DOI:
10.1039/D4SM00521J
(Paper)
Soft Matter, 2024,
20, 7111-7121
Understanding the gelation properties of the fluorophenyl glycosides of arabinoside gelators: experimental and theoretical studies†
Received
1st May 2024
, Accepted 11th July 2024
First published on 11th July 2024
Abstract
In supramolecular gelation, fluorinated gelators are important due to the unique properties displayed by these compounds that arise out of the presence of fluorine atoms. Generally, incorporation of fluorine leads to higher mechanical strength of the gels compared to their non-fluorinated counterparts and this property is enhanced with increasing the number of fluorine atoms. Herein, we show that the incorporation of fluorine into the phenyl ring of phenyl arabinoside allows the molecule to act as a gelator, unlike the non-fluorinated compound. We also show that the mechanical strength and stiffness of the gels is not only dependent on the positions of the fluorine atoms but also guided by their number. Detailed experimental studies, supported by computational studies, allowed us to rationalize the observed supramolecular interactions and propose reasons based on the conformational preferences of these compounds that allow additional hydrogen bonds and π–π interactions which guide the self-assembly, in addition to the primary H-bonding interactions. This, in turn, affects the mechanical behavior of these gels.
1 Introduction
Fluorinated gelators have attracted attention as drug delivery agents due to their unique properties that are imparted to these compounds by the presence of one or multiple fluorine atoms.1,2 Among the first examples of fluorinated gelators, Carran reported fluorinated propargylic alcohols as gelators of alkane liquids and/or silicone oil (Fig. 1).3 Raghavanpillai reported a series of fluorinated bis-amide and bis-urea gelators with enhanced gelation abilities than the non-fluorinated counterparts, which was rationalized by the extra surface roughness and the presence of fluoroalkyl functionalities in the gelator backbone.3 Steed also demonstrated that appending fluorous tails to bis-urea gelators led to thinner fibres than their non-fluorinated partners which in turn led to more robust gels.4 Fluorinated phenylalanine derivatives are particularly efficient and Tomasini further reported their dipeptide versions that were very efficient hydrogelators.5 Particularly interesting was the fact that increasing the number of F-atoms on the gelator led to increased stiffness of the gels, a property that was attributed to the presence of additional halogen bonds because of the increased number of F-atoms.6 Nilsson reported a perfluorophenyl alanine gelator for water, in which the perfluorination accentuates the hydrophobic and electronic properties of F5-phenylalanine.7 In a subsequent study, Nilsson also showed that fluorination of the phenyl ring in phenylalanine not only increases the gelation ability, but it does so more effectively than other halogens. Also, the position of the fluorination in the aryl ring controls the gelation properties.8 Hatanaka demonstrated that fluorogelators based on perfluoroalkyl ethers of anthracene and anthraquinone were particularly useful for gelation of perfluorinated solvents.9 The advantages of incorporating F-atoms in LMOGs stem from the fact that they provide additional sites for supramolecular assembly via H-bonding or halogen-bonding10–12 interactions.
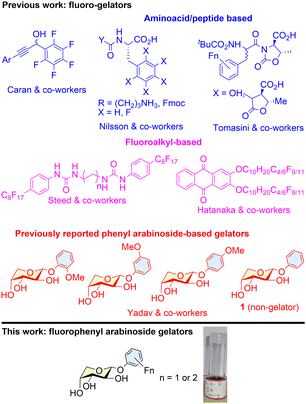 |
| Fig. 1 Previously reported fluoro and phenylarabinoside gelators. | |
Carbohydrate based supramolecular gelators are widely reported because they are biocompatible and easily available, along with the fact that they can be easily manipulated to afford diverse structural features that enhance their gelation properties.13–15 Although the primary interaction responsible for the self-assembly in carbohydrate based low molecular weight gelators (LMOGs) is H-bonding, other weak interactions are frequently used for imparting amphiphilic nature to the molecules for gelation. Thus, additional non-covalent interactions like π–π interactions through aromatic groups,16–20 hydrophobic interactions through aliphatic tails,21–30 halogen bonding interactions through introduction of halogen (Cl, Br, I) atoms31,32 as well as additional H-bonding interactions through amino acids31,33–37 are introduced into the sugar core for enhancing their gelation abilities, in line with the broad principles of designing LMOGs.
However, to date there have been only a few reports of fluorine bearing carbohydrate LMOGs.38 We have been interested in the synthesis of structurally simple arabinose based gelators with a view to understanding the factors that enable the self-assembly of this class of molecules.39–42 However, the simple phenolic glycoside of arabinose bearing no substitutions on the phenyl ring was unable to act as a gelator of any solvent (1, Fig. 1).43 Due to the importance of such gelators towards controlled release of drugs and the fact that fluorinated gelators are more suitable for the delivery of some fluorinated drugs,1,5,6 we explore the effect of incorporation of F-atoms on the gelation properties of such phenolic glycoside 1 and herein report the results of our studies of the structure–activity relationship of introducing F-atoms in the phenyl part of the glycoside (3a–e) using experimental and computational tools.
2 Materials and methods
2.1 Syntheses of 3a–e
The small molecule gelators were synthesized by a simple glycosylation reaction involving the reaction of the fluorinated phenols with α-2,3,4-O-acetyl-1-bromoarabinopyranoside under basic conditions. The resulting α-2,3,4-O-acetyl-1-bromoarabinopyranoside, in turn, was obtained by the treatment of 1,2,34-tetra-O-acetylarabinopyranose with PBr3. The 1-fluorophenyl β-2,3,4-acetylarabinoside thus obtained was subjected to global deacetylation using NaOMe/MeOH to yield the desired fluorophenyl arabinosides 3a–e (Scheme 1). Details of the experimental procedures followed are provided in the ESI.†
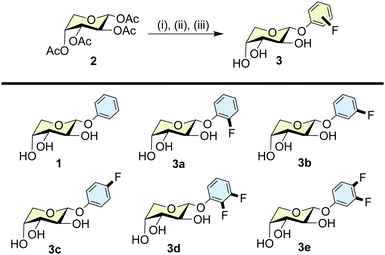 |
| Scheme 1 Synthesis and the structures of 1, 3a, 3b, 3c, 3d, and 3e. Reagents and conditions: (i) PBr3, AcOH, RT, 4 h; (ii) NaH, DMF, RArOH, RT, 6 h; and (iii) NaOMe (Cat.), MeOH, RT, 1 h. | |
2.2 Preparation of gels
For the gelation experiments, fluoro compounds (3a–e) were weighed in a 5 ml sample vial and an organic solvent or water (the detailed solvent list is provided in the ESI†) was added to it and then the vial was warmed in a water bath at 50–80 °C until a clear solution was obtained. After that, it was cooled at room temperature for 5–10 minutes, after which the vial was inverted to check gelation. It was considered a gel if the inverted sample could support the system's weight. If gels were formed, their stability was tested by keeping the vial inverted for a minimum of 24 h. All the fluoro compounds 3a–e were found to be able to gel aromatic organic solvents like benzene, toluene, ortho-, meta- or para-xylene, and chlorobenzene (see Fig. S1–S5 and Table S1, ESI†).
