purification of wastewater containing cesium ions.
2 Experimental section
2.1 Materials
RB was obtained from Thailand. Cesium standard solution (CsCl in water), hydrochloric acid (HCl), sodium hydroxide (NaOH), and iron(III) nitrate nonahydrate (Fe(NO3)3·9H2O) were supplied from FUJIFILM Wako Pure Chemical Co., Ltd (Japan). Potassium chloride, sodium chloride, sodium sulfate, and sodium nitrate were also purchased from FUJIFILM Wako Pure Chemical Ind., Ltd (Special grade reagent, Japan).
Calcined bamboo treated with iron(III) nitrate nonahydrate at different temperatures was prepared using the following method: RB and iron(III) nitrate nonahydrate were mixed at an RB/Fe(NO3)3·9H2O mass ratio of 1
:
1.5 in a crucible. Then, the mixtures were calcined at 200 °C, 400 °C, 600 °C, 800 °C, or 1000 °C for 2 h by KDF S-80 instrument (Denken-Highdental Co., Ltd, Japan) and the calcination temperature was held for 2 h, and then, the obtained materials were naturally cooled in this study; the samples were labeled as CB200F, CB400F, CB600F, CB800F, and CB1000F, respectively.
2.2 Characteristics of raw bamboo (RB) and prepared samples
The physical and chemical characteristics of both RB and the prepared samples were evaluated using the following methods: Scanning Electron Microscope (SEM) images were acquired using an SU1510 instrument from Hitachi High-Technologies Co., Ltd, Japan. Fourier-transform infrared (FT-IR) spectroscopy was also measured using an FT-IR-460Plus spectrometer (JASCO, Co., Japan). The specific surface area was determined using a NOVA4200e instrument (Yuasa Ionics, Japan). The point of zero charge (pHpzc) and surface functional groups, including acidic or basic functional groups, were also determined following the methods as detailed in prior studies by Faria et al.34,35 and Boehm's titration method,36,37 respectively. Finally, X-ray diffraction (XRD) patterns of CB400F and Fe3O4 were measured using a MiniFlex II instrument (Rigaku, Japan).
2.3 Adsorption capacity of cesium ions
In this study, an initial screening experiment was carried out to evaluate the adsorption capacity of cesium ions using RB and prepared samples. Cesium ion solution (50 mg L−1, 50 mL) and each adsorbent (0.05 g) were mixed and reacted at 100 rpm for 24 h at 25 °C. After the completion of the reaction, the solution was filtered through a 0.45 μm membrane filter. The equilibrium concentration of cesium ions was subsequently quantified using an inductively coupled plasma optical emission spectrometer (iCAP-7600 Duo, Thermo Fisher Scientific Inc., Japan). Finally, the quantity of cesium ions adsorbed was calculated by the differences between initial and equilibrium concentrations.
2.4 Changes in the adsorption capacity of cesium ions under several experimental conditions
The assessment of adsorption isotherms was conducted as follows: cesium ions solutions at different concentrations (10 mg L−1, 20 mg L−1, 30 mg L−1, 40 mg L−1, or 50 mg L−1, 50 mL) were mixed with 0.05 g of CB400F and then reacted at 100 rpm for 24 h at 7 °C, 25 °C, and 45 °C. For analyzing adsorption isotherm models, typically, Langmuir (eqn (1)) and Freundlich (eqn (2)) isotherms were chosen.38,39 The following equation presents the linearized expression of Langmuir's isotherm model: | 1/q = 1/(qmaxKLCe) + 1/qmax | (1) |
where q is the quantity of cesium ions adsorbed (mg g−1), qmax is the maximum quantity adsorbed (mg g−1), Ce is the equilibrium concentration (mg L−1), and KL is the Langmuir isotherm constant (binding energy) (L mg−1).
Moreover, the linear form of Freundlich's isotherm model is:
| 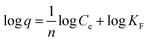 | (2) |
where
KF and 1/
n are the adsorption capacity and strength of adsorption, respectively.
Next, the effect of contact time on the adsorption of cesium ions was measured; cesium ion solution at 50 mg L−1 (50 mL) and CB400F of 0.05 g was mixed and reacted at 100 rpm for 10 min, 1 h, 6 h, 9 h, 15 h, 18 h, 21 h, and 24 h at 25 °C. Adsorption kinetics were assessed by the pseudo-first-order (PFOM, eqn (3)) and pseudo-second-order kinetic models (PSOM, eqn (4)).40,41
| ln(qe − qt) = ln qe − k1t | (3) |
| 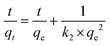 | (4) |
where
qe (mg g
−1) is the quantity of the adsorbed cesium ions at equilibrium,
qt (mg g
−1) is the quantity of cesium ions adsorbed at time
t,
k1 (h
−1) is the overall constant in the pseudo-first-order model, and
k2 (g mg
−1 h
−1) is the pseudo-second-order adsorption constant.
Finally, 50 mg L−1 cesium ion solution at different pH (pH 2, 4, 6, 8, 10, or 12 and 50 mL) and CB400F of 0.05 g was mixed and reacted at 100 rpm for 24 h at 25 °C. The quantity of the adsorbed cesium ions was calculated using the method described in Section 2.3. Moreover, electron probe microanalysis was performed both before and after the adsorption of cesium ions using a JXA-8530F microanalyzer (JEOL, Japan).
