Robust magnetic energy harvesting with flexible lead-free poly(vinylidene fluoride)-Ba0.85Ca0.15Ti0.9Zr0.1O3 fibers and Metglas-based magnetoelectric composites†
Received
23rd November 2023
, Accepted 7th March 2024
First published on 21st March 2024
Abstract
The integration of magnetoelectric (ME) principles using magneto-mechano-electrical (MME) generators enables the construction of self-powered wireless sensor networks (WSNs) for mechanical energy harvesting. In this study, we propose a lead-free, flexible MME generator that incorporates poly(vinylidene fluoride) (PVDF)/Ba0.85Ca0.15Ti0.9Zr0.1O3 (BCZT) fiber composites and Metglas. This generator produces a robust output voltage even in the presence of stray magnetic fields, without requiring a magnetic bias field. We prepared flexible PVDF/BCZT fiber composites by electrospinning the components at various proportions, and a magnetostrictive Metglas layer was incorporated during the ME composite fabrication process. Under resonance conditions (50 Hz), the optimized ME composition yielded a maximum ME voltage of 472 V cm−1 Oe−1 without a magnetic DC bias field. This significant improvement is attributed to the interfacial interactions between the surface of inorganic BCZT nanoparticles and dipoles within the PVDF polymer matrix, as well as the high permeability of Metglas. Additionally, the flexible MME generator proposed in this study produced an open-circuit voltage of 14.8 V and an approximate power density of 4.7 µW cm−3 under an AC magnetic field of 10 Oe with a frequency of 50 Hz. We demonstrate that our MME device can be used to monitor the health of a muffle furnace by tapping into the magnetic field noise coming from its electronic cables. The as-developed lead-free flexible MME generator shows potential for advanced applications in self-powered WSN and energy harvesting technologies.
1. Introduction
The wide-scale applicability of the Internet of Things (IoTs) is limited by the use of conventional battery systems to power them.1–3 Generally, powering miniature IoTs with conventional batteries has several disadvantages, such as lead toxicity, limited life span and particularly, the need for their periodic replacement.4,5 To broaden the application scope of IoTs, there is a need for efficient alternative power sources to seamlessly power the IoTs without any external power sources.6,7 In this context, effective progress has been made in powering the IoTs with irregular energy sources, which are ubiquitously available in our surrounding environment.6,8 Some examples include harnessing low-frequency mechanical vibrations from human biological motions and other natural energy resources such as thermal, wind and solar energy.8,9 One such energy source is the stray magnetic field noise (less than 10 Oe at a consistent frequency of 50/60 Hz) arising from power transmission infrastructures installed in factories, buildings and transportation facilities as it is highly ubiquitous in our living environment.6,7 Usually, magnetic energy conversion in practical IoTs applications relies on conventional electromagnetic generators featuring bulky magnetic cores and a cluster of coils.7 Unfortunately, this approach leads to an impractical, cumbersome, and inefficient solution that does not meet the requirements of modern IoT applications.
Magnetoelectric (ME) materials are a class of multifunctional materials, in which long-range ferroelectric and ferromagnetic orders coexist in a single phase.10–12 A cross-coupling exists between ferroelectric and ferromagnetic mechanisms because of which polarization and magnetization can be manipulated using a magnetic field and an electric field, respectively.13–15 ME materials have a wide range of applications, such as in ME-RAM memory devices, magnetic sensors, and mechanical and low-field magnetic energy harvesters.16,17 Low-frequency magnetic noises can be effectively scavenged using the concept of magneto-mechano-electric (MME) energy conversion through a ME cantilever structure, which is operated in a resonance mode at a frequency of 50/60 Hz.5,6
MME generators generally comprise piezoelectric, magnetostrictive, and proof-mass magnetic components, in which direct elastic coupling occurs between the piezoelectric and magnetostrictive elements, and a magnetic torque will originate from magnetic stimuli.6 This process involves multiple energy conversion processes ranging from magnetic energy to mechanical energy and then into the sinusoidal form of electrical energy, which is the output. MME devices can generate electric power output in the range of a few micro-watts to a few milli-watts by sourcing from a weak magnetic noise field.7,16–19
Due to the inherent brittleness and rigidity of ceramics, ceramics-based MME devices generate very low output power, which prevents their multifaceted applicability in commercial contexts.20 Recently, MME generators were designed with lead-based materials, such as Pb (Zr,Ti)O3 (PZT), and single crystals as piezoelectric components due to their exceptional piezoelectric and electromechanical parameters when applied in the form of micro-fiber composites.6,26 However, their toxicity concerns limit their practical applications. This, in turn, has paved the way for lead-free ceramics like (Ba, Ca) (Zr, Ti) O3 (BCZT) to be implemented as piezoelectric components as their piezoelectric response (d33 ∼ 620 pC/N) is comparable to that of higher-end PZT ceramics.6 They have been incorporated in recently reported MME generators in the form of single-crystal macro-fibers and generate comparable power density output to lead-based systems.6 Given the drawbacks associated with single crystals, such as their limited range of sizes and shapes and resource-intensive and time-consuming production processes, flexible composites have emerged as the more favorable choice for various applications. This preference stems from their inherent benefits, including cost-effectiveness, adaptability to diverse shapes, robust durability, and the ability to be customized for multiple functions.36
To resolve this challenge, flexible MME generators have been fabricated with piezoelectric ceramic-based fiber composites.21,22 Piezoelectric poly(vinylidene fluoride) (PVDF) and its copolymers exhibit high electro activity, high chemical stability, and eco-friendly nature.20,22 Recently, many studies have reported the design of ferrite/ceramic–polymer (CoFe2O4–PVDF, NiFe2O4–PVDF, BCZT–PVDF)-type energy harvesters and demonstrate the harvest of electrical energy from mechanical and triboelectric energy.23,24,57 In this context, the incorporation of a ferroelectric filler into PVDF is an effective technique to improve the ferroelectric characteristics of the ceramic–polymer matrix.25,26 BCZT can be introduced into the PVDF matrix to enhance the piezoelectric properties and flexibility of the device, which in turn can improve the energy-harvesting performance. Metglas is an amorphous magnetic material, which offers high magnetoelastic coupling owing to its high permeability (µr > 10
000) and mediocre magnetostriction values (λ ∼ 44 ppm).27 It offers the advantage of the self-bias effect (non-zero αME at Hdc = 0).28 Therefore, an ME composite containing layers of flexible BCZT–PVDF fibers and a thin layer of Metglas might be a better choice for designing an efficient MME generator. In this work, a self-biased flexible PVDF-BCZT/Metglas MME generator, which demonstrates optimal device performance at its resonance condition, was fabricated. The proposed lead-free flexible magnetic energy harvester generated an open-circuit voltage of 14.8 V and an approximate power density of 4.7 µW cm−3 with a magnetic field of 10 Oe.
2. Materials and methods
2.1 Materials
All the starting chemicals and solvents were analytically pure and used without further purification. For the preparation of Ba0.85Ca0.15Ti0.9Zr0.1O3 (BCZT) powder, barium acetate (>99%, Hi-media), calcium nitrate tetrahydrate (>99%, Hi-Media), zirconium oxy-chloride octahydrate (>99%, Avra), and titanium tetra isopropoxide (>99%, SRL) were used. Acetic acid (SRL) and ethanol (China), as well as N,N-dimethylformamide (>99%, Sigma Aldrich), were used as solvents. Poly(vinylidene fluoride) (PVDF) pellets with a molecular weight of 530
000 (Sigma Aldrich) were used for preparing the polymer matrix solution.
BCZT nanoparticles were prepared using a wet-chemical (sol–gel) process reported elsewhere.30 Barium acetate and calcium nitrate were weighed according to their stoichiometric ratio and added to distilled water and acetic acid. The mixture was maintained under agitation for 60 minutes, and this solution was stirred to achieve complete dissolution. The required amounts of titanium tetra iso-propoxide and zirconium oxy-chloride octahydrate were placed in acetic acid, and ethanol was added and stirred for 30 minutes. After these two solutions were separately prepared, they were mixed and stirred at 90 °C to form a sol. Subsequently, this sol transformed into a gel. The obtained gel was dried in an oven for 12 h, and the dried gel was crushed into fine powder using a mortar. The resulting fine powder was calcined in the air at 950 °C for 2 h to obtain the pure phase BCZT powder. The optimal calcination temperature was 950 °C, as confirmed using thermo-gravimetric analysis (TGA) and further supported by X-ray diffraction (XRD) data from a previous study.70 Green pellets were prepared using a uniaxial hydraulic system at a pressure of 1.7 MPa. These pellets were then sintered at 1300 °C for a duration of 4 h to yield highly dense BCZT ceramics. For the preparation of fiber composites, the sintered BCZT pellets were crushed into fine particles using a vigorous grinding method with acetone as the medium. The calcination and sintering procedures were performed at a heating and cooling ramp rate of ±5 °C min−1.
2.2 Preparation of electrospun PVDF–BCZT fiber composites
For the preparation of electrospun fibers, PVDF pellets were dissolved in a N,N-dimethylformamide (DMF)/acetone solvent mixture (1
:
1 v/v ratio). The solution was stirred at a temperature of 60 °C for 5 h using a magnetic stirrer to obtain a clear and transparent solution. Following this step, specific proportions of sintered BCZT ceramic powders making up 5%, 10%, and 15 wt% (i.e., 0.05 g, 0.10 g, and 0.15 g of BCZT powders) were individually dispersed in the PVDF transparent solution. A sonication procedure was employed for 30 minutes to finely control the dispersion efficacy of the powders within the polymer matrix. The as-prepared solutions were then reserved for the electrospinning process. The prepared solutions were transferred into three individual 10 mL glass syringes (Hamilton) with a needle tip approximately 0.5 mm in diameter. After optimizing the operating parameters, each syringe with the loaded solution was connected to a high-voltage DC power supply set at 14 kV and the injection rate was set to 5 mL h−1. Due to the application of a high DC voltage, the significant stretching forces acting on these polymer composites led to the spontaneous formation of self-poled nanofibers. It is noteworthy that this electrical poling method has been acknowledged for its effectiveness in enhancing the piezoelectric properties of films. Electrospinning was carried out at room temperature. The resulting fiber composites were collected using commercial aluminium foils placed 15 cm away from the spinning needle.
2.3 Characterization techniques
The structural attributes of the specimens were assessed by X-ray diffraction (XRD) using an X-ray Diffractometer (XRD; RIGAKU ULTIMA-IV, Japan) equipped with Cu Kα radiation (λ = 1.54056 Å). To identify the functional groups within the samples, FTIR spectroscopy (FT-IR; PerkinElmer Frontier FT-IR/FIR spectrometry) was employed. The morphology of the samples was investigated via scanning electron microscopy (SEM; ZEISS EVO 18, Germany). The concentrations of the constituent elements were analyzed using energy-dispersive X-ray spectrometry (EDS; Aztec, Oxford instruments plc., UK). The magnetization data was recorded at room temperature using a vibrating sample magnetometer (VSM; 7400-S, LakeShore Cryotronics, Inc., Westerville, Ohio, USA). For the investigation of ferroelectric properties and leakage current characteristics, the samples were using a precision multiferroic tester (Radiant Technologies, Inc., USA). The output performance of the MME generator was assessed using a specially designed setup, which included a Helmholtz coil, a digital multimeter (GW Instek, GDM-9061) equipped with a multifunctional resistance control relay providing a range of load resistors from 100 Ω to 10 MΩ, and a digital oscilloscope (Tektronix DPO2024B, 200 MHz). The magnetic field was generated by applying an AC input to the Helmholtz coil using a function generator (GW Instek, AFG-2225, 25 MHz) and an AC power supply.