2.3 Determination of minimum gelation concentration (MGC)
To determine the minimum gelation concentration, 1 mg of a fluoro compound (gelator) was taken in a 5 ml sample vial, 1 ml of solvent was added, and the heating and cooling was conducted as above. If a gel was not formed, 1 mg incremental weights of the gelator were added and the above cycle was repeated until a gel was formed. The minimum weight of the gelator that formed the gel was reported as the minimum gelation concentration (MGC). If no gels were formed till the addition of 20 mg of the gelator, then the solvent was considered to be non-gellable. The fluoro compounds 3a–3e had minimum gelation concentrations (MGCs) in the range of 0.5% to 1% w/v (see Table S1, ESI†) for solvents such as benzene, toluene, ortho-, meta- or para-xylene, and chlorobenzene.
2.4 Field emission scanning electron microscopy (FESEM)
Samples for FESEM analysis of gels from 3a–e were prepared by warming a 1% w/v solution of each gelator in p-xylene, which was then drop-cast onto a glass slide (2 mm × 2 mm) carefully. Then, it was dried in air overnight to allow the solvent to evaporate, followed by drying in a desiccator for one week. The dried xerogel containing glass slides were placed on a stub, and gold sputter coating was performed with a Quorum-Q150RES sputter coater under a vacuum of 5 × 10−5 mbar and a current of 20 mA for 2 minutes. The samples were then subjected to FESEM experiments using a Zeiss Supra-55 FESEM. The operating voltage for the imaging was 5 kV with an in-lens signal detection system as the detector.
2.5 Rheology
The samples for rheological studies of all the gelators 3a, 3b, 3c, 3d, and 3e were prepared at 1% w/v in p-xylene solvent. The experiments were carried out with a Anton Paar MCR 302e rheometer using parallel plates (8 mm, stainless steel) with gaps of 500 microns between the parallel plates. The samples were placed through a spatula on the parallel plates, and the gel was distributed equally. Two different experiments were carried out to study the mechanical properties of gels: dynamic strain sweep (DSS) and dynamic frequency sweep (DFS). Dynamic strain sweep experiments were conducted at a constant frequency of 1 Hz and 25 °C temperature. The strain value was determined at a point where tan
δ = G′′/G′ (G′′ = loss modulus; G′ = storage modulus). Variation of the storage modulus (G′) was carried out in the frequency range of 0.1 Hz to 100 Hz and a strain of 0.01% through DFS experiments.
2.6 Wide angle X-ray diffraction (WXRD)
The samples for WXRD experiments were prepared by dissolving 3a, 3b, 3c, 3d, and 3e (50 mg) in 5 ml of p-xylene solvent in a beaker and then drying the gels over a period of 7 days in a desiccator under nitrogen stream. The WXRD diffractogram of the samples was recorded on a Rigaku SmartLab diffractometer. The Converging Beam Optics (CBO) technology was used for fully automated beam alignment. X-rays of 1.5 Å wavelength were used.
2.7 FTIR experiments
FTIR experiments were carried out on a PerkinElmer Spectrum Two FTIR spectrometer. Crystalline samples for IR experiments were prepared by crystallization from CH2Cl2–hexane mixtures. For FTIR experiments in the gel state, the p-xylene gel of each gelator was analyzed on a KBr pellet. A comparison of the nature of the H-bonding during the self-assembly and the crystalline state was made based on the shifts of the O–H stretching frequencies in respective states.
2.8 Computational methods
The ORCA 5.0.4 program44 was used to determine all the possible geometry-optimized structures as well as all possible H-bonding and π–π interactions, their electron density maps, molecular electrostatic potential surface maps and the energies of the HOMO and LUMO, employing density functional theory (DFT) calculations at the B3LYP level.45 Initial geometries of 3a–e were modelled in the Avogadro46 molecular builder and visualization program. The nature of stationary points for all the modelled geometries was confirmed by the frequency calculations at the same level. All the molecules were optimized to their energy minimum geometries. The absence of negative frequencies of the corresponding minima confirmed the localization of the stable states of molecules on the potential energy surface (PES). The def2-SVP basis set47 was used for all atoms. All the optimized geometries, molecular electrostatic potential surface maps, and HOMO–LUMO were represented with the help of the Chemcraft48 visualization program.
3 Results and discussion
3.1 Gelation properties
The gelation abilities of 3a–e were determined in a range of solvents (see Fig. S1–S5, ESI†) by a simple inversion test, wherein it was observed that all the compounds 3a–e formed gels with aromatic organic solvents like benzene, toluene, xylenes and chlorobenzene (Table 1). Their minimum gelation concentrations were determined and the results are presented in Table 1. From the MGC experiments, it was found that the gels were formed in the concentration range of 0.5% (w/v) to 1.0% (w/v) for the solvents listed in Table 1. The lowest MGC was displayed by the 3,4-difluorinated phenolic glycoside 3e (0.5%, w/v) for all the solvents that were gelled. In contrast, the 2,3-difluorinated gelator 3d gelled these solvents with an MGC at 1.0% (w/v). The thermal stability of the gels at the minimum concentration was also studied by determining the temperature for the gel–sol conversion (Tg). The highest thermal stability of the gels as determined by their Tg for any particular solvent was also displayed by gelator 3e, when compared to the other gelators. The thermal reversibility of the gels was also studied over ten heating–cooling cycles and all the gels were found to be thermally reversible. The gels were also found to be stable under ultrasonic radiation or upon exposure to UV-light.
Table 1 Gelation studies of 3a–e in various solvents
Solventa |
(MGC,bTgc) |
3a
|
3b
|
3c
|
3d
|
3e
|
G = gel.