2.5 Effect of coexistences on the adsorption characteristics of cesium ions
In order to investigate the adsorption selectivity of cesium ions in an aqueous phase, a complex solution system (50 mL) was prepared by adding 0.05 g of CB400F. Potassium (potassium chloride) and sodium (sodium chloride) ions were used as the interfering cations. Meanwhile, chloride (sodium chloride), sulfate (sodium sulfate), and nitrate (sodium nitrate) ions were used as anions in this study. The initial concentration of each anion and cations was set at 50 mg L−1. The binary solution systems (cesium ion + cation or anion) were shaken for 24 h at 100 rpm and 25 °C. After the adsorption, the sample solution underwent separation through a 0.45 μm membrane filter. The concentration of each cation was measured using the iCAP-7600 Duo instrument, while the concentration of each anion was measured using a DIONEX ICS-900 (Thermo Fisher Scientific Inc., Tokyo, Japan) following the previously reported experimental conditions.42
2.6 Recycling performance of CB400F for removal of cesium ions
To elucidate the recycling performance of CB400F, the adsorption/desorption capability of cesium ions was demonstrated. First, 100 mg L−1 cesium ion solution (50 mL) and CB400F of 0.1 g were mixed and reacted at 100 rpm for 24 h at 25 °C. The quantity of cesium ions adsorbed was also calculated using the method described in Section 2.3. After adsorption, CB400F was collected, dried at 50 °C, and used for desorption experiments. The desorption experiments were performed according to the following methods. Collected CB400F (0.05 g) was mixed with a desorption solution such as distilled water, 1 mmol L−1 sodium hydroxide solution, or 1 mmol L−1 hydrochloric acid solution (50 mL). The reaction solution was shaken at 100 rpm for 24 h at 25 °C. After desorption, the solution was then filtered through a 0.45 μm membrane filter. Subsequently, the concentration of cesium ions was measured using the iCAP-7600 Duo instrument. The desorption capacity was calculated using the equilibrium concentrations.
3 Results and discussion
3.1 Characteristics of RB and magnetic carbonaceous material (CB) adsorbents
The SEM images of RB and the prepared CB samples are presented in Fig. 1. The RB surface exhibited a dense and constricted morphology; compared to CB samples, the number of pores on the CB surface increased with increasing calcination temperatures. In particular, the CB400F, CB600F, and CB800F models were composed of porous material with a rougher surface and looser structure. Furthermore, in Fig. 2, the FT-IR spectra of the prepared samples are presented. Some CB samples showed a broad and intense band within the range of 3200–3600 cm−1 due to the presence of free and hydrogen-bonded OH stretching vibrations. Additionally, a prominent peak in the spectral range of 1500–1700 cm−1 was observed, indicating the presence of C–O carbonyl stretching vibrations. In Table 1, the pHpzc, specific surface area, and the amount of acid or basic functional groups of CB samples are presented. The value of pHpzc increased from 5.4 to 9.7 with increasing calcination temperatures, and a similar trend was observed in the value of specific surface area. Furthermore, the values of CB400F, CB600F, and CB800F were greater than those of other samples. Finally, the amount of acid or basic functional groups of RB was 0 or 0.81 mmol g−1, respectively, and the amount of basic functional groups decreased with increasing calcination temperatures. These changes were consistent with the results of FT-IRs.
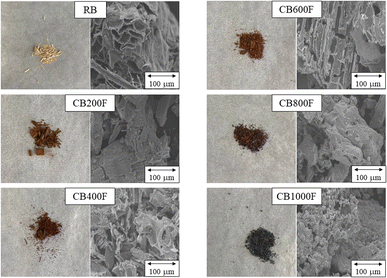 |
| Fig. 1 SEM images of the prepared samples. | |
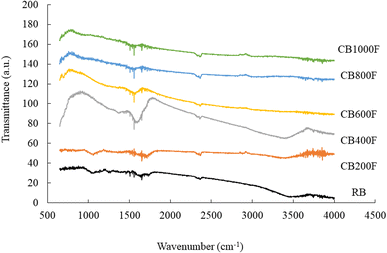 |
| Fig. 2 FT-IR spectra of the prepared samples. | |
Table 1 Characteristics of the prepared samples
Samples |
RB |
CB200F |
CB400F |
CB600F |
CB800F |
CB1000F |
pHpzc |
5.4 |
5.1 |
7.8 |
8.8 |
9.9 |
9.7 |
Specific surface area (m2 g−1) |
3.3 |
14.0 |
50.6 |
50.5 |
56.9 |
24.1 |
Amount of acid functional groups (mmol g−1) |
0 |
0 |
0.10 |
0.07 |
0.13 |
0.02 |
Amount of basic functional groups (mmol g−1) |
0.81 |
0.87 |
0.83 |
0.08 |
0.11 |
0 |
3.2 Amount of cesium ions adsorbed using RB and CB samples
Fig. 3 shows the adsorption capacity of cesium ions. Under our experimental conditions, the amount adsorbed followed this order: CB1000F (0.08 mg g−1) exhibited the lowest adsorption, followed by RB (0.1 mg g−1), CB200F (0.4 mg g−1), CB800F (0.8 mg g−1), CB600F (10.1 mg g−1), and CB400F (12.8 mg g−1). The characteristics of both RB and CB samples suggest that the specific surface area plays a significant role in enhancing the adsorption capacity of cesium ions in the aqueous phase. Nevertheless, additional research is imperative to comprehensively understand the adsorption mechanism of cesium ions when employing these prepared samples. Recently, some studies have focused on a separation method toward the use of magnetic carbon for preventing clogging during the water purification process.32,33 Thus, the efficiency of solid–liquid separation using CB samples was evaluated in this study. Fig. 4 shows the effective separation of CB400F, CB600F, and CB800F from the sample solution due to the action of the magnetic field, suggesting the potential utility of prepared CB samples in water purification for solid–liquid separation. In addition, the existence of Fe3O4 in CB400F was measured by XRD patterns (Fig. 5). As a result, the peaks of Fe3O4 were clearly detected in CB400F. Therefore, CB400F showed the efficiency of solid–liquid separation under our experimental conditions. These data support the abovementioned results and discussion.