3. Results and discussion
3.1 Characteristics of the BCZT nanoparticles
Fig. 1a depicts the XRD profile obtained for the BCZT nanoparticle powder sample. Notably, no secondary or additional peaks were observed, signifying the formation of a pure perovskite phase. The prominent peaks corresponding to (100), (110), (111), (002), (211), and (210) match the JCPDS #05-0626 reference pattern.29 At ambient conditions, the splitting of the diffraction peaks of the (2 0 0)/(0 0 2) planes at 2θ ∼ 45° suggests the presence of a tetragonal phase. Furthermore, the peak observed at around 2θ ∼ 66° signifies the existence of an orthorhombic phase.30 To ascertain the structural attributes of the BCZT sample, we conducted Rietveld refinement of the acquired XRD data, employing FULLPROF software by considering tetragonal symmetry in the space group P4mm and orthorhombic symmetry in the phase group Amm2. The refined outcomes revealed the presence of the Amm2 phase at 12.9% and the P4mm phase at 87.1%, which is deemed satisfactory.30 The phase analysis results validate the existence of MPB regions in the 0.5BCZT-0.5BZT composition sample, which is in line with the report by Dash et al.31
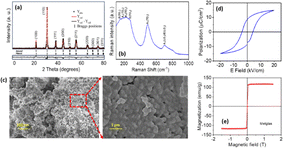 |
| Fig. 1 (a) Graphical representation of the BCZT powder using Rietveld analysis; the vertical bars denote reflection positions for both orthorhombic (O) and tetragonal (T) phases. (b) Raman spectroscopy data of the BCZT ceramic obtained at room temperature in the spectral range from 10 cm−1 to 1000 cm−1. (c) SEM micrographs of the BCZT powder calcined at 950 °C. (d) Room-temperature P–E hysteresis loops of the sintered BCZT ceramic. (e) Experimental magnetization hysteresis curves of Metglas. | |
To further investigate the MPB region and crystal symmetry, the room-temperature (RT) Raman spectrum of the BCZT sample was obtained, as presented in Fig. 1b. The characteristic Raman bands were observed, in excellent agreement with previous reports.35 Notably, the distinctive peaks of the ferroelectric long-range order in the tetragonal phase were observed in the modes B1 + E (TO + LO) and A1(LO3) + E(LO3) at around 300 and 720 cm−1, respectively. Additionally, the presence of the A1(LO) mode at approximately 200 cm−1 suggests the coexistence of orthorhombic and tetragonal phases, thus corroborating the XRD findings.35
In Fig. 1c, the SEM micrographs depict the BCZT powder. The optimized calcination process at elevated temperatures facilitates the conversion of agglomerated powder blocks into smaller grains.36 The resultant grain size was distributed within a narrower range, spanning from 40 to 100 nm, whereas, the agglomerate blocks displayed an average particle size of 62 nm.
Fig. 1d displays the polarization–electric field (P–E) hysteresis loop of a BCZT ceramic capacitor at 100 Hz at room temperature (RT). The strength of polarization in the ceramic capacitor exhibited a nonlinear relationship with the external electric field, indicating distinct ferroelectric behavior in the sol–gel-derived BCZT ceramic. The extracted values of maximum polarization (Pmax), remnant polarization, and coercive field were 14.9 µC cm−2, 7.9 µC cm−2, and 4.02 kV cm−1, respectively, at an applied electric field of 20 kV cm−1. The maximum polarization value exceeds that of the BCZT ceramic sample prepared through solid-state reactions (Pmax = 9–14 µC cm−2)37,39,40 and is comparable to those of the BCZT ceramics prepared via sol–gel (Pmax = 6.8–18.1 µC cm−2) and hydrothermal (Pmax = 8–13 µC cm−2) methods.38,41,42
To confirm the magnetostrictive properties of Metglas, we obtained a magnetization curve using a VSM by applying a DC magnetic field, Hdc, and the resulting M–H curve is shown in Fig. 1e. The sample was oriented in the longitudinal direction. Metglas reached saturation magnetization at 117 emu g−1 as Hdc was increased.43 This is attributed to the presence of nanosized domains, which align more easily along the applied magnetic field direction, resulting in a low coercive field (Hc) of approximately 5.6 mT (56 Oe) and high reversibility.44 Therefore, Metglas stands out as a typical soft ferromagnetic material capable of generating substantial magnetostrictive strain at low Hdc.45,46 The coexistence of ferroelectricity and soft magnetic behavior in the BCZT–PVDF fibers/Metglas composite is substantiated by the observed P–E and M–H plots, thus confirming that the composite structure possesses ME multiferroic properties.
3.2 Characteristics of the PVDF–BCZT fiber composites
Fig. 2a provides a schematic representation of the electrospinning setup designed based on a fundamental concept elucidated in prior reports.47,48 Through the systematic management of solution concentration, operational voltage, flow rate, and the needle-to-collector distance, as well as continuous refinement of the electrospinning configuration, we attained superior control of the process, producing high-quality electrospun BCZT–PVDF fiber composites. Fig. 2b-i–d-i provide a visual representation of the morphological features exhibited by the as-spun BCZT–PVDF fiber composites BCZT5, BCZT10, and BCZT15, which denote 5 wt%, 10 wt%, and 15 wt% BCZT incorporated into the PVDF matrix, respectively. The analysis indicates that the fibers display a smooth surface and a randomly oriented fiber morphology devoid of voids, beads, or cracks.49 This observation suggests that the inclusion of BCZT nanoparticles did not make the fibers brittle. Some visible clusters of BCZT particles were found on the surface of the electrospun fibers. The diameter distribution of the electrospun fiber composites was measured using Image J software, and the average fiber diameters of the BCZT–PVDF fiber composites are presented in Fig. 2e. The data clearly shows that as the filler content in the nanofiber structure increased, there was a corresponding increase in the fiber diameter, ranging from 0.58 µm to 1.08 µm. The addition of BCZT particles results in increased solution viscosity, which leads to increased fiber diameters.50
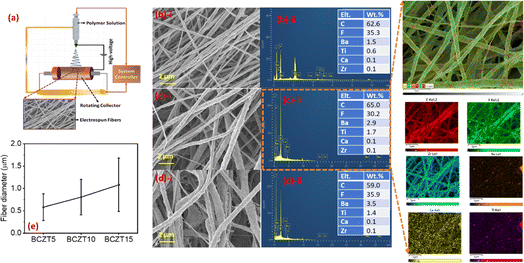 |
| Fig. 2 (a) The schematic diagram of the electrospinning process; the electrospinning apparatus typically comprises a high-voltage power source, injection pump, spinning head, and collection plate. (b-i–d-i) SEM micrographs and (b-ii–d-ii) EDS spectra of the electrospun BCZT5, BCZT10, BCZT15 fibers. The enlarged section of (c-ii) shows the energy-dispersive spectral images of carbon, fluorine zirconium, barium, calcium, and titanium elements, respectively. (e) Average fiber diameters of the electrospun BCZT5, BCZT10, and BCZT15 fibers. | |
Fig. 2b-ii–d-ii display the EDS spectra of the BCZT–PVDF fiber composites. The EDS analysis revealed the presence of the characteristic elements of the polymer matrix (PVDF) and ceramic filler (BCZT). The respective stoichiometric ratios are tabulated as the weight percentages of BCZT nanoparticles incorporated into the PVDF matrix alongside the EDS data (Fig. 2). For further understanding of the elemental distribution, composition-dependent element mapping images of the BCZT10 fiber composite are presented in the enlarged images of Fig. 2c-ii. The homogeneous spatial arrangement of the polymer-filler locations serves as a clear indication of their thorough and comprehensive dispersion.
Fig. 3a shows the XRD patterns of the electrospun fiber composite mats comprising the PVDF polymer matrix and BCZT fillers along with neat PVDF. In the pattern of the neat PVDF electrospun fibers, both α- and β-peaks of PVDF were discernible at diffraction angles of 18.6° and 20.6°, respectively. The composites exhibited two sets of well-defined peaks corresponding to the ferroelectric perovskite BCZT and PVDF polymer, with no discernible impurity peaks. In the case of fiber composites, diminished diffraction peak intensity in the non-polar α-phase and considerable intensity in the polar β-phase were observed. This suggests a transition from the non-polar to the polar phase, promoting the piezoelectric properties of the sample. Moreover, after the integration of the BCZT fillers into the PVDF matrix, there was a noticeable decrease in the intensity of the PVDF peaks. Additionally, the most prominent diffraction peak for the BCZT nanoparticles was detected at 2θ = 31.6°. However, this peak shifted towards a higher diffraction angle with a relatively increased intensity as the concentration of the embedded BCZT filler was increased up to 15 wt%. This can be attributed to strain within the BCZT filler and PVDF polymer matrix.32,34
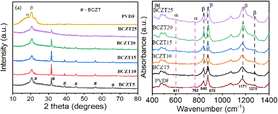 |
| Fig. 3 (a) XRD patterns of neat PVDF and the BCZT10, BCZT15, BCZT20, BCZT25 fiber composites; (b) FTIR analysis of the electrospun neat PVDF and the BCZT5, BCZT10, BCZT15, BCZT20, BCZT25 fiber composites. | |
These results indicate the successful integration of BCZT particles into the PVDF polymer matrix, with the polymer molecular chains likely covering the surface of the particles within the sample.32,33 For further analysis, we investigated the FTIR spectra of the fiber composites to discern and quantify the phase constituents within the PVDF polymer, as depicted in Fig. 3b. The absorbance data in the entire spectral range of 4000–400 cm−1 were meticulously recorded for the as-spun BCZT5, BCZT10, and BCZT15 fiber composites. The spectral bands observed in the range of 400 to 700 cm−1 could primarily be attributed to the presence of metal oxides, specifically associated with the M–O interactions involving elements, such as Ba, Ca, Ti, and Zr. In contrast, the bands located at higher wavenumbers were intricately associated with the characteristic peaks inherent to the PVDF polymer. The presence of both α and β-phases was verified by this analysis. The peaks at 611 and 762 cm−1 signify the non-polar α-phase and are attributed to the rocking vibrations in the PVDF chain.49 Notably, the distinctive band at 840 cm−1 is indicative of the distinct –CH2 rocking vibrations, denoting the presence of the β-phase. The band at 870 cm−1 specifically corresponds to the CH2 rocking and CF2 stretching vibrations within PVDF, respectively.50 Additionally, the peaks at 1171 and 1270 cm−1 are associated with the characteristic vibrations of the polar β-phase.50,51 Interestingly, it was observed that the crystalline transition of PVDF from the α-phase to the β-phase significantly enhanced with the concurrent weakening of the α-phase during the progressive addition of BCZT fillers.
The relative qualitative fraction of the polar β phase was evaluated by employing the Lambert–Beer law followed by infrared absorption:52
| 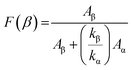 | (1) |
where
Aα and
Aβ represent the intensities of the absorption bands at 762 and 840 cm
−1, respectively.
Kα = 6.1 × 10
4 cm
2 mol
−1 and
Kβ = 7.7 × 10
4 cm
2 mol
−1 are absorption coefficients of the α and β phases, respectively.
52 The beta fraction values of the different fiber composites were as follows: 76.6% for PVDF, 86.4% for BCZT5, 88.8% for BCZT10, and 81.1% for BCZT15, 75.5% for BCZT20 and 69.7% for BCZT25. The incorporation of BCZT particles into PVDF led to an increased
F(
β) value for the optimum BCZT10 sample. This enhancement can be attributed to the establishment of interfacial interactions at the interface between the nanoparticle surfaces and the dipoles within the PVDF structure (
i.e., CF
2 and CF
2), thereby inducing the electroactive β phase.
48 It may also be believed that the larger coulombic forces associated with the local electric field during the electrospinning process are also responsible for the enhancement in the polar β-phase, which is highly desirable from a technological point of view as it provides better piezoelectric properties.
53 Incorporation of excessive BCZT led to increased particle aggregation and disrupted the extended polymer matrix of PVDF, consequently diminishing the β-phase content in the BCZT15, BCZT20 and BCZT25 samples, which corroborates with the XRD results.
46
3.3 Preparation of the flexible MME generator
For seamless MME measurements, the as-spun BCZT–PVDF fiber composite films (45 mm × 28 mm × 0.20 mm) were attached to a magnetostrictive Metglas sheet (45 mm × 28 mm × 0.035 mm), and ohmic contact was made using aluminium (Al) foil. A protective layer was applied to both sides of the BCZT–PVDF fiber/Metglas composite films with commercial laminate sheets made of polyamide (PI) using a hot-pressing system at a temperature of 100 °C. A photograph of the resulting laminated flexible ME fiber composite film is shown in Fig. 4a. The MME generator was fabricated using the ME composite (PVDF–BCZT fibers/Metglas film) with a cantilever structure. One end of the ME cantilever structure was securely clamped, while at the opposite end, a magnetic tip mass comprising NdFeB magnets was attached. The enlarged schematic is depicted in Fig. 4b.