MGC = minimum gelation concentration % (w/v).
T
g = gelation temperature.
|
Benzene |
G,a (0.5%, 42–44 °C) |
G, (0.6%, 47–49 °C) |
G, (0.6%, 40–42 °C) |
G, (1.0%, 55–57 °C) |
G, (0.5%, 66–68 °C) |
Toluene |
G, (0.6%, 51–53 °C) |
G, (0.7%, 54–56 °C) |
G, (0.7%, 53–55 °C) |
G, (1.0%, 61–63 °C) |
G, (0.5%, 70–72 °C) |
o-Xylene |
G, (0.7%, 56–58 °C) |
G, (0.7%, 55–57 °C) |
G, (0.7%, 58–60 °C) |
G, (1.0%, 60–62 °C) |
G, (0.5%, 69–71 °C) |
m-Xylene |
G, (0.7%, 57–59 °C) |
G, (0.7%, 59–61 °C) |
G, (0.8%, 59–61 °C) |
G, (1.0%, 63–65 °C) |
G, (0.5%, 72–74 °C) |
p-Xylene |
G, (0.6%, 57–59 °C) |
G, (0.8%, 54–56 °C) |
G, (0.7%, 56–58 °C) |
G, (1.0%, 60–62 °C) |
G, (0.5%, 71–73 °C) |
Chlorobenzene |
G, (0.7%, 53–54 °C) |
G, (0.7%, 56–58 °C) |
G, (0.8%, 57–59 °C) |
G, (1.0%, 61–63 °C) |
G, (0.5%, 73–75 °C) |
The rheological properties of the p-xylene gels formed by each gelator were also determined through dynamic frequency sweep (DFS) and dynamic strain sweep (DSS) experiments (Fig. 2). From the DSS experiments, the highest limiting strain for the gel-to-sol conversion (γ) was observed for the meta-fluorophenyl glycoside 3b (Table 2). The gelator 3b also showed the lowest tan
δ value. Both these results demonstrated that gelator 3b formed a mechanically robust gel. The mechanically weakest gel was formed by gelator 3a, followed by the difluorinated gelators 3d and 3e.
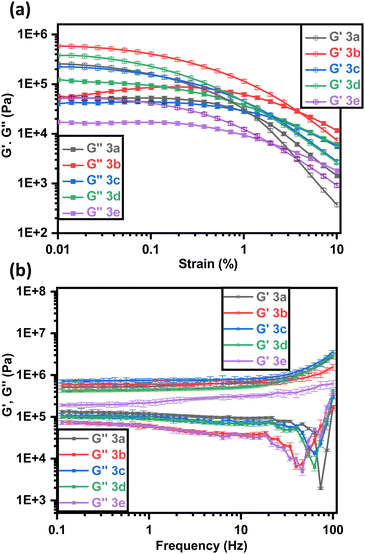 |
| Fig. 2 Rheology of 3a–e gels with p-xylene at 1% (w/v) at 25 °C: (a) DSS curves at a frequency of 1 Hz and (b) DFS curves at a strain of 0.01%. | |
Table 2 Rheological properties of p-xylene gels of 3a–e
Organo-gelators |
p-Xylene |
γ (%), × 10−2 |
G′a (Pa), × 105 |
G′′ b (Pa), × 105 |
tan δc |
G′ = storage modulus.
G′′ = loss modulus.
tan δ = G′′/G′.
|
3a
|
124 ± 7.4 |
6.37 ± 0.62 |
1.01 ± 0.09 |
0.159 ± 0.001 |
3b
|
391 ± 19.5 |
6.33 ± 0.55 |
0.42 ± 0.03 |
0.066 ± 0.001 |
3c
|
237 ± 16.6 |
8.22 ± 0.8 |
0.80 ± 0.07 |
0.097 ± 0.001 |
3d
|
156 ± 7.8 |
4.90 ± 0.45 |
0.75 ± 0.07 |
0.152 ± 0.001 |
3e
|
196 ± 9.8 |
2.80 ± 0.24 |
0.40 ± 0.03 |
0.142 ± 0.002 |
To rationalize the differences in the mechanical strengths of the different gels, different experiments were performed. The microstructures of the gels were studied by FESEM experiments of their xerogels prepared from the corresponding p-xylene gels (Fig. 3). The FESEM micrographs revealed that while gelator 3a formed sheet like structures, the other gelators formed interconnected fiber bundles, thereby imparting robust mechanical properties to the gels (Table S7, ESI†).
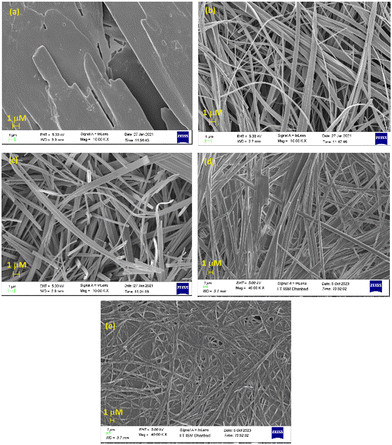 |
| Fig. 3 FESEM micrographs of p-xylene gels of 3a–e. | |
Powder X-ray diffraction experiments of the p-xylene derived xerogels of 3a–e revealed that the gels were polycrystalline and several diffraction planes resulting from different kinds of interactions were observed (see Fig. S5–S10, ESI†). All the compounds showed peaks in the region of 2θ corresponding to hydrogen bonding distances in the range of 2.7–3.3 Å as well as peaks for π–π stacking at 2θ corresponding to 3.4 A to 3.6 Å. Further proof of the supramolecular assembly via H-bonding was also obtained from the FTIR experiments. A consistent higher shift of the O–H stretching frequency in the gel state was observed for all the gelators 3a–e when compared to the solid-state absorption for the same (Fig. 4). This indicated that the H-bonding involving the O–H groups were more robust in the crystalline state than in the gel state which was expected, considering that molecular packing (or assembly) in the gel state is somewhat in between the purely crystalline state and the purely amorphous state.