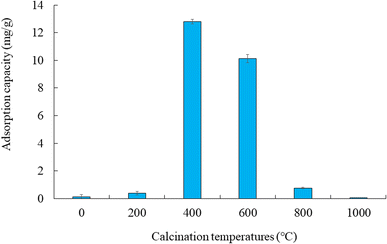 |
| Fig. 3 Adsorption capacity of cesium ions. Initial concentration: 50 mg L−1, sample volume: 50 mL, adsorbent: 0.05 g, temperature: 25 °C, contact time: 24 h, 100 rpm. | |
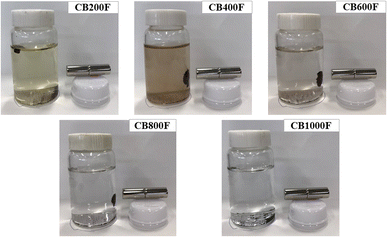 |
| Fig. 4 Photographs of sample solutions after adsorption. | |
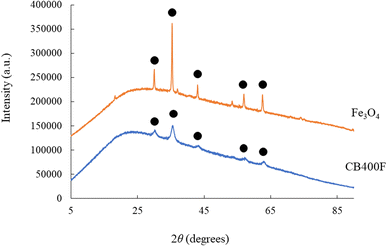 |
| Fig. 5 XRD patterns of CB400F and Fe3O4. | |
3.3 Effect of contact time on the adsorption of cesium ions using CB400F
The time course for the adsorption of cesium ions is shown in Fig. 6, it indicates a rapid adsorption rate with equilibrium achieved within 10 min for CB400F. This rapid cesium ion adsorption can be explained by the availability of active sites on the adsorbent surface, which indicates that rapid initial adsorption (within 9 h) was likely due to the outer surface binding, while slower adsorption (after 9 h) was likely due to inner surface binding.43,44
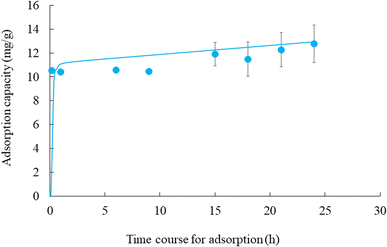 |
| Fig. 6 Time course of adsorption of cesium ions. Initial concentration: 50 mg L−1, sample volume: 50 mL, adsorbent: 0.05 g, temperature: 25 °C, contact time: 10 min, 1 h, 6 h, 9 h, 15 h, 18 h, 21 h, and 24 h, 100 rpm. | |
Additionally, PFOM and PSOM were used to comprehend the adsorption mechanism of cesium ions. Among the two applied kinetic models, the PFOM model appears unsuitable for describing the adsorption of cesium ions with CB400F (Table 2). According to this model, the correlation coefficient was not high (0.752), and the theoretical value of adsorption at equilibrium (12.8 mg g−1) is significantly different from the experimental value (2.8 mg g−1). In contrast, under our experimental conditions, the PSOM exhibits a higher correlation coefficient of 0.992. Additionally, the adsorption capacity of cesium ions obtained from this kinetic model is closely aligned with the experimental value (12.6 mg g−1). Therefore, these results indicate that the pseudo-second-order adsorption mechanism could explain the adsorption of cesium ions by CB400F.
Table 2 Fitting results of kinetic data using PFOM and PSOM
Sample |
q
e,exp (mg g−1) |
Pseudo-first-order model |
Pseudo-second-order model |
K
1 (h−1) |
q
e,cal (mg g−1) |
R
2
|
K
2 (mg g−1 h−1) |
q
e,cal (mg g−1) |
R
2
|
CB400F |
12.8 |
0.07 |
2.8 |
0.752 |
0.08 |
12.6 |
0.992 |
3.4 Effect of initial concentration and temperature on the adsorption of cesium ions using CB400F
Fig. 7 shows the adsorption isotherms of cesium ions at different temperatures. The amount adsorbed increased with decreasing adsorption temperatures (45 °C < 25 °C < 7 °C). These results demonstrate that the adsorption process of cesium ions is exothermic. The adsorption isotherms parameters are presented in Table 3; the data clearly show that the Langmuir model (correlation coefficient: 0.906 to 0.929) is more appropriate than the Freundlich model (correlation coefficient: 0.752 to 0.939) for the adsorption of cesium ions. Moreover, the Langmuir adsorption isotherm proves to be a valuable tool for calculating the maximum quantity of cesium ion adsorption. The maximum amount quantity (qmas) increased with decreasing adsorption temperatures [45 °C (11.0 mg g−1) < 7 °C (20.7 mg g−1)]; these phenomena showed similar trends in the adsorption isotherms (Fig. 6). Finally, cesium ions were easily adsorbed onto the CB400F surface when 1/n was in the 0.1–0.5 range.45 Conversely, when 1/n was >2, cesium ions did not easily adsorb onto the surface of CB400F. In this study, the value of 1/n was 0.4–0.8, indicating that cesium ions were easily adsorbed onto CB400F under our experimental conditions.
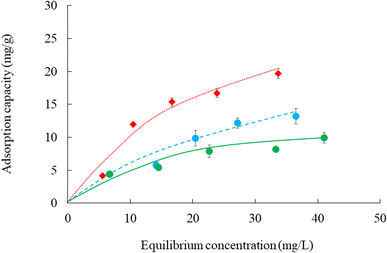 |
| Fig. 7 Adsorption isotherms of cesium ions at different temperatures. Initial concentration: 10–50 mg L−1, sample volume: 50 mL, adsorbent: 0.05 g, temperature: 7 ( ), 25 ( ), and 45 ( ) °C, contact time: 24 h, 100 rpm. | |
Table 3 Fitting results of isotherm data using Freundlich and Langmuir models
Sample |
Temperature (°C) |
Langmuir isotherm model |
Freundlich isotherm model |
K
L (L mg−1) |
q
max (mg g−1) |
R
2
|
log KF |
1/n |
R
2
|
CB400F |
5 |
5.3 |
20.7 |
0.929 |
0.1 |
0.8 |
0.870 |
25 |
26.6 |
21.1 |
0.906 |
0.03 |
0.7 |
0.939 |
45 |
10.8 |
11.0 |
0.908 |
0.3 |
0.4 |
0.752 |
To further confirm the adsorption of cesium ions onto CB400F surface, the elemental distribution of cesium (Cs) onto CB400F before and after the adsorption was evaluated (Fig. 8). Based on the results, the amount of cesium (Cs) increased after the adsorption treatment. This indicates that cesium ions were adsorbed onto the CB400F surface and supports the adsorption phenomenon mentioned above in this experiment.
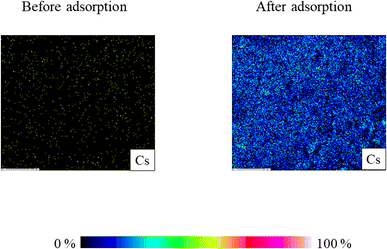 |
| Fig. 8 Elemental distributions of cesium (Cs) before and after adsorption. Initial concentration: 50 mg L−1, sample volume: 50 mL, adsorbent: 0.05 g, temperature: 25 °C, contact time: 24 h, 100 rpm. | |
Finally, many studies have successfully been able to remove cesium ions from the aqueous phase using various types of biomass adsorbents,46–51 and these were compared with this study (Table 4). This demonstrated that CB400F exhibited superior performance in removing cesium ions from the aqueous phase (except for nitric acid-modified bamboo charcoal and Mucilaginous seeds of Ocimum basulicum).
Table 4 Comparison of the adsorption capacity of cesium ions with other reported adsorbents
Adsorbents |
Adsorption capability (mg g−1) |
pH |
Temperature (°C) |
Initial concentration (mg L−1) |
Contact time (h) |
Adsorbent (g L−1) |
References |
Coal and chitosan |
3.0 |
— |
Ambient |
3.9 |
24 |
5–10 |
46
|
Coconut shell-activated carbon |
0.76 |
7.3–10.0 |
— |
10–30 |
24 |
0.67–3.3 |
47
|
Bamboo charcoal |
0.17 |
4.0 |
20 |
100 |
6 |
1.0 |
48
|
Nitric acid-modified bamboo charcoal |
45.87 |
4.0 |
20 |
100 |
6 |
1.0 |
48
|
Brewery's waste biomass |
10.1 |
4.0 |
30 |
1064 |
3 |
2 |
49
|
Pre-treated arca shell biomass |
3.93 |
1–7 |
20 ± 2 |
10–500 |
0.17–7 |
0.1–15.0 |
50
|
Mucilaginous seeds of Ocimum basulicum |
160 |
— |
28 |
10 000 |
2 |
25 |
51
|
CB400F |
12.8 |
— |
25 |
50 |
24 |
1.0 |
This study |
3.5 Effect of pH on the adsorption of cesium ions using CB400F
Fig. 9 shows the adsorption capacity of cesium ions at different pH conditions. The study found that higher cesium ion adsorption was achieved at pH 10 compared to other pH conditions, and adsorption was seriously interrupted at lower pH (<4). There are two underlying reasons contributing to this phenomenon: the competition of H3O+ with cesium ions, as well as the electrostatic repulsion between cesium ions and the positively charged surface of CB400F (the value of pHpzc is 7.8). Similar adsorption mechanisms have already been reported in previous studies.52,53 Conversely, it is assumed that under basic conditions, the adsorption of cesium ions was also significantly influenced under an alkaline state (>10), possibly due to the formation of cesium hydroxide. Furthermore, the presence of negative ions in cesium hydroxide (Cs(OH)2−) might increase an electrostatic repulsion among hydroxide ions or the negatively charged surface of CB400F, inhibiting cesium ion adsorption.53,54
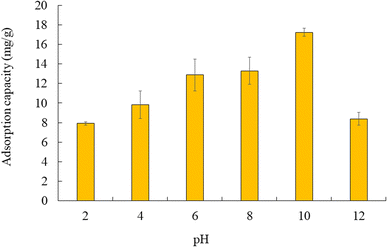 |
| Fig. 9 Adsorption capacity of cesium ions at different pH conditions. Initial concentration: 50 mg L−1, sample volume: 50 mL, adsorbent: 0.05 g, temperature: 25 °C, contact time: 24 h, 100 rpm. | |
3.6 Effect of coexistences on the adsorption of cesium ions
Fig. 10 shows the effect of coexistence on the adsorption of cesium ions. The amount of adsorbed cesium ions onto CB400F in a single solution system was 12.8 mg g−1, while the amount adsorbed in a cation complex solution system clearly decreased. Furthermore, the amount of cesium ions adsorbed in an anion complex solution system was significantly unchanged compared to the single solution system. These phenomena indicate that anions, including chloride ions, sulfate ions, and nitrate ions, have a limited impact on the adsorption capacity of cesium ions using CB400F. Consequently, CB400F exhibits potential utility as an effective adsorbent for the removal of cesium ions from aqueous solutions.
 |
| Fig. 10 Effect of coexistence on the adsorption of cesium ions. Initial concentration: 50 mg L−1, sample volume: 50 mL, adsorbent: 0.05 g, temperature: 25 °C, contact time: 24 h, 100 rpm. | |
3.7 Cesium ions adsorption/desorption capacity of CB400F
CB400F's capacity for desorption of the adsorbed cesium ions was assessed using various desorption solutions such as distilled water, 1 mmol L−1 sodium hydroxide solution, or 1 mmol L−1 hydrochloric acid solution. The desorption percentages of cesium ions from CB400F using distilled water, 1 mmol L−1 sodium hydroxide solution, or 1 mmol L−1 hydrochloric acid solution were 0%, 48.5%, or 0%, respectively. The optimal pH condition for the removal of cesium ions was approximately 10 in Section 3.5. Therefore, adsorbed cesium ions were easily desorbed under acidic conditions. The results show that hydrochloric acid solution is the suitable solution for the desorption of cesium ions from CB400F under the explored experimental conditions.
3.8 Adsorption mechanism of cesium ions using CB400F
Previous studies have reported the adsorption mechanism between an adsorbent and heavy metal in detail.55,56 Therefore, we briefly summarized the schematic mechanism of cesium ions adsorption using CB400F (Fig. 11). The adsorption mechanisms can mainly be divided into two processes in this study. One is cesium ions are adsorbed by the CB400F surface due to the surface area and/or surface charge (physisorption). Another is electrostatic attraction occurring between the surface functional groups and cesium ions (chemisorption). However, further studies are necessary to elucidate the adsorption mechanism of cesium ions in detail using CB400F.
 |
| Fig. 11 The schematic mechanism of cesium ions adsorption using CB400F. | |
4. Conclusions
In this study, magnetic carbonaceous materials derived from waste bamboo were prepared, and the findings demonstrate that the adsorption capability of cesium ions using CB400F (12.8 mg g−1) was higher compared to other prepared adsorbents (0.08–10.1 mg g−1). Additionally, CB400 exhibited superior performance in achieving solid–liquid separation during water purification. The optimization of the factors, including contact time (within 10 min), temperature (45 °C < 25 °C < 7 °C), and pH (approximately 10), on cesium ion adsorption using CB400 was determined. In addition, the adsorption process followed the pseudo-second-order model (correlation coefficient: 0.992). The experimental adsorption data are well-fitted by the Langmuir model (correlation coefficient: 0.906–0.929) compared to the Freundlich model (correlation coefficient: 0.752–0.939). To confirm the adsorption of cesium ions onto the CB400F surface, the elemental distribution of Cs onto the CB400 surface was evaluated before and after the adsorption treatment. The intensity of Cs was observed to exhibit an increase following the adsorption process in comparison to its initial state. Finally, anions, including chloride ions, sulfate ions, and nitrate ions, exhibited limited influence on the adsorption capacity of cesium ions using CB400F. Consequently, CB400F demonstrates promise as a valuable adsorbent for the removal of cesium ions from aqueous solutions.
Author contributions
Fumihiko Ogata: conceptualization, project administration, writing–original draft, and writing–review and editing; Noriaki Nagai: investigation and visualization; Taiki Ogawa: investigation and visualization; Yugo Uematsu: investigation and visualization; Chalermpong Saenjum: investigation and visualization; Shigeharu Tanei: methodology; Naohito Kawasaki; project administration, supervision, and writing–review and editing.
Conflicts of interest
There are no conflicts to declare.
Acknowledgements
This work is supported by JSPS KAKENHI (JP22K06674).
References
- M. R. Awual, Ring size dependent crown ether based mesoporous adsorbent for high cesium adsorption from wastewater, Chem. Eng. J., 2016, 303, 539–546 Search PubMed.
- M. R. Awual, M. Miyazaki, T. Taguchi, H. Shiwaku and T. Yaita, Encapsulation of cesium from contaminated water with highly selective facial organic-inorganic mesoporous hybrid adsorbent, Chem. Eng. J., 2016, 291, 128–137 Search PubMed.
- M. R. Awual, T. Yaita, M. Miyazaki, D. Matsumura, H. Shiwaku and T. Taguchi, A reliable hybrid adsorbent for efficient radioactive cesium accumulation from contaminated wastewater, Sci. Rep., 2016, 6, 19937 Search PubMed.
- M. Aoyama and K. Hirose, Artificial radionuclides database in the Pacific Ocean: HAM database, Sci. World J., 2004, 4, 200–215 Search PubMed.
- D. Parajuli, H. Tanaka, Y. Hakuta, K. Minami, S. Fukuda, K. Umeoka, R. Kamimura, Y. Hayashi, M. Ouchi and T. Kawamoto, Dealing with the aftermath of Fukushima Daiichi nuclear accident: Decontamination of radioactive cesium enriched ash, Environ. Sci. Technol., 2013, 47, 3800–3806 Search PubMed.
- M. R. Awual, T. Yaita, T. Taguchi, H. Shiwaku, S. Suzuki and Y. Okamoto, Selective cesium removal from radioactive liquid waste by crown ether immobilized new class conjugate adsorbent, J. Hazard. Mater., 2014, 278, 227–235 Search PubMed.
- T. J. Yasunari, A. Stohl, R. S. Hayano, J. F. Burkhart, S. Eckhardt and T. Yasunari, Cesium-137 deposition and contamination of Japanese soils due to the Fukushima nuclear accident, Proc. Natl. Acad. Sci. U. S. A., 2011, 108, 19530–19534 Search PubMed.
- R. Chen, H. Tanaka, T. Kawamoto, M. Asai, C. Fukushima, M. Kurihara, M. Ishizaki, M. Watanabe, M. Arisaka and T. Nankawa, Thermodynamics and mechanism studies on electrochemical removal of cesium ions from aqueous solution using a nanoparticle film of copper hexacyanoferrate, ACS Appl. Mater. Interfaces, 2013, 24, 12984–12990 Search PubMed.
- H. Long, P. Wu, L. Yang, Z. Huang, N. Zhu and Z. Hu, Efficient removal of cesium from aqueous solution with vermiculite of enhanced adsorption property through surface modification by ethylamine, J. Colloid Interface Sci., 2014, 428, 295–301 Search PubMed.
- H. Yang, H. Yu, J. Sun, J. Liu, J. Xia, J. Fang, Y. Li, F. Qu, A. Song and T. Wu, Facile synthesis of mesoporous magnetic AMP polyhedric composites for rapid and highly efficient separation of Cs+ from water, Chem. Eng. J., 2017, 317, 533–543 Search PubMed.
- V. J. Sharavanan, M. Sivaramakrishnan, N. Sivarajasekar, N. Senthilrani, R. Kothandan, N. Dhakal, S. Sivamani, P. L. Show, M. R. Awual and M. Naushad, Pollutants inducing epigenetic changes and diseases, Environ. Chem. Lett., 2020, 18, 325–343 Search PubMed.
- H. Yang, H. Li, J. Zhai, L. Sun, Y. Zhao and H. Yu, Magnetic prussian blue/graphene oxide nanocomposites caged in calcium alginate microbeads for elimination of cesium ions from water and soil, Chem. Eng. J., 2014, 246, 10–19 Search PubMed.
- B. Hu, B. Fugetsu, H. Yu and Y. Abe, Prussian blue caged in spongiform adsorbents using diatomite and carbon nanotubes for elimination of cesium, J. Hazard. Mater., 2012, 217–218, 85–91 Search PubMed.
- C. W. Park, B. H. Kim, H. M. Yang, B. K. Seo, J. K. Moon and K. W. Lee, Removal of cesium ions from clays by cationic surfactant intercalation, Chemosphere, 2017, 168, 1068–1074 Search PubMed.
- I. Lee, C. W. Park, S. S. Yoon and H. M. Yang, Facile synthesis of copper ferrocyanide-embedded magnetic hydrogel beads for the enhanced removal of cesium from water, Chemosphere, 2019, 224, 776–785 Search PubMed.
- S. R. H. Vanderheyden, J. Yperman, R. Carleer and S. Schreurs, Enhanced cesium removal from real matrices by nickel-hexacyanoferrate modified activated carbons, Chemosphere, 2018, 202, 569–575 Search PubMed.
- A. Zehad, G. J. Islam, M. Rashid, N. J. Juthy and S. Zannah, Antidiabetic and antihyperlipidemic activities of methanolic leaf extract of stephania japonica in alloxan induced diabetic rats, Pharmacol. Pharm., 2017, 8, 109–127 Search PubMed.
- S. Zannah, M. Asaduzzaman, A. A. A. Bari, Y. Ali, G. J. Islam, A. H. M. K. Khurshid Alam, H. Ali and M. Rashid, Antidiabetic drugs in combination with hydroxychloroquine improve glycemic control in alloxan induced diabetic rats, Pharmacol. Pharm., 2014, 5, 725–735 Search PubMed.
- B. Işık, A. E. Kurtoğlu, G. Gürdağ and G. Keçeli, Radioactive cesium ion removal from wastewater using polymer metal oxide composites, J. Hazard. Mater., 2021, 403, 123652 Search PubMed.
- A. S. Bhatt, D. K. Bhat and M. S. Santosh, Electrical and magnetic properties of chitosan-magnetite nanocomposites, Phys. B, 2010, 405, 2078–2082 Search PubMed.
-
N. A. Chapman and I. G. McKinley, The Geological Disposal of Nuclear Waste, Wiley-Interscience, New York, 1987, p. 49 Search PubMed.
- B. Li, H. Liu, X. Ye, S. Li and Z. Wu, Rubidium and cesium ion adsorption by a potassium titanium silicate-calcium alginate composite adsorbent, Sep. Sci. Technol., 2014, 49, 1076–1085 Search PubMed.
- Ş. Sert and M. Eral, Uranium adsorption studies on aminopropyl modified mesoporous sorbent (NH2 – MCM – 41) using statistical design method, J. Nucl. Mater., 2010, 406, 285–292 Search PubMed.
- Y. Uematsu, F. Ogata, N. Nagai, S. Chalermpong, T. Nakamura and N. Kawasaki, In vitro removal of paraquat and diquat from aqueous media using raw and calcined basil seed, Heliyon, 2021, 7, e07644 Search PubMed.
- S. R. H. Vanderheyden, R. Van Ammel, K. Sobiech-Mature, K. Vanerppelen, S. Schreurs, W. Schroeyers, J. Yperman and R. Carleer, Adsorption of cesium on different types of activated carbon, J. Radioanal. Nucl. Chem., 2016, 310, 301–310 Search PubMed.
- S. Khandarker, M. F. Chowdhury, Md. R. Awual, A. Islam and T. Kuba, Efficient cesium encapsulation from contaminated water by cellulosic biomass based activated wood charcoal, Chemosphere, 2021, 262, 127801 Search PubMed.
- X. Liu, G. R. Chen, D. J. Lee, T. Kawamoto, H. Tanaka, M. L. Chen and Y. K. Luo, Adsorption removal of cesium from drinking waters: A mini review on use of biosorbents and other adsorbents, Bioresour. Technol., 2014, 160, 142–149 Search PubMed.
- S. R. H. Vanderheyden, J. Yperman, R. Carleer and S. Schreurs, Enhanced cesium removal from real matrices by nickel-hexacyanoferrate modified activated carbon, Chemosphere, 2018, 202, 569–575 Search PubMed.
- S. Khandaker, Y. Toyohara, S. Kamida and T. Kuba, Adsorptive removal of secium from aqueous solution using oxidized bamboo charcoal, Water Res. Ind., 2018, 19, 35–46 Search PubMed.
- Y. Fan, B. Wang, S. Yuan, X. Wu, J. Chen and L. Wang, Adsorptive removal of chloramphenicol from wastewater by NaOH modified bamboo charcoal, Bioresour. Technol., 2010, 101, 7661–7664 Search PubMed.
- R. S. Zhao, J. P. Yuan, T. Jiang, J. B. Shi and C. G. Cheng, Application of bamboo charcoal as solid-phase extraction adsorbent for the determination of atrazine and simazine in environmental waster samples by high-performance liquid chromatography-ultraviolet detector?, Talanta, 2008, 76, 956–959 Search PubMed.
- W. Jiang, L. Zhang, X. Guo, M. Yang, Y. Lu, Y. Wang, Y. Zheng and G. Wei, Adsorption of cationic dye from water using an iron oxide/activated carbon magnetic composites prepared from sugarcane bagasse by microwave method, Environ. Technol., 2021, 42, 337–350 Search PubMed.
- B. B. Joanna and J. K. Agata, Cesium ion sorption on hybrid pectin-Prussian blue beads: Bath and column studies to remove radioactive cesium from contaminated wastewater, Hydrometallurgy, 2022, 213, 105937 Search PubMed.
- P. C. C. Faria, J. J. M. Orfao and M. F. R. Pereira, Adsorption of anionic and cationic dyes on activated carbons with different surface chemistries, Water Res., 2004, 38, 2043–2052 Search PubMed.
- G. Lee, C. Chen, S. T. Yang and W. S. Ahn, Enhanced adsorptive removal of fluoride using mesoporous alumina, Microporous Mesoporous Mater., 2010, 127, 152–156 Search PubMed.
- H. P. Boehm, Chemical identification of surface groups, Adv. Catal., 1996, 16, 179–274 Search PubMed.
- H. P. Boehm, Functional groups on the surfaces of solids, Angew. Chem., Int. Ed. Engl., 1996, 5, 533–544 Search PubMed.
- I. Langmuir, The adsorption of gases on plane surfaces of glass, mica and platinum, J. Am. Chem. Soc., 1918, 40, 1361–1403 Search PubMed.
- H. Freundlich, Over the adsorption in solution, J. Phys. Chem., 1906, 57, 384–471 Search PubMed.
- D. Karamanis and P. A. Assimakopoulos, Efficiency of aluminum-pillared montmorillonite on the removal cesium and copper from aqueous solutions, Water Res., 2007, 41, 1897–1906 Search PubMed.
- Y. S. Ho and G. MacKay, Pseudo-second-order model for sorption process, Process Biochem., 1999, 34, 451–465 Search PubMed.
- F. Ogata, S. Kawamoto, A. Tabuchi, M. Toda, M. Otani and N. Kawasaki, Feasibility of nickel-aluminum complex hydroxides for recovering tungsten ions from aqueous media, Sustainability, 2022, 14, 3219 Search PubMed.
- G. A. Rahman Dakroury, S. F. Abo-Zahra, H. S. Hassan and H. E. Ahmed Ali, Improvement of the sorption behavior of aluminum silicate composite toward 134Cs and 60Co radionuclides by non-living biomass of Chlorella vulgaris, Environ. Sci. Pollut. Res., 2020, 27, 21109–21125 Search PubMed.
-
Proceedings of 1989 SME Symposium on Biotechnology in Minerals and Metal Processing, ed. M. R. Ferguson, T. M. Peterson, A. Jeffers, B. J. Scheiver, F. M. Doyle, and S. K. Kawatra, SME, 1989, vol. 24, pp. 201–207 Search PubMed.
- I. Abe, M. Hayashi and M. Kitagawa, Studies on the adsorption of surfactants on activated carbon, J. Jpn. Oil Chem. Soc., 1976, 25, 145–150 Search PubMed.
- J. Mizera, G. Mizerová, V. Machovič and L. Borecká, Sorption of cesium, cobalt and europium on low-rank coal and chitosan, Water Res., 2007, 41(3), 620–626 Search PubMed.
- M. Caccin, F. Giacobbo, M. Da Ros, L. Besozzi and M. Mariani, Adsorption of uranium: cesium and strontium onto coconut shell activated carbon, J. Radioanal. Nucl. Chem., 2013, 297, 9–18 Search PubMed.
- S. Khandaker, T. Kuba, S. Kamida and Y. Uchikawa, Adsorption of cesium from aqueous solution by raw and concentrated nitric acid–modified bamboo charcoal, J. Environ. Chem. Eng., 2017, 5, 1456–1464 Search PubMed.
- C. Chen and C. J. Wang, Removal of Pb2+, Ag+, Cs+ and Sr2+ from aqueous solution by brewery's waste biomass, J. Hazard. Mater., 2008, 151(1), 65–70 Search PubMed.
- S. Dahiya, R. Tripathi and A. Hegde, Biosorption of heavy metals and radionuclide from aqueous solutions by pre-treated arca shell biomass, J. Hazard. Mater., 2008, 150(2), 376–386 Search PubMed.
- D. Chakraborty, S. Maji, A. Bandyopadhyay and S. Basu, Biosorption of cesium-137 and strontium-90 by mucilaginous seeds of Ocimum basilicum, Bioresour. Technol., 2007, 98, 2949–2952 Search PubMed.
- M. R. Awual, T. Yaita, Y. Miyazaki, D. Matsumura, H. Shiwaku and T. Taguchi, A reliable hybrid adsorbent for efficient radioactive cesium accumulation from contaminated wastewater, Sci. Rep., 2016, 6, 19937 Search PubMed.
- D. Ding, Z. Lei, Y. Yang, C. Feng and Z. Zhang, Selective removal of cesium from aqueous solutions with nickel(II) hexacyanoferrate(III) functionalized agricultural residue-walnut shell, J. Hazard. Mater., 2014, 270, 187–195 Search PubMed.
- M. R. Awual, T. Yaita, T. Taguchi, H. Shiwaku, S. Suzuki and Y. Okamoto, Selective cesium removal of radioactive liquid waste by crown ether immobilized new class conjugate adsorbent, J. Hazard. Mater., 2014, 278, 227–235 Search PubMed.
- D. Tang, M. Yin, X. Du, Y. Duan, J. Chen and T. Qiu, Wettability tunable conjugated microporous poly(aniline)s for long-term, rapid and ppb level sequestration of Hg(II), Chem. Eng. J., 2023, 474, 145527 Search PubMed.
- X. Lou, D. Tang, C. Ye, J. Chen and T. Qiu, Conjugated micro-mesoporous poly(aniline)s for ultrafast Hg(II) capture, Sep. Purif. Technol., 2023, 325, 124646 Search PubMed.
|
This journal is © The Royal Society of Chemistry 2024 |
Click here to see how this site uses Cookies. View our privacy policy here.