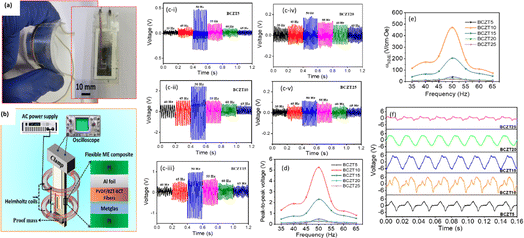 |
| Fig. 4 (a) Photograph of the fabricated ME fiber composite film. (b) An overview of the customized MME measurement setup, with an enlarged schematic representation of the ME cantilever structure. (c-(i–v)) Open-circuit voltage signals generated by different magnetoelectric (MME) generators when subjected to a magnetic noise field of 1 Oe under varying frequencies. (d) Peak-to-peak voltages of the different MME composites at different resonance frequencies. (e) Variation in the magnetoelectric voltage coefficient (αMME) of the fabricated MME generators as a function of Hac. (f) Voltage signals obtained from the designed flexible MME generators under open-circuit conditions exposed to a 10 Oe AC magnetic noise field and at a 50 Hz frequency. | |
To efficiently utilize the MME generator as a power source for low-power electronic devices, it must be capable of extracting the highest available electric power from the surrounding environment. The fabricated MME generator was strategically positioned at the center of a Helmholtz coil, without any extrinsic magnetic DC bias field (Hdc). To replicate the authentic conditions of a power transmission environment, it is essential to set the resonance frequency of the MME device at 50 Hz. We adjusted the position and weight of the magnetic tip mass to modify the resonance frequency of the device.6 To achieve a flexural bending resonance frequency of 50 Hz for the MME device, we attached a magnetic tip mass weighing approximately 8 g and occupying a volume of 615 mm3. In this configuration, the MME generator harnessed energy through a combination of flexural transverse magnetic torque and in-plane longitudinal magnetostrictive oscillations.6
3.4 Characterization of the flexible MME generator
The characterization of the MME device was carried out using a custom-designed measurement setup, as schematically shown in Fig. 4b. This setup involved creating a uniform AC magnetic field through the Helmholtz coils using an AC power supply for various ranges of frequencies, and the output voltage was measured using a digital storage oscilloscope. Fig. 4c(i–v) present the electric output open-circuit voltage signals of the MME generators containing different materials with varying frequencies under a magnetic noise field of 1 Oe.
The MME generators with BCZT5, BCZT10, BCZT15, BCZT20 and BCZT25 exhibited peak-to-peak voltage (Vpp) values of 0.90 V, 5.34 V, 2.2 V, 0.32 V and 0.24 V, respectively, at the resonance frequency of 50 Hz (refer to Fig. 4d).
The ME voltage coupling coefficient (αMME) of each MME generator was calculated using eqn (2):
|  | (2) |
where
V is the output voltage produced upon changing the applied AC magnetic field (
Hac), and
t is the sample thickness. The
αMME values of the MME generators with BCZT5, BCZT10, and BCZT15 operating at their resonance condition, as depicted in
Fig. 4e, were determined to be 41.4 V cm
−1Oe
−1, 472.1 V cm
−1Oe
−1, and 205.4 V cm
−1 Oe
−1, 27.0 V cm
−1 Oe
−1 and 20.1 V cm
−1 Oe
−1, respectively. The output voltage obtained from the optimized BCZT10-based MME generator was remarkable, even without the magnetic DC bias field. It surpasses the values of particulate composites and traditional 2–2 type composites reported previously.
55,56 Notably, the improved harvesting performance of the optimized BCZT10 sample can be attributed to higher interfacial polarization at the filler and polymer interfaces, which is enhanced by the higher magnetic flux concentration of Metglas, ultimately resulting in enhanced piezoelectric effectiveness and high magnetoelastic coupling.
54,61
Power transmission infrastructures are widespread in our surroundings and produce stray magnetic field noise (<10 Oe at 50 Hz). Subsequently, we conducted practical tests with our designed MME generators by subjecting them to an applied AC magnetic noise field of 10 Oe at a 50 Hz frequency. Fig. 4f shows the voltage signals obtained from various MME generators characterized by a waveform period of 20 ms (equivalent to a 50 Hz frequency) under open-circuit conditions: 9.5 V for BCZT5, 14.8 V for BCZT10, 13.3 V for BCZT15, 10.1 V for BCZT20 and 4.2 V for BCZT25 samples. The open-circuit voltage is again favorable for the BCZT10 sample, and the value decreased with an increase in BCZT filler concentration.
The output voltage obtained in this work is significantly greater than those of previously reported piezoelectric nanogenerators designed based on solution-cast and fiber composite films. This value is approximately 360% higher than that of the previously reported flexible polyvinyl-pyrrolidone (PVP)–0.4(BCZT)–0.6 Ni0.5Zn0.5Fe2O4-based MME generator (4.1 V) under similar experimental conditions, as listed in Table 1. Nevertheless, the harvested voltage is relatively lower than those of MME generators designed using single-crystal micro-fiber piezoelectric composites.6
Table 1 Comparison of the energy-harvesting performance of our MME generator with previously reported similar types of piezoelectric energy harvesters and MME generators
Harvesting technique |
Material |
Composite form |
Output voltage (V) |
Power density (µW cm−3) |
Ref. |
Piezoelectric energy harvester |
PVDF/NiFe2O4 |
Casted films |
4.8 |
— |
58
|
DA modified BCZT/PVDF-HFP |
10 |
2.9 |
59
|
PVDF/ZnFe2O4 |
Electrospun fibers |
7 |
4.0 |
60
|
PVDF-HFP/NiFe2O4 |
4 |
— |
61
|
BCZT–PVDF-HFP |
3.2 |
0.51 |
62
|
PVDF–KNN |
25 |
0.30 |
63
|
MME generator |
BCT–BZT/Ni |
Single-crystal macrofibres composites |
44 |
504 |
6
|
PMNZT single crystal/PVDF |
33.4 |
— |
64
|
Pb(Mg1/3Nb2/3)O3–Pb(Zr,Ti)O3 |
— |
42 |
65
|
PMN–PZT SCF/Ni |
45.60 |
265 |
66
|
PMN–PZT SCF/Ni |
96 |
51 000 |
67
|
Polyvinyl-pyrrolidone (PVP)-0.4(BCZT)-0.6 Ni0.5Zn0.5Fe2O4 |
Casted films |
4.1 |
3.89 |
30
|
CuO NP/nylon |
Flash-enhancement |
870 |
720 000 |
68
|
(PVDF–AlN)–(NiO–CNF) |
Electrospun fibers |
9.7 |
6.46 |
69
|
BCZT–PVDF/Metglas |
14.8 |
4.7 |
This work |
3.5 Performance of the flexible MME generators
The harvested individual AC signals had to be transformed into DC signals that can be collected and stored in capacitors or batteries to provide a steady voltage supply to external electronics through a power management circuit. This transformation was achieved through a custom-designed circuit comprising an MME generator, a full-bridge rectifier, a capacitor (selected to optimize the output voltage for a given load resistor), and an adjustable load resistor, as shown in Fig. 5a.
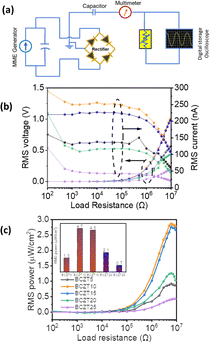 |
| Fig. 5 (a) A schematic representation of the experimental setup used to assess the performance of the flexible MME generators in generating power under the influence of a 50 Hz AC magnetic field. (b) DC voltages and current values after rectification. (c) The calculated electric power generated by the designed flexible MME generators at different load resistances. The inset shows the power density values for the fabricated flexible MME generators. | |
Fig. 5b illustrates the recorded root mean square (RMS) voltages and current values across a range of different external load (RL) conditions ranging from 100 Ω to 8 MΩ. The resultant RMS DC voltages and DC currents of the MME generators were graphed as a function of the load resistance (RL). In contrast, the generated voltage showed a saturation phenomenon at elevated load resistances (approximately 8 MΩ), ultimately converging at the open-circuit DC values of 1.5 V, 2.2 V, 1.8 V, 0.64 V and 0.39 V for BCZT5, BCZT10, BCZT15, BCZT20, and BCZT25 respectively. The corresponding output power (PRMS) obtained from the MME generators was estimated, leveraging the measured RMS current and voltage values in conjunction with the designated external electrical load resistance, as shown in Fig. 5.
The MME generators with BCZT5, BCZT10, BCZT15, BCZT20 and BCZT25 composite films manifested the PRMS values of 0.9, 2.9, 2.8, 1.26 and 0.41 µW (corresponding to power density values of 1.5, 4.7, 4.5, 2.1 and 0.7 µW cm−3), respectively the at optimized load resistance (∼6 MΩ); a representation of this depicted in the inset of Fig. 5c. Although this output power density is in line with recently reported MME generators, it falls short of the output generated by piezoelectric single crystal-based MME generators and the hybrid counterparts.6,57 In view of output performance and inherent versatility, the MME generators featuring the BCZT5, BCZT10 and BCZT15 ME composites underscore a promising capacity to function as an autonomous on-board power supply for sensor circuits rooted in the realm of Internet of Things (IoT) applications.
To retain the energy generated by the MME device, we employed capacitors with capacitance values of up to approximately 50 µF. We then documented the charging process until the charging curve achieved a stable voltage (see Fig. 6a). Additionally, the quantification of electric energy stored within a capacitor was elucidated by using eqn (3) denoted below:
|  | (3) |
where
C signifies the capacitance of the capacitor, while
V pertains to the cumulative electric potential resulting from charging. The energy influx fed per unit time from the MME device into different capacitors (1 µF, 4.7 µF, 10 µF, and 47 µF) was assessed by utilizing the capacitance parameters and the time required for charging to achieve the voltage levels of 2.3 V, 2.32 V, 2.04 V, and 1.60 V, respectively. With a charging time of less than 2 minutes, the optimized MME generator could store approximately 15.5 µW power, which is sufficient to power small IoT devices like micro-robots and digital wristwatches that typically consume power in the range of 10 nW to a few microwatts.
8
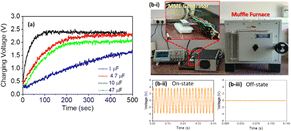 |
| Fig. 6 (a) The charging curves of the different capacitors (1 µF, 4.7 µF, 10 µF and 47 µF) obtained from the MME charging system. (b) (i) The energy-harvesting ability of the optimized MME generator from real-time electronics. (ii) On-state condition. (iii) Off-state condition. | |
3.6 Real-time energy-harvesting performance
Finally, we conducted an in-depth analysis of the energy-harvesting capability of the flexible MME generator from real-time power electronics in the context of a muffle furnace equipment, as illustrated in Fig. 6b-i. The flexible BCZT10 MME device, which was meticulously optimized for superior performance, was strategically positioned beneath the power cord of the muffle furnace operated at 230 V, 50 Hz, and 5 kW. To capture the maximum magnetic noise field emanating from the power cord, the MME generator was placed in proximity (approximately 1 mm away) of the power cord. This configuration enabled us to effectively detect and quantify the magnetic interference caused by the power cord.
Notably, the optimized MME generator demonstrated good capability by generating an output voltage of approximately 7.6 V (Fig. 6b-ii) even in the absence of any external load resistance or battery power (ESI movie S1†). Notably, when the muffle furnace was powered off or under a breakdown condition, we observed the complete absence of a sinusoidal output response from the MME device (Fig. 6b-iii). This observation underscores the critical role of the magnetic interference field in driving voltage generation in this setup.
4. Conclusions
In conclusion, flexible piezoelectric and magnetostrictive Metglas layers were created by laminating BCZT–PVDF microfibers through a cost-effective electrospinning and hot-pressing process. To achieve both high flexibility and magnetoelectric output performance, we fabricated a film-type piezoelectric layer by adjusting the BCZT concentration in the PVDF fibers. We designed flexible MME generators using BCZT–PVDF microfibers and Metglas, which could efficiently harvest magnetic energy without the need for an external DC magnetic bias field, resulting in optimal performance. The maximum performance was achieved at a BCZT concentration of 10 wt% due to the optimal increase in the polar phase of PVDF caused by the BCZT nanoparticles. This structure maintained film flexibility while demonstrating a significantly elevated MME voltage coefficient of approximately 472.1 V cm−1Oe−1. Under an AC magnetic field of 10 Oe at 50 Hz, it generated an RMS open-circuit voltage of 14.8 V (equivalent to a power density of 4.7 µW cm−3). These experimental results demonstrate that the fabricated flexible device not only outperforms particulate composites in magnetic energy conversion but also offers superior flexibility to traditional 2–2 composites. Moreover, our optimized MME device successfully demonstrates practical feasibility in the health monitoring of a muffle furnace by harnessing the stray magnetic field noise emanating from its electronic cables. Therefore, this study introduces a new framework for the development of flexible, lead-free MME generators that hold significant potential for application in wearable self-powered electronic devices and magnetic sensors.
Author contributions
J. Kaarthik: methodology, software, writing – original draft; Nayak Ram: data analysis, writing – review & editing; Radhamanohar Aepuru: writing – review & editing; Salla Gangi Reddy: writing – review & editing. Durga Prasad Pabba – conceptualization, writing – review & editing. Annapureddy Venkateswarlu – supervision, conceptualization, writing – review & editing.
Conflicts of interest
There are no conflicts to declare.
Acknowledgements
The author Annapureddy Venkateswarlu would like to acknowledge the support from the Department of Science and Technology (DST), Government of India, under the INSPIRE Faculty scheme (Grant No. DST/INSPIRE/04/2016/001295) and the Science and Engineering Research Board (SERB), Government of India, under the Start-up Research Grant (Grant No. SERB/F/7249/2019–2020). The authors Radhamanohar Aepuru & Durga Prasad Pabba acknowledge the FONDECYT ANID, Chile for the funding of post-doctoral fellowship FONDECYT 2022 # 3220360. The author Salla Gangi Reddy would like to acknowledge the support from Science and Engineering Research Board (SERB), Government of India, under the Start-up research grant (Grant No. SERB/SRG/2019/000857).
Notes and references
- P. Choudhary, L. Bhargava, V. Singh, M. Choudhary and A. kumar Suhag, Mater. Today: Proc., 2020, 30, 52–56 Search PubMed
.
- Vandana, R. Goel, Shashikant, A. K. Singh and S. Kumar, Mater. Today Commun., 2023, 37, 106985 CrossRef CAS
.
- S. Zeadally, F. K. Shaikh, A. Talpur and Q. Z. Sheng, Renewable Sustainable Energy Rev., 2020, 128, 109901 CrossRef
.
- S. Bairagi, Shahid-ul-Islam, M. Shahadat, D. M. Mulvihill and W. Ali, Nano Energy, 2023, 111, 108414 CrossRef CAS
.
- M. Wlazło, M. Haras, G. Kołodziej, O. Szawcow, J. Ostapko, W. Andrysiewicz, D. S. Kharytonau and T. Skotnicki, Materials, 2022, 15, 6767 CrossRef PubMed
.
- S. H. Park, A. Kumar, J. Kaarthik, V. Annapureddy and J. Ryu, Electron. Mater. Lett., 2020, 16, 369–375 CrossRef CAS
.
- V. Annapureddy, S.-M. Na, G.-T. Hwang, M. G. Kang, R. Sriramdas, H. Palneedi, W.-H. Yoon, B.-D. Hahn, J.-W. Kim, C.-W. Ahn, D.-S. Park, J.-J. Choi, D.-Y. Jeong, A. B. Flatau, M. Peddigari, S. Priya, K.-H. Kim and J. Ryu, Energy Environ. Sci., 2018, 11, 818–829 RSC
.
- V. Annapureddy, H. Palneedi, G.-T. Hwang, M. Peddigari, D.-Y. Jeong, W.-H. Yoon, K.-H. Kim and J. Ryu, Sustainable Energy Fuels, 2017, 1, 2039–2052 RSC
.
- P. Bansal, R. Goel, A. K. Singh, G. Sharma and S. Kumar, J. Magn. Magn. Mater., 2023, 568, 170427 CrossRef CAS
.
- M. A. Tamerd, A. Marjaoui, M. Zanouni, M. El Marssi, M. Jouiad and A. Lahmar, Phys. B, 2023, 667, 415192 CrossRef CAS
.
- M. Kumar, S. Shankar, A. Kumar, A. Anshul, M. Jayasimhadri and O. P. Thakur, J. Mater. Sci.: Mater. Electron., 2020, 31, 19487–19510 CrossRef
.
- D. K. Pradhan, S. Kumari, V. S. Puli, D. K. Pradhan, A. Kumar, S. V Kalinin, R. K. Vasudevan, R. S. Katiyar and P. D. Rack, J. Mater. Chem. C, 2020, 8, 12113–12122 RSC
.
- M. Zheng, T. Usami and T. Taniyama, NPG Asia Mater., 2021, 13, 7 CrossRef CAS
.
- J. S. Feng, K. Xu, L. Bellaiche and H. J. Xiang, New J. Phys., 2018, 20, 53025 CrossRef
.
- T. Jia, Z. Cheng, H. Zhao and H. Kimura, Appl. Phys. Rev., 2018, 5, 21102 Search PubMed
.
- S. Pattipaka, J. Jeong, H. Choi, J. Ryu and G.-T. Hwang, Sensors, 2022, 22, 5723 CrossRef CAS PubMed
.
- D. K. Pradhan, S. Kumari and P. D. Rack, Nanomaterials, 2020, 10, 1–22 CrossRef PubMed
.
- D. R. Patil, S. Lee, S. H. Park, J. Ryu and D.-Y. Jeong, Sens. Actuators, A, 2022, 338, 113451 CrossRef CAS
.
- V. Annapureddy, H. Y. Lee, W. H. Yoon, H. J. Woo, J. H. Lee, H. Palneedi, H. J. Kim, J. J. Choi, D. Y. Jeong, S. N. Yi and J. Ryu, Appl. Phys. Lett., 2016, 109, 093901 CrossRef
.
- D. P. Pabba, B. V. B. Rao, A. Thiam, M. P. Kumar, R. V Mangalaraja, R. Udayabhaskar, R. Aepuru and A. Thirumurugan, Ceram. Int., 2023, 49, 31096–31105 CrossRef CAS
.
- N. D. Kulkarni and P. Kumari, Mater. Res. Bull., 2023, 157, 112039 CrossRef CAS
.
- D. M. Nivedhitha, S. Jeyanthi, P. Venkatraman, A. S. Viswapriyan, S. Guru Nishaanth and S. Manoranjith, Mater. Today: Proc. DOI:10.1016/j.matpr.2023.08.226
.
- P. D. Prasad and J. Hemalatha, J. Magn. Magn. Mater., 2021, 532, 167986 CrossRef CAS
.
- P. D. Prasad and J. Hemalatha, Mater. Res. Express, 2019, 6, 94007 CrossRef CAS
.
- A. Mayeen, M. S. Kala, S. Sunija, D. Rouxel, R. N. Bhowmik, S. Thomas and N. Kalarikkal, J. Alloys Compd., 2020, 837, 155492 CrossRef CAS
.
- M. Zahid, S. Touili, M. Amjoud, D. Mezzane, M. Gouné, H. Uršič, M. Šadl, Y. Elamraoui, K. Hoummada, Z. Kutnjak and M. El Marssi, RSC Adv., 2023, 13, 26041–26049 RSC
.
- H. Staaf, A. Sawatdee, C. Rusu, D. Nilsson, P. Schäffner and C. Johansson, Sci. Rep., 2022, 12, 5233 CrossRef CAS PubMed
.
- V. Annapureddy, S. H. Park, H. Song and J. Ryu, J. Alloys Compd., 2023, 957, 170121 CrossRef CAS
.
- J. Paul Praveen, M. V. Reddy, J. Kolte, S. Dinesh Kumar, V. Subramanian and D. Das, Int. J. Appl. Ceram. Technol., 2017, 14, 200–210 CrossRef CAS
.
- J. Kaarthik, Nitanshi, D. P. Pabba, C. Kaushiga, N. Ram, R. Aepuru, S. G. Reddy and A. Venkateswarlu, Ceram. Int., 2024, 50, 1398–1410 CrossRef CAS
.
- S. Dash, D. K. Pradhan, S. Kumari, Ravikant, M. M. Rahaman, C. Cazorla, K. Brajesh, A. Kumar, R. Thomas, P. D. Rack and D. K. Pradhan, Phys. Rev. B, 2021, 104, 224105 CrossRef CAS
.
- G. A. Kaur, S. Kumar and M. Shandilya, J. Mater. Sci.: Mater. Electron., 2020, 31, 20303–20314 CrossRef
.
- T. Garg, V. Annapureddy, K. C. Sekhar, D.-Y. Jeong, N. Dabra and J. S. Hundal, Polym. Compos., 2020, 41, 5305–5316 CrossRef CAS
.
- Q. Chi, G. Liu, C. Zhang, Y. Cui, X. Wang and Q. Lei, Results Phys, 2018, 8, 391–396 CrossRef
.
- S. Belkhadir, A. Neqali, M. Amjoud, D. Mezzane, A. Alimoussa, E. Choukri, Y. Gagou, I. Raevski, M. El Marssi, I. A. Luk’yanchuk, B. Rožič and Z. Kutnjak, J. Mater. Sci.: Mater. Electron., 2019, 30, 14099–14111 CrossRef CAS
.
- X. Ji, C. Wang, S. Li, S. Zhang, R. Tu, Q. Shen, J. Shi and L. Zhang, J. Mater. Sci.: Mater. Electron., 2018, 29, 7592–7599 CrossRef CAS
.
- J. Kaarthik, C. Kaushiga, G. Sradha, N. Ram, S. G. Reddy, K. C. Sekhar and A. Venkateswarlu, J. Alloys Compd., 2023, 943, 169069 CrossRef CAS
.
- S. T. Dang, L. L. Xue, L. F. He, Y. C. Shi, H. N. Li, Y. C. Hu, J. Shang, S. Q. Yin and X. W. Wang, J. Mater. Sci.: Mater. Electron., 2022, 33, 26100–26112 CrossRef CAS
.
- N. Buatip, N. Promsawat, N. Pisitpipathsin, O. Namsar, P. Pawasri, K. Ounsung, K. Phabsimma, S. T. Rattanachan, P. Janphuang and S. Projprapai, Integr. Ferroelectr., 2018, 187, 45–52 CrossRef CAS
.
- S. Kumari, A. Kumar, V. Kumar, S. K. Dubey, P. K. Goyal, S. Kumar, A. L. Sharma and A. Arya, J. Mater. Sci.: Mater. Electron., 2021, 32, 16900–16915 CrossRef CAS
.
- X. Ji, C. Wang, T. Harumoto, S. Zhang, R. Tu, Q. Shen and J. Shi, Sci. Rep., 2020, 10, 20352 CrossRef CAS PubMed
.
- X. Lu, B. Fang, S. Zhang, N. Yuan, J. Ding, X. Zhao, F. Wang, Y. Tang, W. Shi, H. Xu and H. Luo, Funct. Mater. Lett., 2017, 10, 1750046 CrossRef CAS
.
- L. Chen, Y. Wang, T. Luo, Y. Zou and Z. Wan, Materials, 2021, 14, 6397 CrossRef CAS PubMed
.
- T. Zhang, X. Yang, J. Ouyang, S. Chen, B. Tong, Y. Zhu and Y. Zhang, Appl. Compos. Mater., 2014, 21, 579–590 CrossRef
.
- S. Yang, J. Xu, X. Zhang, S. Fan, C. Zhang, Y. Huang, Q. Li, X. Wang, D. Cao, J. Xu and S. Li, J. Phys. D Appl. Phys., 2022, 55, 175002 CrossRef
.
- Y. Liu, B. Ducharne, G. Sebald, K. Makihara and M. Lallart, Appl. Sci., 2023, 13, 3477 CrossRef CAS
.
- K. S. Chary, V. Kumar, C. D. Prasad and H. S. Panda, J. Australas. Ceram. Soc., 2020, 56, 1107–1117 CrossRef CAS
.
- S. Liu, S. Xue, W. Zhang, J. Zhai and G. Chen, J. Mater. Chem. A, 2014, 2, 18040–18046 RSC
.
- S. Ramasundaram, A. Rahaman and B. Kim, Polymer (Guildf)., 2019, 183, 121910 CrossRef CAS
.
- X. Hu, X. Yan, L. Gong, F. Wang, Y. Xu, L. Feng, D. Zhang and Y. Jiang, ACS Appl. Mater. Interfaces, 2019, 11, 7379–7386 CrossRef CAS PubMed
.
- B. Bagchi, N. A. Hoque, N. Janowicz, S. Das and M. K. Tiwari, Nano Energy, 2020, 78, 105339 CrossRef CAS PubMed
.
- A. Sasmal, S. Maity, P. Maiti, A. Arockiarajan and S. Sen, