DOI:
10.1039/D4SM00270A
(Paper)
Soft Matter, 2024,
20, 4859-4867
Polymerisation of twist-bend nematic textures for electro-optical applications†
Received
29th February 2024
, Accepted 29th April 2024
First published on 7th May 2024
Abstract
Polymer-stabilised liquid crystals (PSLCs) have recently been used to maintain the focal conic domains (FCDs) typical of the smectic A phase in the nematic phase for smart window applications. The newly discovered twist-bend nematic phase of bent-shaped dimers also exhibits FCDs due to its pseudo-layered structure. The variety of topological defects in the NTB phase is arguably even greater than in the smectic A phase, but the NTB phase is often metastable and usually crystallises at room temperature, which hinders its use in electro-optical applications. Here we show how different textures (FCDs, rope-like texture, double helices) of the NTB phase can be polymerised and then maintained in the nematic phase, at room temperature. This allows us to combine in PSLCs the optical properties of these defects, the thermal stability of the nematic phase and its reversible response to an electric field. We also show that the polymerised FCDs of the NTB phase could be used in smart glass applications and that the polymerised rope-like texture could be of interest for optical modulators and beam steering. In addition, the polymerisation of double helices could help to better understand their formation and structure in the NTB phase. More fundamentally, our work shows that despite the lack of density modulation, the textures of the NTB phase, thanks to its periodic character, can be exploited in the same way as those of the smectic A phase.
Introduction
The development of “smart” materials whose properties can be modulated by the application of an external stimulus (electric field, UV light, temperature etc.) is of great industrial interest.1,2 Such materials are used, for example, to make smart windows that change from a scattering state to a transparent state when an electric field is applied. Liquid crystals are very good candidates for this type of application, and several concepts have been proposed to achieve this property. The most mature technology, called “polymer dispersed liquid crystal” (PDLC)3–5 and already on the market, consists of dispersing nematic (N) droplets in a polymer matrix.6 Its implementation on an industrial scale is well mastered, but the transparent state is not perfectly clear since a residual haze is observed when the direction of observation is not at normal incidence. Other concepts have been proposed in the literature, some involving an electrodynamic instability,7 others involving another type of liquid crystal – polymer composites called “polymer stabilised liquid crystals” (PSLCs).8 PSLCs consist of a polymer network in which the liquid crystal is confined and have found a wide range of applications such as the stabilisation of blue phases9 and smart windows based on nematic10 or cholesteric phases.11 The main differences between PDLCs and PSLCs are the amount of polymer (about 70 wt% for PDLCs vs. about 5 wt% for PSLCs) and the state of the polymer matrix (bulk vs. network).12
An original strategy (Fig. 1) has recently been proposed to make smart windows.13,14 The idea is to combine the light scattering power of the focal conic domain (FCD) defects of the smectic A (SmA) phase with the reversibility of the response to an electric field typical of the nematic phase (Freedericksz transition).15 A PSLC material is prepared by using the smectic A defects as a template for the polymer network. Then, when the sample is heated in the nematic phase, the smectic A defects (and the scattering properties) are retained by the polymer network, and the liquid crystal can easily and reversibly be aligned by the electric field, resulting in a transparent state.
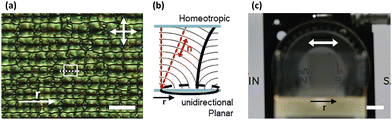 |
| Fig. 1 (a) Image in polarised-light microscopy in transmission of EHFCDs in an 8CB sample in a 10 μm thick cell, under hybrid anchoring conditions, between crossed polarisers. The white box encloses a single EHFCD. Scale bar: 20 μm. (b) Schematic representation of the structure of an EHFCD in its median plane of symmetry (side cut along the dotted line). The thick lines represent the singular lines (ellipse and hyperbola) and the thin lines the smectic layers. The red solid ellipsoids symbolize rod-like mesogens with different orientations of the director n. (c) Photograph of the 8CB cell as observed in a light booth using a D65 illuminant. A linear polarizer (white double-headed arrow) was set between the cell and the background (cell-background distance: 16 cm) and parallel to the rubbing axis r. Scale bar: 1 cm. Reproduced with permission from ref. 13. | |
From a different perspective, in the absence of polymer, the FCD defects16,17 that occur in thin films of smectic A phases under hybrid anchoring (i.e. homeotropic on one side and planar on the other) have been exploited for various types of optical applications, such as lenses,18 diffraction gratings,19 and nanoparticles clustering arrays.20 FCD defects directly result from the layered structure of the phase because the periodicity of the layers prohibits twist and bend deformations of the director.21 Therefore, in order to satisfy the hybrid anchoring conditions, the liquid crystal layers bend and wrap around singular lines taking the form of ellipses and confocal hyberbolas,22 giving rise to elliptic-hyperbolic FCDs (EHFCDs). Since FCD defects occur due to the presence of a periodic structure, whatever its nature, they are observed not only in the case of a density modulation (as in smectic phases) but also in the case of an orientation modulation (as in cholesteric phases).15
The newly discovered twist-bend nematic (NTB) phase, which is often observed with LC bent-shaped dimers, also has a periodic organisation.23 Due to a spontaneous bend of the director field, the NTB phase has a heliconical structure (Fig. 2), with doubly-degenerate chirality (i.e. with both left- and right-handed domains). Unlike the cholesteric phase, the period of the helical modulation of the director is quite short, about 10 nm,24 which is not very different from the typical smectic periods (about 3 nm15). Therefore, we show here that the topological defects of the NTB phase can be exploited to create a device that scatters light at rest and becomes transparent when submitted to an electric field, thus extending the concept of the smart window prototype (Fig. 1) described above13 to another periodic phase, namely the NTB phase.
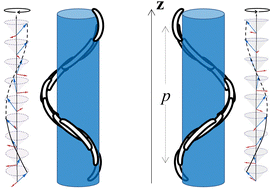 |
| Fig. 2 Heliconical structure of the NTB phase. The director of the bent-shaped molecules is on average parallel to their long axis (blue arrow). It rotates equally clock-wise or counter clock-wise and follows a conical helix with pitch p. | |
Another advantage of the NTB phase is its wide variety of textures. Indeed, FCD defects,23 stripe patterns25 and double helices26 have already been reported for this phase. In smectics, the structure of double helices has been explained in terms of screw dislocations of giant Burgers vector.22 In this model, the double helix represents disclinations of the director and the smectic layers are equally spaced on an helicoid (Fig. 3). In SmA and SmA* phases, the director coincides with the optic axis so that its singularities should be readily observable using a polarising microscope. Previous studies of the optical properties of the rope and stripe textures have shown that they could be of great interest for electro-optic modulators and beam steering applications.27 We also report here how, by polymerising the NTB phase, we have been able to make these optical properties electrically tunable.
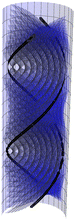 |
| Fig. 3 Schematic representation of the NTB pseudo-layers in double helices. The layers are equidistantly nested onto a ruled helicoid. The thick black lines represent the singular lines. | |
Materials and methods
Liquid crystal formulation
The liquid crystal with a twist-bend nematic phase chosen for this study is the classical compound CB7CB (4′,4′′-(heptane-1,7-diyl)dibiphenyl-4-carbonitrile, Fig. 4a). It was supplied by Synthon Chemicals (ST06137, CAS number: 149430-73-9) and purified by recrystallisation in methanol. To lower the temperature of the N/NTB phase transition, CB7CB was mixed with 8CB (4-octyl-4′-cyanobiphenyl, CAS number: 52709-84-9, Fig. 4b).28 8CB was supplied by Tokyo Chemical Industry. The mesogenic monomer 1,4-bis-[4-(3-acryloyloxypropyloxy)benzoyloxy]-2-methylbenzene (ST03021, Synthon Chemicals, Fig. 4c) and the photoinitiator 2,2-dimethoxy-2-phenylacetophenone (DMPA, Tokyo Chemical Industry, Fig. 4d) were added to the liquid crystal mixture to polymerise the system. Toluene was added to homogenise the mixture (liquid crystal/toluene mass ratio ≈1
:
1) and then evaporated at 60 °C within 3 hours. The phase transition temperatures of these compounds and their mixtures are given in Table 1.
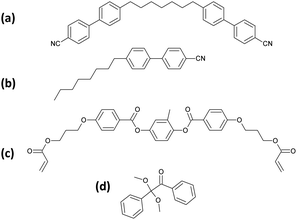 |
| Fig. 4 Compounds used in this study: (a) CB7CB, (b) 8CB, (c) ST03021, (d) DMPA. | |
Table 1 Phase transition temperatures of the compounds and mixtures studied
|
T(NTB–N) (°C) |
T(N–I) (°C) |
8CB |
— |
39.6 |
CB7CB |
102.5 |
114.9 |
ST03021 |
— |
126.0 |
CB7CB/8CB (60 : 40) |
43.0 |
70.2 |
CB7CB/8CB (60 : 40) with 5 wt% ST03021 and 0.15 wt% DMPA |
After polymerisation: 42.8 |
After polymerisation: 72.2 |
CB7CB/8CB (40 : 60) |
13.0 |
54.8 |
CB7CB/8CB (40 : 60) with 5 wt% ST03021 and 0.15 wt% DMPA |
After polymerisation: 13.2 |
After polymerisation: 59.4 |
Sample preparation
Electro-optical cells (EHC KSRO-10/B111P6NSS05, KSRP-03/B111P6NSS05, KSRT-05/D611P6NSS05) were filled with the mixtures described above, in the isotropic phase at 90 °C. Three types of samples were prepared (Table 2). Specifications of chemical composition, cell thickness, anchoring, thermal treatment, and polymerisation conditions are given in Table 2. The samples were cooled down using a hot stage (hotstage LTS 420E, Linkam) and polymerised using a UV lamp (Omnicure LX180, Lumen dynamics). The light power was set to 50 mW and the light source positioned at 11 cm from the sample.
Table 2 Sample characteristics
Sample type |
Type 1 |
Type 2 |
Type 3 |
CB7CB/8CB |
60 : 40 |
40 : 60 |
40 : 60 |
Cell thickness |
10 μm |
3 μm |
5 μm |
Anchoring |
Planar |
Planar antiparallel |
90° twisted cell |
Thermal treatment |
Quenching from isotropic phase to NTB phase |
Cycles between 0 °C and 10 °C at 10 °C min−1 |
Cycles between −5 °C and 11 °C at 5 °C min−1 |
Polymerisation conditions |
35 °C |
−10 °C |
−10 °C |
10 minutes |
10 minutes |
10 minutes |
365 nm |
365 nm |
365 nm |
15 mW cm−2 |
15 mW cm−2 |
15 mW cm−2 |
Texture |
Focal conic domains |
Rope-like texture |
Double helices |
Sample characterisation
Texture observation.
Textures were observed with a polarised-light optical microscope (POM, Nikon Eclipse LV100N POL, Japan), between crossed polarisers, with a 20× objective (Nikon, 20×, NA: 0.40, WD 19 mm). Images were taken using a CCD camera (Microvision CCD-2Mc). The period of the defect lattice was measured by Fourier transform of these images with a python program.
Phase transition temperatures.
The phase transition temperatures of pure compounds and their mixtures without monomer were measured by differential scanning calorimetry (DSC Discovery, TA Instruments). A 2 °C min−1 rate was applied during the DSC cycle and the average of the start and end values of each peak was taken as the phase transition temperature.
The phase transition temperatures of mixtures containing monomers were measured directly in the cells under the microscope on cooling at 1 °C min−1. For this purpose, images of the samples between crossed polarisers were taken every 0.1 °C. The intensity of each image was calculated by summing the values of all the pixels. The temperatures of the maximum transmitted intensity variations were taken as the phase transition temperatures.
Electric-field response.
Electric fields were applied using a programmable AC power supply (ITECH IT-M7700, MB Electronique). A sinusoidal AC voltage (f = 1 kHz) of variable amplitude was applied to the samples.
The evolution of the microscopy images with applied voltage was characterised by calculating the intensity of the images, as described in the previous paragraph.
Response times were measured using a previously described setup29 under a polarising microscope (Leitz Ortholux) equipped with a photomultiplier tube (PMT). A small load resistor (1 kΩ) was chosen for the PMT anode to achieve sub-microsecond response time of the setup. The voltage drop across the load resistor was measured using a digital oscilloscope (DSO-X 2004A, Keysight).
Optical properties.
The scattering power of the samples was characterised by photographing them with a grid (period 1 mm) in the background, at 21 cm behind the samples. A polariser was placed between the sample and the grid. The images were taken with a digital camera (Canon EOS 400D, Japan, focal length 55 mm, aperture F/36, exposure time 0.8 s, ISO-1600). The distance between the sample and the camera was 60 cm. Photographs were taken in a light booth under D65 lighting conditions, which corresponds to natural midday light in Western Europe (norm ISO 11664-2). Some photographs were taken by heating the sample above room temperature using a homemade transparent hot stage. A glass plate was coated with ITO (indium tin oxide) and an electric current was applied using a temperature controller (TC-200 from Thorlabs).
Light scattering measurements were performed with an instrument (OMS4, OPTIS) equipped with three circularly polarised lasers: red (635 nm), green (520 nm), and blue (450 nm). The detector aperture was 0.2°.
Results
Generation of different textures in the NTB phase: FCDs, rope-like texture, double helices
We first discuss the very different textures observed in the NTB phase and the conditions under which they occur. Under planar anchoring, the formation of FCD or rope-like textures clearly depends on the cell thickness. Large areas of rope-like texture were mostly obtained in thin cells (3 μm), although the rope-like texture was also observed in 10 μm thick cells, but only in small areas (around 100 μm × 100 μm). The fact that ropes are more likely to appear in thin cells has already been reported.30 The period of the ropes is twice the cell thickness.25 When the temperature is lowered through the N/NTB phase transition, stripes are first observed. These stripes are thought to be due to a Helfrich-Hurault instability.31 As the temperature is lowered further, the stripes turn into ropes, where topological defects are detected as optical singularities without an analyser (Fig. SI1, ESI†).
FCDs were only observed in thick samples (10 μm) where this texture generally competes with the rope-like texture. One way to avoid the latter and grow the former over several square millimetres is to cross the N/NTB phase transition rapidly (1 °C min−1). This is probably because the instability does not have enough time to grow. However, FCDs were not obtained for all CB7CB:8CB mixtures. This could be due to the variation of elastic constants or transition enthalpy with composition.28
Double helices were only observed in twisted cells. Depending on the sign of the twist, two orientations of DH were observed. In both cases, the axis of the DHs lies at 45° to the two rubbing axes. Interestingly, the DHs do not appear immediately upon crossing the N/NTB phase transition, regardless of the temperature ramp. Temperature cycles in the NTB phase were necessary to first generate disclinations oriented at 45° to the rubbing axis and then to grow the DHs from these disclinations (Fig. SI2, ESI†). Double helices have already been observed in SmA and SmA* phases32,33 and, like FCDs, they appear to keep the layer thickness constant.22,32 Again, this reveals a deep analogy between the SmA and NTB phases.
Type 1 samples: EHFCD lattice
In POM, type 1 PSLC samples (thick cell, see Table 2) show in the NTB phase a texture that looks like a lattice of FCDs (Fig. 5). These defects are approximately 5 μm in diameter (Fig. SI3, ESI†). The FCD lattice is perfectly preserved when the sample is heated to the nematic phase (58 °C) and therefore the cell strongly scatters light, as expected (Fig. 5).
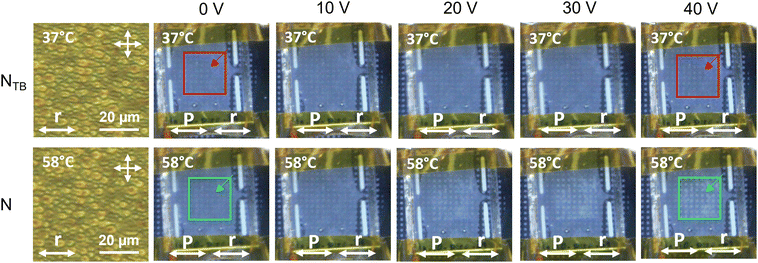 |
| Fig. 5 Left: POM images of a type 1 sample (37 °C: NTB phase, top row; 58 °C: N phase, bottom row). Right: Series of images illustrating the effect of the electric field on the scattering power of the cell. P represents the polariser axis and r represents the rubbing axis. The electric field is applied in the area enclosed by the red or green squares. In the NTB phase (top row, 37 °C), no change in the scattering power is observed as the electric field increases: the grid is as blurred at 0 V as it is at 40 V (red arrows in red squares). In the N phase (bottom row, 58 °C), a decrease of the scattering power is observed as the electric field increases: the background grid is more visible at 40 V than at 0 V (green arrows in green squares). Photographs taken under the conditions described in Materials and methods. | |
To quantify the scattering power, we define the quantity α = (1 − I1,s/I1,ref) × 100, where I1,s is the intensity of the first harmonic of the Fourier transform of the photograph of the sample with a grid in the background and I1,ref is the first harmonic of the Fourier transform of the photograph of the grid without any sample. There was no significant difference in α measurements between the NTB and N phases: α = 91.5% at 37 °C and 90.5% at 58 °C. However, a strong variation of α with polariser orientation was observed in both phases (Fig. SI4, ESI†). When an electric field was applied perpendicularly to the cell plates, at 37 °C in the NTB phase, the scattering power decreased slightly to 89.9% at 40 V. In contrast, when the sample was submitted to the same procedure, but at 58 °C in the N phase, the grid became visible (Fig. 5), the sample remained homogeneous, and the scattering power decreased much more: from 90.5% at 0 V to 68.6% at 40 V. (The detailed dependence of α on voltage is shown in the ESI,† in Fig. SI5.) This is probably because the NTB phase, being more organized, is more difficult to align along the electric field than the N phase.
The switch-off times, 4.4 ± 0.2 ms at 35 °C (NTB) and 2.0 ± 0.1 ms at 60 °C (N), are rather fast in both phases. As in the SmA phase, the reorientation under field of the helix axis in the NTB phase does not occur because it would involve the formation and migration of a large density of defects.34
Although the scattering power in the on-state needs to be further reduced and the operating temperature lowered to room temperature, this experiment shows that the approach to designing smart windows previously developed for the SmA phase can indeed be extended to the NTB phase.
Type 2 samples: rope-like texture
Polymerisation and electric-field effect.
The type 2 samples, in thin cells (3 μm, see Table 2) with planar anchoring, showed large areas of rope-like texture at 10 °C (Fig. 6a). This texture is typical of the NTB phase.23 The period, 2.8 μm, of the images was measured by Fourier transform (Fig. SI6, ESI†). However, this period is actually half of the real period of the texture since dislocations with a Burger vector of 2 were systematically detected (Fig. SI7, ESI†). Moreover, a chevron-like structure in the stripes is barely visible on close inspection of the POM images, which shows that the real period is twice the value obtained by the Fourier transform.31,35
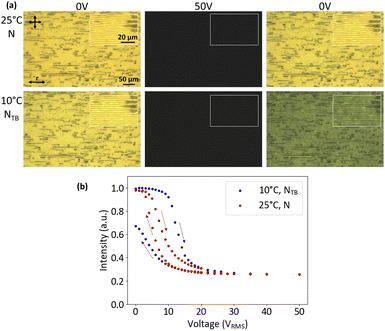 |
| Fig. 6 (a) POM images of a type 2 sample between crossed polarisers in the NTB phase (10 °C, bottom row) and in the N phase (25 °C, top row), and the effect of an electric field on the texture. In the upper left image, r represents the rubbing axis, which is the same for all photographs. (b) Intensity of POM images as a function of voltage. | |
Most importantly, the rope-like texture of the NTB phase was retained in the N phase thanks to polymerisation. When an electric field was applied perpendicularly to the cell surface in the polymerized NTB phase (10 °C), the director realigned along the electric field direction but it only partially relaxed to the previous state when the field was lowered (Fig. 6b). When the electric field was applied in the N phase (25 °C), the director aligned along the electric field direction, but, in contrast with the NTB phase, it perfectly relaxed to the previous state. In this configuration, the electro-optical response is similar to the usual Freedericksz transition,15 but without a sharp threshold.
(Due to the strong zenithal and azimuthal variations of the director orientations, in the absence of field, and to the director anchoring on the fine structures of the polymer network, fitting the electro-optic curve is presently out of reach.) We then choose as criterion for the threshold voltage the value of the inflexion point of the electro-optical curve: 7 VRMS at 25 °C and 12 VRMS at 10 °C. These threshold voltages are lower than those reported for PDLCs (17 VRMS6), PSLCs (around 20 VRMS36) or for polymerised focal conic domains in smectic A phases: we performed experiments showing that 90% of the on-state haze is achieved at about 20 VRMS.
In fact, in both phases, the type 2 sample was almost perfectly aligned along the field at 20 VRMS (Fig. 6b). Although the field alignment process was reversible in the N phase, a small hysteresis was observed. The light intensity is decreased by 75%. The contrast between the on-state and the off-state can be compared with the PDLC and PSLC literature, although authors generally directly study the scattering properties as a function of voltage: for PDLCs, the specular transmitted light increases from 0% in the off-state to 90% in the on-state,6 for PSLCs the specular transmitted light decreases by 45% when the voltage increased36 and for polymerised FCDs in SmA, the haze decreases from 50% to 25% when the voltage is applied. The switch-off time was 5.2 ± 0.8 ms, which is in agreement with the PSLC literature.37 Decay times of about 1 ms were reported for PDLCs (depending on the droplet size) and about 10 ms were reported for PSLCs and polymerised FCDs.13
Scattering properties of the polymerised rope-like texture.
When a type 2 sample, in the polymerised N phase at room temperature, was irradiated with a laser beam, up to 7 orders of light diffraction were observed (Fig. SI8, ESI†). The grating period was calculated using the formula: a = kλ/sin
θk, where k is the diffraction order, λ is the wavelength of the laser beam, and θk is the angle between the direct and diffracted beams. The result obtained with the green laser was 5.54 ± 0.07 μm, which is in good agreement with the previous measurement on the POM image. Note that, as expected for a diffraction phenomenon, the period does not depend on the wavelength of the laser (Fig. SI8, ESI†). It is also independent of the voltage (Fig. SI9, ESI†). The diffraction efficiency is defined as DEi = Ii/I0, where Ii is the intensity of the i-th diffracted peak and I0 is the intensity of the incident beam.38 Because the diffraction peaks are broad (i.e. beyond the instrumental resolution), their intensity was integrated to calculate the DE. The peak width could be due to the poor regularity of the defect lattice as a similar peak broadening was observed in the Fourier transform of the microscopy images (Fig. SI6, ESI†). DE1 decreased by an order of magnitude (from 0.14 to 0.015) as the voltage increased from 0 V to 20 V (Fig. 7).
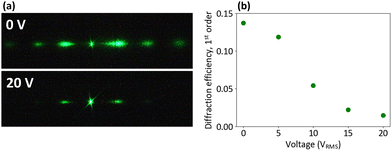 |
| Fig. 7 (a) Photographs of the diffraction pattern at 0 V and 20 V of a green laser (520 nm) by a type 2 sample. (b) Voltage dependence of the diffraction efficiency of the first diffraction order. | |
Type 3 samples: double helices
In the NTB phase, the textures of the type 3 samples (in twisted cells, see Table 2) showed double helices (DHs) oriented at 45° to the rubbing axis (Fig. 8a). The typical distance between the DHs is 10.5 μm and the pitch of the DH helix is 11 μm (Fig. SI10, ESI†). Most importantly, when the sample was heated to the N phase (25 °C), the texture was retained, probably due to the polymer network. In both phases, when an electric field was applied perpendicularly to the cell plates, the director aligned along the electric field direction above a certain voltage: the inflexion points of the electro-optical curves (Fig. 8b) were measured at 10 V at 25 °C and 23 V at 10 °C. At 50 V, the textures were not completely dark, indicating that the director was not yet fully aligned along the field direction. The transition was reversible in the N phase, but not in the NTB phase (Fig. 8b). The switch-off time was 5.8 ± 0.6 ms, a value quite similar to those of the previously described samples.
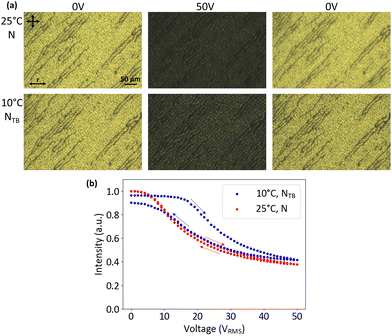 |
| Fig. 8 (a) POM images of a type 3 sample between crossed polarisers in the NTB phase (10 °C, bottom row) and the N phase (25 °C, top row), and the effect of an electric field on the texture. In the upper left image, r represents the rubbing axis, which is the same for all photographs. (b) Intensity of POM images as a function of voltage. | |
Type 3 samples also diffract light, but only a few diffraction orders were visible (Fig. SI11, ESI†).
Discussion
Formulation work was required to maintain the NTB defects in the nematic phase by polymerisation. If too much monomer (≥12 wt%) is added to the liquid crystal, the mixture remains inhomogeneous, even when the sample is heated in the isotropic phase or toluene is added. The cell is difficult to fill by capillary action, even when the sample is in the isotropic phase. This is probably because the solubility limit of the monomer has been exceeded. On the contrary, if the amount of monomer is too low (≤2 wt%), the NTB defects are not maintained in the nematic phase, although the polymer network modifies the texture of the nematic phase (Fig. SI12, ESI†). In contrast, for SmA systems, 2 wt% of monomer is sufficient to maintain the defects. A similar threshold was also reported in the N phase.39 Several hypotheses come to mind to explain this difference in threshold concentration between the NTB and SmA phases. First, the defects of the NTB phase do not have exactly the same structure as that of the focal conic domains of the SmA phase since the singular lines do not have precisely the same shape and the liquid crystal distortions are slightly different. Templating the FCD, rope-like and double-helices textures in the NTB phase may be more difficult than the FCD texture of the SmA phase due to these structural differences. However, a higher monomer concentration is required in the NTB phase, regardless of the defect type, which casts doubt on this hypothesis. Another explanation would be that the polymer network doesn’t have the same morphology in the SmA and the NTB phases. At constant monomer mass fraction, the number of polymer fibres per unit volume could be higher in the SmA phase than in the NTB phase. The contact surface between the polymer fibres and the liquid crystal would be higher in the SmA phase and the defects would then be better maintained in the SmA phase. These differences in morphology could be due to a different phase separation process during the polymerisation leading to thicker fibres in the NTB phase than in the SmA phase. A third explanation would be related to the type of anchoring. In both cases, defect stabilisation is achieved by anchoring of the liquid crystal molecules on the polymer fibres. In the NTB phase, due to the lack of positional order, only the molecular orientation is memorised at the polymer – liquid crystal interface. In the SmA phase, due to the long-range positional order, the position of the smectic layers is also memorised during the polymerisation process, which makes the anchoring much more effective and hence the defect stabilisation.
Our work shows that, thanks to the polymer network, the different textures (focal conic domains, ropes, double helices) of the NTB phase can be maintained when the sample is heated to the nematic phase. Two examples of polymerisation of the rope-like texture in the NTB phase have already been reported.35,40 This approach was first proposed by Panov et al. to better understand the rope-like texture. Using scanning electron microscopy images of the polymer network, after washing out the liquid crystal, Panov et al. proposed a ‘fish-bone’ organisation. Trbejovic et al. observed similar structures and proposed that the helical axis follows the rubbing axis and the polymer strands follow the nematic director. They also argue that the polymer network stabilises the NTB phase at room temperature, preventing sample crystallisation for months and making the NTB phase metastable. Our approach is different in that we keep the NTB textures in the nematic phase. The anchoring strength of the liquid crystal on the polymer fibrils is probably strong enough to make the nematic director follow the topology of the polymer network, which reflects the NTB textures.41,42 Moreover, our samples are completely stable at room temperature since they are in the nematic phase and not in the NTB phase, although they exhibit typical NTB textures instead of nematic ones.
Our work also shows that the director in the three samples can be aligned by an electric field in both the NTB and nematic phases, but the alignment was only reversible in the latter. This result was expected for the nematic phase, since our experimental conditions are reminiscent of the Freedericksz transition.15 The threshold voltage, 1.2 V, was measured in the N phase by Aouini et al.43 for the CB7CB/8CB (42
:
58) mixture, whereas we only observed a response at around 3 V for the two textures (DH, rope-like textures) at the same reduced temperature (Tr = Texp/TI–N, in this case Tr = 0.9). This difference is probably due to the anchoring of the liquid crystal on the polymer strands.
Borshch et al. have discussed the case of the analogue of the Freedericksz transition in the NTB phase and suggested that the liquid crystal rotates by blocks in this pseudo-layered phase.44 Moreover, Challa et al. showed that the stripe texture could be suppressed by applying a magnetic field, but the stripes did not reappear when the field was removed.31 Here we have demonstrated a method to make this transformation reversible. Indeed, when the sample is in the nematic phase, it retains the rope-like texture (or the FCD or DH texture) but it can be reversibly aligned by an electric field. We noticed a difference in the threshold voltage according to the texture as it is around 7 V for the stripes, but around 10 V for the DH. This confirms that the alignment mechanism here is very different from the Freedericksz transition which is based on a competition between the bulk alignment of the liquid crystal along the field and the anchoring torque at the cell surfaces. In polymerised samples, the anchoring torque acts at the fibres which are distributed throughout the volume of the cell.
Our measurements of the switch-off time show that it does not seem to depend very much on either the cell thickness or the texture (Fig. SI13, type 2 and 3 samples, ESI†). The two samples have the same chemical composition and the measurements were made at the same temperature (25 °C, nematic phase). This behaviour is indeed unusual. In the nematic phase, for the twist Freedericksz transition, the decay time τD is given by: τD = (γ1d2)/(K22π2) (where γ1 is the rotational viscosity, d is the cell thickness, and K22 is the twist elastic constant).45 In our case, τD does not depend on the cell thickness because the characteristic distance to be considered is probably the mesh size of the polymer network. In addition, as expected, the switch-off time decreases with increasing temperature (Fig. SI14, ESI†), probably due to the decrease in viscosity.
The three types of samples scatter light. Type 1 samples scatter light strongly and therefore hide the test grid (Fig. 5), suggesting possible applications in smart windows for privacy control. They are more responsive to the electric field in the N phase than in the NTB phase. This system is very similar to the prototype of Boniello et al.,13 which further supports the analogy between the smectic A and NTB phases. High levels of scattering power are obtained in both cases (type 1 samples and Boniello's samples). The two samples have the same thickness (10 μm) and the same type of texture (FCDs). The scattering properties are probably determined by both the textures and the sample thickness. However, the transparent state of type 1 samples is less clear than that of Boniello's samples. This could be due to a larger amount of polymer, 5 wt%, in type 1 samples compared to 2 wt% in Boniello's samples. The propagation of light in these samples is not trivial and is currently being investigated in our laboratories.
Type 2 samples are also interesting because their texture is quite well ordered and therefore easier to study. The laser diffraction pattern of these samples is very similar to the Fourier transform of the microscope images (Fig. SI6, ESI†). The period calculated from the diffraction experiments is about twice that obtained from the microscopy images (5.54 μm versus 2.8 μm). In fact, the real period is probably 5.6 μm because the microscopy images are simply not contrasted enough to distinguish clearly the chevron pattern. Apart from this feature, the Fourier transform of the microscopy images is similar to the laser diffraction pattern. Consequently, such samples can be considered as phase gratings27 whose diffraction efficiency can be tuned by a factor of 10 with an electric field, with values similar to those reported in the literature.46 Electrically tunable gratings based on liquid crystals generally require a microstructuring step (photolithography,43,47 micropatterned mask,46 linear azo-polymers48…). Here the defects that naturally occur in the NTB phase are simply used in a low-cost bottom-up approach.
Conclusions
We obtained large domains of three types of textures in the NTB phase, namely the focal conic domains, ropes, and double helices. These three textures were polymerised to prepare PSLCs. Thanks to the polymer network, the NTB textures were retained when the system was heated to the nematic phase. The system formulation allowed us to obtain samples in the nematic phase, at room temperature, with textures (ropes and double helices) characteristic of the twist-bend nematic phase. Applying an electric field to the samples resulted in the alignment of the director along the field direction. This alignment was reversible in the nematic phase but not in the NTB phase. The optical properties of these systems were studied under an electric field. Polymerised FCDs strongly scatter light and could be used for smart glass applications in a manner similar to the defect lattices of SmA phases. Polymerised ropes diffract light and behave like phase gratings whose diffraction efficiency can be tuned by an electric field.
Further development of this work would be aimed at better understanding the haze due to light propagation in the FCD texture and the differences in the polymerisation process between the SmA and NTB phases. From another point of view, the study of the formation of double helices, a still unfamiliar type of texture, could benefit from the freezing of samples, at different stages, by polymerisation.
Author contributions
I. D., C. M. and P. D. designed and supervised the project, and C. N. M. performed the experiments and analysed the data which was interpreted by all the authors. C. N. M. prepared the figures and wrote the initial draft of the manuscript which was edited and reviewed by all the authors.
Conflicts of interest
There are no conflicts to declare.
Acknowledgements
We thank Emmanuel Garre for his help with the optical measurements as a function of temperature, Axel Fouques, Cécile Ozanam, Iryna Gozhyk, Laurent Maillaud and Christophe Blanc for helpful discussions, Claire Goldmann for the purification of CB7CB, and Frédéric Mondiot for initial discussions of this project. This work was funded by a joint PhD grant from Agence Nationale Recherche et Technologie and Saint-Gobain Research Paris.
Notes and references
- S. Nundy, A. Mesloub, B. M. Alsolami and A. Ghosh, Electrically Actuated Visible and Near-Infrared Regulating Switchable Smart Window for Energy Positive Building: A Review, J. Cleaner Prod., 2021, 301, 126854, DOI:10.1016/j.jclepro.2021.126854.
- C. M. Lampert, Large-Area Smart Glass and Integrated Photovoltaics, Sol. Energy Mater. Sol. Cells, 2003, 76(4), 489–499, DOI:10.1016/S0927-0248(02)00259-3.
- P. S. Drzaic, Polymer Dispersed Nematic Liquid Crystal for Large Area Displays and Light Valves, J. Appl. Phys., 1986, 60(6), 2142–2148 CrossRef CAS.
- J. L. West, Phase Separation of Liquid Crystals in Polymers, Mol. Cryst. Liquid Cryst. Incorporating Nonlinear Optics, 1988, 157(1), 427–441, DOI:10.1080/00268948808080247.
- H. Hakemi, Polymer-Dispersed Liquid Crystal Technology ‘Industrial Evolution and Current Market Situation, Liquid Cryst. Today, 2017, 26(3), 70–73, DOI:10.1080/1358314X.2017.1359143.
- J. W. Doane, N. A. Vaz, B.-G. Wu and S. Žumer, Field Controlled Light Scattering from Nematic Microdroplets, Appl. Phys. Lett., 1986, 48(4), 269–271, DOI:10.1063/1.96577.
- Y. Zhan, H. Lu, M. Jin and G. Zhou, Electrohydrodynamic Instabilities for Smart Window Applications, Liq. Cryst., 2020, 47(7), 977–983, DOI:10.1080/02678292.2019.1692929.
- Y. Ye, L. Guo and T. Zhong, A Review of Developments in Polymer Stabilized Liquid Crystals, Polymers, 2023, 15(13), 2962, DOI:10.3390/polym15132962.
- H. Kikuchi, M. Yokota, Y. Hisakado, H. Yang and T. Kajiyama, Polymer-Stabilized Liquid Crystal Blue Phases, Nat. Mater., 2002, 1(1), 64–68, DOI:10.1038/nmat712.
- X. Hu, X. Zhang, W. Yang, X.-F. Jiang, X. Jiang, L. T. Haan, D. de; Yuan, W. Zhao, N. Zheng and M. Jin,
et al., Stable and Scalable Smart Window Based on Polymer Stabilized Liquid Crystals, J. Appl. Polym. Sci., 2020, 137(30), 48917, DOI:10.1002/app.48917.
- X. Hu, W. Zeng, W. Yang, L. Xiao, L. T. De Haan, W. Zhao, N. Li, L. Shui and G. Zhou, Effective Electrically Tunable Infrared Reflectors Based on Polymer Stabilised Cholesteric Liquid Crystals, Liq. Cryst., 2019, 46(2), 185–192, DOI:10.1080/02678292.2018.1483038.
- I. Dierking, Polymer Network–Stabilized Liquid Crystals, Adv. Mater., 2000, 12(3), 167–181, DOI:10.1002/(SICI)1521-4095(200002)12:3
167::AID-ADMA167
3.0.CO;2-I.
- G. Boniello, V. Vilchez, E. Garre and F. Mondiot, Making Smectic Defect Patterns Electrically Reversible and Dynamically Tunable Using In Situ Polymer-Templated Nematic Liquid Crystals, Macromol. Rapid Commun., 2021, 42(11), 2100087, DOI:10.1002/marc.202100087.
-
F. Mondiot, Electrically Controllable Device Having Variable Diffusion By Liquid Crystals, And Method For Same. WO 2021/198596 A1, 2021 Search PubMed.
-
P. G. Gennes and J. de Prost, The Physics of Liquid Crystals, Clarendon Press, 1993 Search PubMed.
- L. Z. Ruan, J. R. Sambles and I. W. Stewart, Self-Organized Periodic Photonic Structure in a Nonchiral Liquid Crystal, Phys. Rev. Lett., 2003, 91(3), 033901, DOI:10.1103/PhysRevLett.91.033901.
- B. Zappone, C. Meyer, L. Bruno and E. Lacaze, Periodic Lattices of Frustrated Focal Conic Defect Domains in Smectic Liquid Crystal Films, Soft Matter, 2012, 8(16), 4318–4326, 10.1039/C2SM07207F.
- J.-B. Wu, S.-B. Wu, H.-M. Cao, Q.-M. Chen, Y.-Q. Lu and W. Hu, Electrically Tunable Microlens Array Enabled by Polymer-Stabilized Smectic Hierarchical Architectures, Adv. Opt. Mater., 2022, 10(20), 2201015, DOI:10.1002/adom.202201015.
- L.-L. Ma, M.-J. Tang, W. Hu, Z.-Q. Cui, S.-J. Ge, P. Chen, L.-J. Chen, H. Qian, L.-F. Chi and Y.-Q. Lu, Smectic Layer Origami via Preprogrammed Photoalignment, Adv. Mater., 2017, 29(15), 1606671, DOI:10.1002/adma.201606671.
- L.-L. Ma, C.-Y. Li, J.-T. Pan, Y.-E. Ji, C. Jiang, R. Zheng, Z.-Y. Wang, Y. Wang, B.-X. Li and Y.-Q. Lu, Self-Assembled Liquid Crystal Architectures for Soft Matter Photonics, Light: Sci. Appl., 2022, 11(1), 1–24, DOI:10.1038/s41377-022-00930-5.
- D. S. Kim and D. K. Yoon, Curvatures of Smectic Liquid Crystals and Their Applications, J. Information Display, 2018, 19(1), 7–23, DOI:10.1080/15980316.2017.1410500.
- Y. A. Nastishin and C. Meyer, Imperfect Defects in Smectics A, Liquid Cryst. Rev., 2023, 1–47, DOI:10.1080/21680396.2023.2181880.
- M. Cestari, S. Diez-Berart, D. A. Dunmur, A. Ferrarini, M. R. de la Fuente, D. J. B. Jackson, D. O. Lopez, G. R. Luckhurst, M. A. Perez-Jubindo and R. M. Richardson,
et al., Phase Behavior and Properties of the Liquid-Crystal Dimer 1′′,7′′-Bis(4-Cyanobiphenyl-4′-Yl) Heptane: A Twist-Bend Nematic Liquid Crystal, Phys. Rev. E: Stat., Nonlinear, Soft Matter Phys., 2011, 84(3), 031704, DOI:10.1103/PhysRevE.84.031704.
- A. Jákli, O. D. Lavrentovich and J. V. Selinger, Physics of Liquid Crystals of Bent-Shaped Molecules, Rev. Mod. Phys., 2018, 90(4), 045004, DOI:10.1103/RevModPhys.90.045004.
- V. P. Panov, M. Nagaraj, J. K. Vij, Yu. P. Panarin, A. Kohlmeier, M. G. Tamba, R. A. Lewis and G. H. Mehl, Spontaneous Periodic Deformations in Nonchiral Planar-Aligned Bimesogens with a Nematic-Nematic Transition and a Negative Elastic Constant, Phys. Rev. Lett., 2010, 105(16), 167801, DOI:10.1103/PhysRevLett.105.167801.
- M. Kleman and K. S. Krishnamurthy, Defects in the Twist-Bend Nematic Phase: Stabilities and Instabilities of Focal Conic Domains and Related Topics, Phys. Rev. E, 2018, 98(3), 032705, DOI:10.1103/PhysRevE.98.032705.
- N. Vaupotič, M. Ali, P. W. Majewski, E. Gorecka and D. Pociecha, Polarization Gratings Spontaneously Formed from a Helical Twist-Bend Nematic Phase, ChemPhysChem, 2018, 19(19), 2566–2571, DOI:10.1002/cphc.201800360.
- A. Aouini, M. Nobili, E. Chauveau, P. Dieudonné-George, G. Damême, D. Stoenescu, I. Dozov and C. Blanc, Chemical-Physical Characterization of a Binary Mixture of a Twist Bend Nematic Liquid Crystal with a Smectogen, Crystals, 2020, 10(12), 1110, DOI:10.3390/cryst10121110.
- C. Meyer, P. Davidson, D. Constantin, V. Sergan, D. Stoenescu, A. Knežević, I. Dokli, A. Lesac and I. Dozov, Freedericksz-Like Transition in a Biaxial Smectic-A Phase, Phys. Rev. X, 2021, 11(3), 031012, DOI:10.1103/PhysRevX.11.031012.
-
M. Ali, Diffraction Gratigns Formed by Bent-Core Liquid Crystals in the Twist-Bend Nematic Phase, University of Maribor, 2021 Search PubMed.
- P. K. Challa, V. Borshch, O. Parri, C. T. Imrie, S. N. Sprunt, J. T. Gleeson, O. D. Lavrentovich and A. Jákli, Twist-Bend Nematic Liquid Crystals in High Magnetic Fields, Phys. Rev. E: Stat., Nonlinear, Soft Matter Phys., 2014, 89(6), 060501, DOI:10.1103/PhysRevE.89.060501.
- C. E. Williams, Helical Disclination Lines in Smectics a, Philos. Mag., 1975, 32(2), 313–321, DOI:10.1080/14786437508219956.
- C. Meyer, C. Rabette, P. Gisse, K. Antonova and I. Dozov, DH* in Chiral Smectics under Electric Field, Eur. Phys. J. E: Soft Matter Biol. Phys., 2016, 39(7), 76, DOI:10.1140/epje/i2016-16076-7.
- C. Meyer, Nematic Twist-Bend Phase under External Constraints, Liq. Cryst., 2016, 43(13–15), 2144–2162, DOI:10.1080/02678292.2016.1204635.
- V. P. Panov, S. P. Sreenilayam, Y. P. Panarin, J. K. Vij, C. J. Welch and G. H. Mehl, Characterization of the Submicrometer Hierarchy Levels in the Twist-Bend Nematic Phase with Nanometric Helices via Photopolymerization. Explanation for the Sign Reversal in the Polar Response, Nano Lett., 2017, 17(12), 7515–7519, DOI:10.1021/acs.nanolett.7b03441.
- R. a M. Hikmet, Electrically Induced Light Scattering from Anisotropic Gels, J. Appl. Phys., 1990, 68(9), 4406–4412, DOI:10.1063/1.346190.
-
I. Dierking, Polymer-Modified Liquid Crystals, Royal Society of Chemistry, 2019 Search PubMed.
-
T. Scharf, Polarized Light in Liquid Crystals and Polymers, John Wiley & Sons, 2007 Search PubMed.
- T. Nose, S. Masuda, S. Sato, J. Li, L.-C. Chien and P. J. Bos, Effects of Low Polymer Content in a Liquid-Crystal Microlens, Opt. Lett., 1997, 22(6), 351–353, DOI:10.1364/OL.22.000351.
- N. Trbojevic, D. J. Read and M. Nagaraj, Metastable Room-Temperature Twist-Bend Nematic Phases via Photopolymerization, Phys. Rev. E, 2019, 99(6), 062704, DOI:10.1103/PhysRevE.99.062704.
- V. Sergan, T. Sergan, I. Dozov, S. Joly and R. Voss, Orientational Order Induced by a Polymer Network in the Isotropic Phase of Liquid Crystal, Phys. Rev. E, 2020, 101(5), 052705, DOI:10.1103/PhysRevE.101.052705.
- B. P. Radka, T. Lee, I. I. Smalyukh and T. J. White, The Association of Structural Chirality and Liquid Crystal Anchoring in Polymer Stabilized Cholesteric Liquid Crystals, Soft Matter, 2024, 20(8), 1815–1823, 10.1039/D3SM01558K.
-
A. Aouini, Cristaux Liquides Nématiques de Type Twist-Bend – Mécanismes de Commutation et Applications, PhD thesis, Ecole nationale supérieure Mines-Télécom Atlantique Bretagne Pays de la Loire, 2020.
- V. Borshch, Y.-K. Kim, J. Xiang, M. Gao, A. Jákli, V. P. Panov, J. K. Vij, C. T. Imrie, M. G. Tamba and G. H. Mehl,
et al., Nematic Twist-Bend Phase with Nanoscale Modulation of Molecular Orientation, Nat. Commun., 2013, 4(1), 1–8, DOI:10.1038/ncomms3635.
-
L. M. Blinov, Structure and Properties of Liquid Crystals, Springer Science & Business Media, 2010 Search PubMed.
- A. K. Ghosh, S.-W. Choi, Y. Takanishi, B. Park, K. Ishikawa, Y. Ono, J. Kawamura and H. Takezoe, Polarization-Independent Electrically Tunable Phase Grating Fabricated from Ultraviolet-Curable Liquid Crystals, J. Appl. Phys., 2004, 96(10), 5909–5911, DOI:10.1063/1.1805181.
- J. Chen, P. J. Bos, H. Vithana and D. L. Johnson, An Electro-optically Controlled Liquid Crystal Diffraction Grating, Appl. Phys. Lett., 1995, 67(18), 2588–2590, DOI:10.1063/1.115140.
- M. Hendrikx, A. P. H. J. Schenning, M. G. Debije and D. J. Broer, Light-Triggered Formation of Surface Topographies in Azo Polymers, Crystals, 2017, 7(8), 231, DOI:10.3390/cryst7080231.
|
This journal is © The Royal Society of Chemistry 2024 |