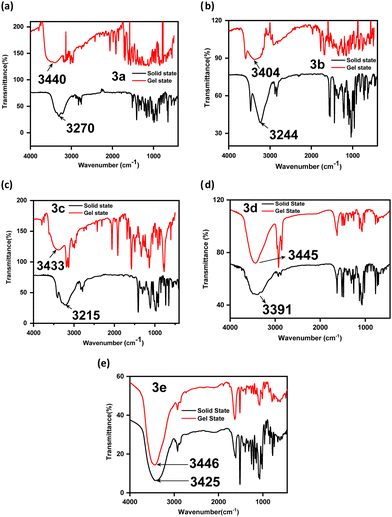 |
| Fig. 4 Comparison of the IR spectra {O–H stretching frequency (cm−1) in crystalline (black lines) and gel (benzene) states (red lines)} of 3a–e. | |
3.2 DFT calculations to understand the self-assembly mechanism
The phenolic glycoside of arabinose 1 was previously found to be a non-gelator.43 Again, the self-assembly pattern and the mechanism of self-assembly of methoxy-substituted phenolic glycosides were already studied in our earlier report through DFT calculations.43 The H-bonding interactions and π–π stacking were identified as the main contributors towards the self-assembly for enabling gelation. Here, the experimental results point to the fact that the gelation abilities and the gelation properties are not only dependent on the presence of the F-atoms but also influenced by their number and position in the phenyl ring. To clearly understand the gelation strength pattern – meta- (3b) > para- (3c) > meta–para- (3e) > ortho–meta- (3d) > ortho- (3a) – and to identify the possible modes of self-assembly during gelation, theoretical calculations were performed on all the compounds. The effect of fluoro-substituted phenyl rings and their orientation plays a significant role in the gelation as the non-fluorinated analogue 1 does not display any gelation property. To rationalize the lack of gelation by compound 1, the H-bonded self-assembly process for it was also computed. The dimer formation energy for 1 was calculated to be about 23–31 kJ mol−1 less stable than those for the fluorinated analogues (i.e.3a–e) (see Table S38, ESI†). This leads us to believe that fluoro-substitutions and their placement in the phenyl rings are the key determining factors for imparting the gelation ability as well dictating the variations in the gel strength.
The geometry optimizations of ortho- (3a), meta- (3b), para- (3c), ortho–meta- (3d) and meta–para- (3e) compounds were performed at the B3LYP level. The FTIR analysis and WXRD experiments already hinted that both the H-bonding and π–π interactions simultaneously exist. Thus, the self-assembly possibilities of all the compounds with both H-bonding (3-H) and π–π stacking (3-π) were explored. During the self-assembly, two sets of possible geometries were investigated. One set of possibilities were where the fluorine atoms were distal (i.e. far apart) from the pyranose-oxygen of the sugar moiety (3a/3a-H/3a-π, 3b/3b-H/3b-π, 3d/3d-H/3d-H_a/3d-π and 3e/3e-H/3e-π). The other set explored the possibilities of proximal (i.e. closer) fluorine atoms (3a′/3a′-H/3a′-π, 3b′/3b′-H/3b′-π, 3d′/3d′-H/3d′-π and 3e′/3e′-H/3e′-π).
Electronic repulsion was observed between the ortho-fluorine atom and glycosidic oxygen in 3a′ (electron density map; Fig. 5(a)), which made it less stable than 3a (ΔH(ΔG) = +19.45(+19.07) kJ mol−1). The molecular electrostatic potential map (Fig. 5(a)) revealed that the hydroxyl groups of the sugar were capable of intermolecular H-bonding interactions (3a-H and 3a′-H; Fig. 5(b)) to form a dimer. Due to the existence of an extra intramolecular H-bonding interaction provided by fluorine, 3a-H is found to be more stable than 3a′-H (ΔH(ΔG) = −25.81(−0.23) kJ mol−1). The HOMO and LUMO of both 3a and 3a′ revealed that the electron pair resides mainly on phenolic moieties (Fig. 5(a)). Thus, π–π stacking between two phenolic moieties was considered during the self-assemblies. In 3a-π, the π–π stacking distance was found to be ∼3.55 Å (with an intramolecular H-bonding interaction between ortho-F and pyranose hydroxyl groups), whereas in 3a′-π the distance was ∼3.70 Å, without any inter- or intramolecular H-bonding interactions (Fig. 5(c)). Expectedly, 3a-π was found to be more stable than 3a′-π (ΔH(ΔG) = −35.49(−56.09) kJ mol−1).
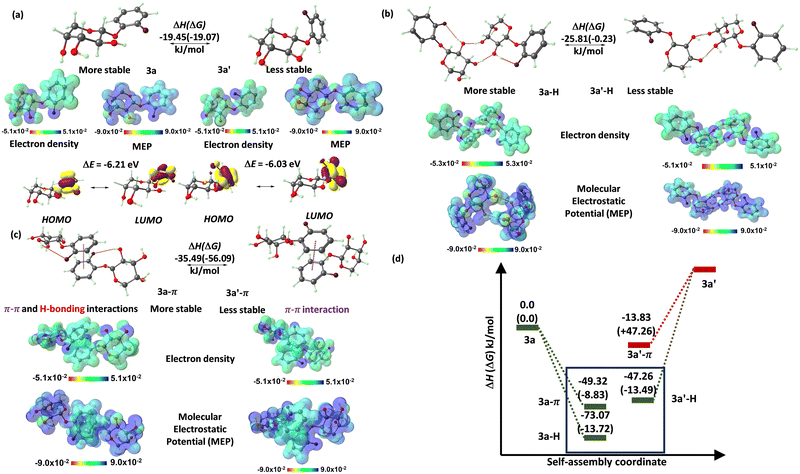 |
| Fig. 5 DFT-optimized geometries, electron density maps and molecular electrostatic potential surface maps of (a) 3a and 3a′, (b) 3a-H and 3a′-H and (c) 3a-π and 3a′-π. (d) Energy profile diagram for the self-assembly of the dimer. | |
During gelation, two H-bonded dimers (either 3a-H or 3a′-H) can be assembled further by weak π–π interactions between the phenolic moieties of each dimeric unit and form a 1D chain in a [π-(H⋯H)-π]–[π-(H⋯H)-π]–[π-(H⋯H)-π]… fashion. Alternatively, two π–π stacked dimers could combine via strong H-bonding interactions between terminal pyranose groups in an [H-(π–π)-H]⋯[H-(π–π)-H]⋯[H-(π–π)-H]… fashion. The energies of 3a-H, 3a-π, and 3a′-H were found to be close to each other, while 3a′-π was found to be comparatively unstable (Fig. 5(d)). All four sets of dimers could exist in the system as they were energetically more stable than 3a. Thus, only three energetically compatible sets of dimers could be self-assembled further in any possible fashion and would make the gelation stronger. On the other hand, 3a′-π would make the gel weaker by destabilizing the binding. This can be ascribed to the ortho-destabilizing factor, which we refer to as the ortho effect.
In the case of the meta-substituted compound (3b), the electron density map revealed that the electronic repulsion between the meta-fluorine and the pyranose oxygen (Fig. 6(a)) was less, as they are far apart. Interestingly, 3b′ was actually found to be slightly more stable than 3b (ΔH(ΔG) = −14.75(−14.07) kJ mol−1), unlike in the case of ortho-F, where 3a was more stable. Once again, the H-bonded {between the sugar moieties; 3b-H and 3b′-H; (ΔH(ΔG) = −7.39(+7.38) kJ mol−1); Fig. 6(b)} and π–π stacked dimers {between the meta-substituted phenyl rings, 3b-π and 3b′-π; Fig. 6(c)} were considered and optimized to their energy minimized geometries. For 3b-π, the π–π stacking distance was found to be ∼3.61 Å along with a weak intermolecular H-bonding interaction provided by the meta-fluorine. However, in 3b′-π, no π–π stacking could be identified. Instead, the dimeric assembly was found to be poorly held by two weakly intermolecular H-bonding interactions (between the pyranose ring and meta-fluoro phenyl group). In terms of enthalpy, 3b′-π was just slightly more stable than 3b-π, but when Gibbs free energy was considered, 3b-π is comparatively stable (ΔH(ΔG) = 2.92(−19.29) kJ mol−1). The energies of 3b-H, 3b′-H, 3b-π and 3b′-π were all in close proximity (Fig. 6(d)) and all of them were fairly stable compared to that of 3a. Thus, comparatively stable self-assembly was possible between the four sets of dimers in any possible combinations. This particular phenomenon occurred due to the unique position of meta-fluorine and low electronic repulsion between glycosidic oxygen and fluorine. This can be ascribed to the stabilizing factor, which we refer to as the meta-effect.
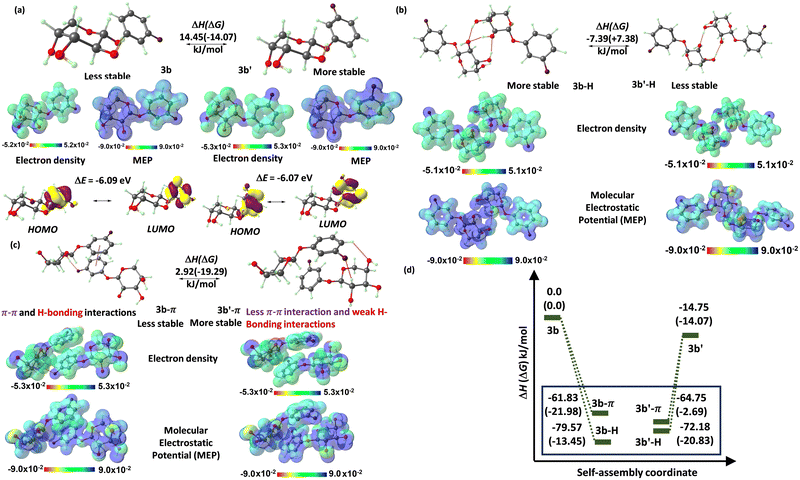 |
| Fig. 6 DFT-optimized geometries, electron density maps and molecular electrostatic potential surface maps of (a) 3b and 3b′, (b) 3b-H and 3b′-H and (c) 3b-π and 3b′-π. (d) Energy profile diagram for the self-assembly of the dimer. | |
In the para-fluorinated gelator 3c, only one geometry (Fig. 7(a)) was possible and thus considered during optimization. During the self-assembly, both H-bonding (Fig. 7(b)) and π–π stacking interactions (∼3.71 Å) (Fig. 7(c)) were explored as suggested for 3a and 3b. The energy difference between 3c-H and 3c-π is only 5.26(8.16) kJ mol−1 (Fig. 7(d)). Thus, strong assemblies (between 3c-H–3c-H or 3c-π–3c-π) were possible between two dimers during gelation. As the H-bonding interactions are weaker compared to 3b (Fig. 6 and 7) (see Table S39, ESI†), the gelation strength would likely be weaker than that of 3b but much stronger than that of 3a.
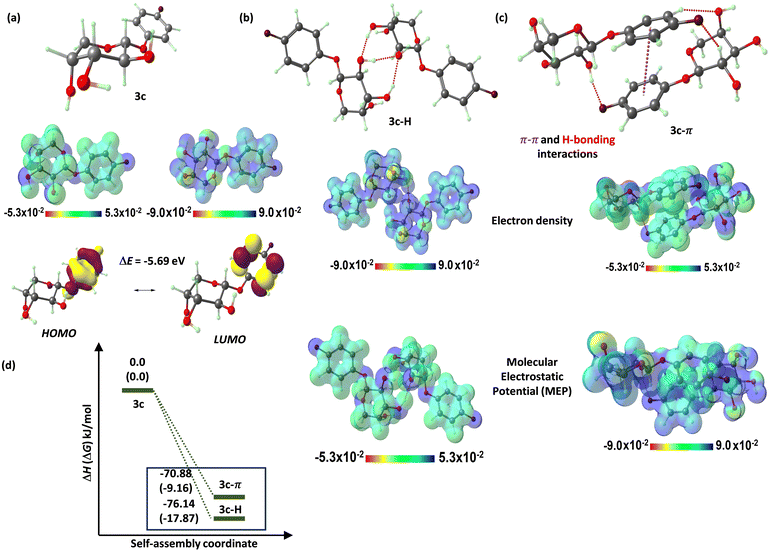 |
| Fig. 7 DFT-optimized geometries, electron density maps and molecular electrostatic potential surface maps of (a) 3c, (b) 3c-H and (c) 3c-π. (d) Energy profile diagram for the self-assembly of dimers. | |
It is generally reported in the literature that the gel strength is stronger with an increasing number of fluoro-substitutions. Thus, ortho-meta-difluorinated gelator 3d and meta–para-difluorinated gelator 3e were initially expected to form more robust gels. However, the rheology experiments proved otherwise. In line with the ortho- and meta-effects that we observed for the mono-fluorinated gelators 3a and 3b, respectively, the presence of the difluoro substitution on 3d, the destabilizing ortho-effect is expected to weaken the self-assembly, whereas the meta-effect would strengthen it. However, DFT studies (Fig. 8) showed that an extra set of H-bonded geometries was prevalent which was possible for 3d (3d-H_a; Fig. 8(b)). In 3d-H_a, intermolecular H-bonding interactions are present between the ortho- and meta-fluoro and pyranose hydroxide groups. Compared to this, in 3d-H, strong H-bonding interactions could be seen between two pyranose moieties, making the assembly more stable than 3d-H_a (Fig. 8(b)). 3d-H, 3d-H_a and 3d-π are found to be comparatively more stable (Fig. 8(b)–(d)) as expected due to the meta-effect and 3d′-H and 3d′-π were destabilized by the ortho-effect (Fig. 8(b) and (c)). Three stable dimers (3d-H_a, 3d-H and 3d-π) would make the gelation strong, by combining themselves in any possible fashion, while 3d′-π and 3d′-H would weaken the gelation by providing weaker interactions. Thus, the opposing ortho- and meta-effects, with the meta-effect dominating, made the gelation somewhat stronger than 3a but weaker than 3b or 3c. The meta–para-difluorinated gelator 3e, in the absence of any destabilizing factors (Fig. 8(a)), constituted stable H-bonded dimers (3e-H and 3e′-H) and π–π stacked dimers (3e-π and 3e′-π) (Fig. 9(b) and (c)). But, unlike 3a, 3b or 3d, the two sets of possibilities were geometrically and energetically almost identical (Fig. 9(d)). Thus, instead of two sets of orientations and assemblies, only one set of dimers was likely to exist (like 3c). Therefore, only homo-assembly (between 3e-H and 3e′-H or between 3e-π and 3e′-π) was possible and thus the gelation strength would be also closer to that of 3c instead of 3b. The energy differences between (3e-H and 3e-π) and (3e′-H ad 3e′-π) are 9.19(8.63) and 7.62(13.31). These values are comparatively higher than that of 3c. Thus, the gelation strength is likely to be less than that of 3c but in the absence of any destabilizing factor, it is far stronger than that of 3d.
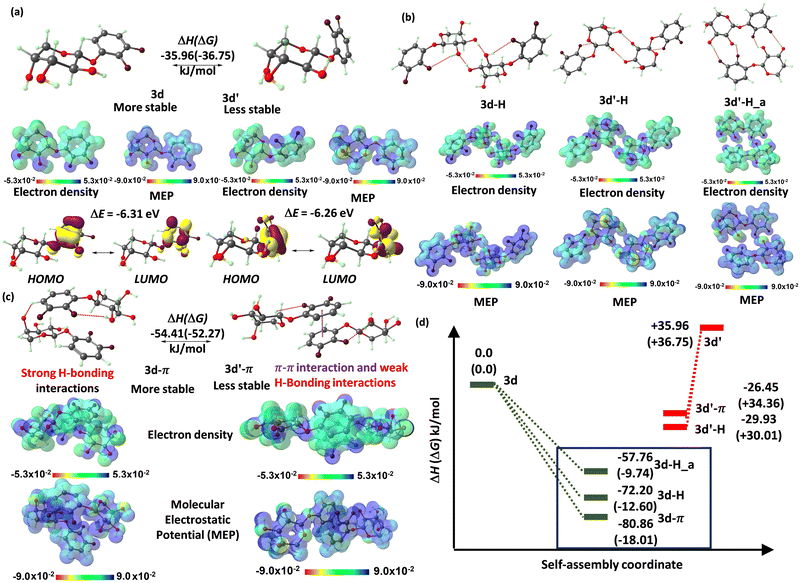 |
| Fig. 8 DFT-optimized geometries, electron density maps and molecular electrostatic potential surface maps of (a) 3d and 3d′, (b) 3d-H, 3d-H_a and 3d′-H and (c) 3d-π and 3d′-π. (d) Energy profile diagram for the self-assembly of dimers. | |
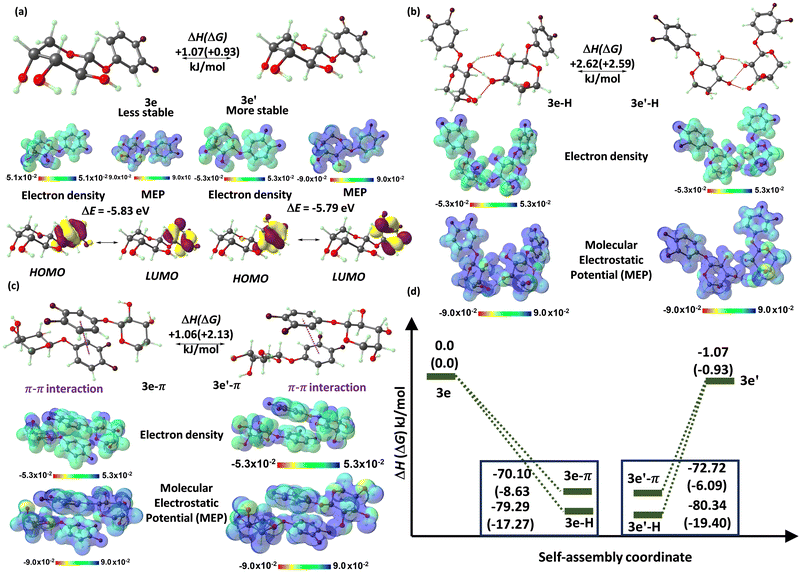 |
| Fig. 9 DFT-optimized geometries, electron density maps and molecular electrostatic potential surface maps of (a) 3e and 3e′, (b) 3e-H and 3e′-H and (c) 3e-π and 3e′-π. (d) Energy profile diagram for the self-assembly of dimers. | |
The DFT calculated dipole moment trends for these five compounds were also in line with the mechanical strengths of the gels, i.e.3b > 3c > 3e > 3d > 3a (see Table S39, ESI†). Castro and co-workers mentioned the relation between the hydrogen bonding and the dipole moment of hydrofluorocarbons.49 The hydrogen bonding is expected to be higher with a larger dipole moment value. Overall, the energetics of the H-bonding interactions and π–π stacking interactions, and dipole moment values obtained from DFT calculations thus support the order meta- (3b) > para- (3c) > meta–para- (3e) > ortho–meta- (3d) > ortho- (3a) and also provide a rationale for the observed order of the gel strengths.
4 Conclusions
In summary, we have reported carbohydrate based fluoro gelators as the fluorophenyl glycosides of arabinose. Several mono- and difluorophenyl arabinosides were synthesized and were able to gel different aromatic solvents. The gels were characterized through various techniques. The rheology of the gels indicated that the mechanical strength of the gels followed the order meta-fluorophenyl (3b) > para-fluorophenyl (3c) > meta–para-difluorophenyl (3e) > ortho–meta-difluorophenyl (3d) > ortho-fluorophenyl (3a). The mechanical strength of the gels could be correlated with the self-assembly of the monomers. This self-assembly occurred mainly due to H-bonding interactions and π–π stacking. The WXRD data suggested that both of them exist in our systems. Computational studies on H-bonded and π–π stacked dimers were performed. The results revealed that the arabinopyranoside hydroxyl groups were able to form strong intermolecular H-bonding with other pyranoside groups along with weak intra- and inter-molecular H-bonding with fluorophenyl groups. π–π stacking was possible between the fluorophenyl moieties of two arabinoses. Further assemblies to form tetramers, octamers, and so on could occur between two H-bonded dimers through π–π stacking or between π–π stacked dimers through H-binding interactions. Due to a strong electronic repulsion between ortho-fluorine and pyranose oxygen, ortho-substituents diverged to an unstable dimeric structure, which resulted in the weakening of gelation strength. On the other hand, on the meta- and para-substituents, the absence of such repulsion would have resulted in a far stronger gelation. Due to stronger primary H-bonding interactions, 3b formed a slightly robust gel. Di-fluoro substitution also reflected the same results. Due to the presence of the contradictory destabilising ortho-effect and stabilising meta-effect, the mechanical strength of 3d was found to be lower than those of 3b, 3c and even 3e. The dipole moment values which could be an indicator of binding strength were also calculated with the help of geometry optimization at the B3LYP level and it was found that they follow the same trend as the mechanical strength obtained from rheological data. This provides a detailed understanding of the effect of fluorophenyl on gel strength when they were introduced in β-arabinopyranoside gelators.
Author contributions
SNP, NPP and AS were responsible for the investigations and formal analysis. SY was responsible for the conceptualization and supervision of the work and the manuscript was written through contributions of all authors.
Data availability
The data supporting this article have been included as part of the ESI.†
Conflicts of interest
The authors declare no conflicts of interest.
Acknowledgements
SNP and NPP acknowledge IIT (ISM) Dhanbad for PhD fellowships. AS acknowledges IIT (ISM) Dhanbad for a post-doctoral fellowship.
Notes and references
- D. M. Raymond, B. L. Abraham, T. Fujita, M. J. Watrous, E. S. Toriki, T. Takano and B. L. Nilsson, ACS Appl. Bio Mater., 2019, 2, 2116 CrossRef CAS PubMed
.
- P. Tiwari, A. Gupta, R. R. Mehra, N. Khan, J. Harjit, C. R. Ashby Jr, A. Basu, A. K. Tiwari, M. Singh and A. D. Konar, Supramol. Chem., 2020, 32, 495 CrossRef CAS
.
- A. R. Borges, M. Hyacinth, M. Lum, C. M. Dingle, P. L. Hamilton, M. Chruszcz, L. Pu, M. Sabat and K. L. Caran, Langmuir, 2008, 24, 7421 CrossRef CAS
.
- H. Kumari, S. E. Armitage, S. R. Kilane, K. K. Damodaran, S. R. Kennedy, J. L. Atwood and J. W. Steed, Soft Matter, 2015, 11, 8471 RSC
.
- P. Ravarino, N. D. Domenico, M. Barbalinardo, D. Faccio, G. Falini, D. Giuri and C. Tomasini, Gels, 2022, 8, 98 CrossRef CAS PubMed
.
- P. Ravarino, D. Giuri, D. Faccio and C. Tomasini, Gels, 2021, 7, 43 CrossRef CAS PubMed
.
- D. M. Ryan, S. B. Anderson, F. T. Senguen, R. E. Youngman and B. L. Nilsson, Soft Matter, 2010, 6, 475 RSC
.
- D. M. Ryan, S. B. Anderson and B. L. Nilsson, Soft Matter, 2010, 6, 3220 RSC
.
- H. Miyajima, M. C. Z. Kasuya, A. D. Guerzo, J.-M. Vincent and K. Hatanaka, J. Fluorine Chem., 2018, 205, 30 CrossRef CAS
.
- G. R. Desiraju, P. S. Ho, L. Kloo, A. C. Legon, R. Marquadt, P. Metrangolo, P. Politzer, G. Resnati and K. Rissanen, Pure Appl. Chem., 2013, 85, 1711 CrossRef CAS
.
- A. Bertolani, L. Pirrie, L. Stefan, N. Houbenov, J. S. Haataja, L. Catalano, G. Terraneo, G. Giancane, L. Valli, R. Milani, O. Ikkala, G. Resnati and P. Metrangolo, Nat. Commun., 2015, 6(7574), 1 Search PubMed
.
- A. Bertolani, A. Pizzi, L. Pirrie, L. Gazzera, G. Morra, M. Meli, G. Colombo, A. Genoni, G. Cavallo, G. Terraneo and P. Metrangolo, Chem. − Eur. J., 2017, 23, 2051 CrossRef CAS
.
- J. Morris, J. Bietsch, K. Bashaw and G. Wang, Gels, 2021, 7, 24 CrossRef CAS PubMed
.
- N. Basu, A. Chakraborty and R. Ghosh, Gels, 2018, 4, 52 CrossRef PubMed
.
- S. Datta and S. Bhattacharya, Chem. Soc. Rev., 2015, 44, 5596 RSC
.
- V. Singh, V. Lalitha, C. U. Maheswari, V. Sridharan, D. Pradhan, S. Batra and S. Nagarajan, ACS Omega, 2024, 9, 5695–5705 CrossRef CAS
.
- K. Fan, X. Wang, X. Wang, H. Yang, G. Han, L. Zhou and S. Song, RSC Adv., 2020, 10, 37080 RSC
.
- K. Soudarajan and T. M. Das, Carbohydr. Res., 2019, 481, 60 CrossRef
.
- B. P. Krishnan and K. M. Sureshan, Chem. − Asian J., 2017, 13, 187 CrossRef PubMed
.
- A. M. Vibhute, V. Muvvala and K. M. Sureshan, Angew. Chem., Int. Ed., 2016, 55, 7782 CrossRef CAS
.
- J. Bietsch, L. Baker, A. Duffney, A. Mao, M. Foutz, C. Ackermann and G. Wang, Gels, 2023, 9, 445 CrossRef CAS
.
- M. Khan, S. Das, A. Roy and S. Roy, Langmuir, 2023, 39, 899 CrossRef CAS
.
- P. Sharma and G. Wang, Gels, 2022, 8, 191 CrossRef CAS PubMed
.
- K. P. C. Sekhar, D. K. Swain, S. A. Holey, S. Bojja and R. Nayak, Langmuir, 2020, 36, 3080 CrossRef CAS
.
- R. Oosumi, M. Ikeda, A. Ito, M. Izumi and R. Ochi, Soft Matter, 2020, 16, 7274 RSC
.
- J. Morris, P. Kozlowski and G. Wang, Langmuir, 2019, 35, 14639 CrossRef CAS
.
- A. Chalard, L. Vaysse, P. Joseph, L. Malaquin, S. Souleille, B. Lonetti, J. C. Sol, I. Loubinoux and J. Fitremann, ACS Appl. Mater. Interfaces, 2018, 10, 17004 CrossRef CAS PubMed
.
- Y. S. Prasad, B. Saritha, A. Tamizhanban, K. Lalitha, S. Kabilan, C. U. Maheswari, V. Sridharan and S. Nagarajan, RSC Adv., 2018, 8, 37136 RSC
.
- C. D. A. León, G. A. Echeverría, O. E. Piro, S. E. Ulic, J. L. Jios, J. A. Pereañez, I. C. H. Castañeda and H. Pérez, New J. Chem., 2017, 41, 14659 RSC
.
- X. Guan, K. Fan, T. Gao, A. Ma, B. Zhang and J. A. Song, Chem. Commun., 2016, 52, 962 RSC
.
- T. Tsuzuki, M. Kabumoto, H. Arakawa and M. Ikeda, Org. Biomol. Chem., 2017, 15, 4595 RSC
.
-
(a) Y. Bai, P. Nasr, G. King, J. W. Reid, A. F. G. Leontowich, M. G. Corradini, R. G. Weiss, F.-I. Auzanneau and M. A. Rogers, Nanoscale, 2023, 15, 16933 RSC
;
(b) T. Sutthatang, U. Wichai and S. Wangsoub, J. Met., Mater. Miner., 2013, 23(1), 1 CAS
.
- J. Liu, Z. Sun, Y. Yuan, X. Tian, X. Liu, G. Duan, Y. Yang, L. Yuan, H.-C. Lin and X. Li, ACS Appl. Mater. Interfaces, 2016, 8, 6917 CrossRef CAS
.
- J. Liu, F. Xu, Z. Sun, J. Pan, H.-C. Lin and X. Li, Soft Matter, 2016, 12, 141 RSC
.
- R. Roytam, L. Adler-Abramovich, K. S. A. Kumar, T.-C. Kuan, E. Gazit and A. Brik, Org. Biomol. Chem., 2011, 9, 5755 RSC
.
- H. P. R. Mangunuru, H. Yang and G. Wang, Chem. Commun., 2013, 49, 4489 RSC
.
- X. Li, K. Yi, J. Shi, Y. Gao, H.-C. Lin and B. Xu, J. Am. Chem. Soc., 2011, 133, 17513 CrossRef CAS
.
- J. Bietsch, M. Olson and G. Wang, Gels, 2021, 7(3), 134 CrossRef CAS PubMed
.
- Rajkamal, D. Chatterjee, A. Paul, S. Banerjee and S. Yadav, Chem. Commun., 2014, 50, 12131 RSC
.
- Rajkamal, N. P. Pathak, D. Chatterjee, A. Paul and S. Yadav, RSC Adv., 2016, 6, 92225 RSC
.
- Rajkamal, N. P. Pathak, T. Halder, S. Dhara and S. Yadav, Chem. − Eur. J., 2017, 23, 11323 CrossRef CAS PubMed
.
- N. P. Pathak, Rajkamal and S. Yadav, Chem. Commun., 2020, 56, 2999 RSC
.
- N. P. Pathak, A. Sengupta and S. Yadav, Mater. Adv., 2022, 3, 3906 RSC
.
-
(a) F. Neese, Wiley Interdiscip. Rev.: Comput. Mol. Sci., 2012, 2, 73 CAS
;
(b) F. Neese, Wiley Interdiscip. Rev.: Comput. Mol. Sci., 2022, 12, e1606 Search PubMed
;
(c) E. Caldeweyher, C. Bannwarth and S. Grimme, J. Chem. Phys., 2017, 147, 034112 CrossRef
;
(d) B. A. Helmich-Paris, J. Chem. Phys., 2021, 154, 164104 CrossRef CAS PubMed
.
-
(a) A. D. Becke, Phys. Rev. A: At., Mol., Opt. Phys., 1988, 38, 3098 CrossRef CAS PubMed
;
(b) C. Lee, W. Yang and R. G. Parr, Phys. Rev. B: Condens. Matter Mater. Phys., 1988, 37, 785 CrossRef CAS
.
- M. D. Hanwell, D. E. Curtis, D. C. Lonie, T. Vandermeersch, E. Zurek and G. R. Hutchison, J. Cheminf., 2012, 4, 17 CAS
.
-
(a) F. Weigend and R. Ahlrichs, Phys. Chem. Chem. Phys., 2005, 7, 3297 RSC
;
(b) F. Weigend, Phys. Chem. Chem. Phys., 2006, 8, 1057 RSC
.
-
G. A. Zhurko, Chemcraft – graphical program for visualization of quantum chemistry computations. Ivanovo, Russia, 2005, https://chemcraftprog.com.
- B. J. C. Cabral, R. C. Guedes, R. S. Pai-Panadiker and C. A. Castro, Phys. Chem. Chem. Phys., 2001, 3, 4200–4207 RSC
.
Footnote |
† Electronic supplementary information (ESI) available: Synthetic procedures and characterization data of 3a–e, additional figures, tables, characterization data for new compounds and 1H and 13C NMR spectra are available. See DOI: https://doi.org/10.1039/d4sm00521j |
|
This journal is © The Royal Society of Chemistry 2024 |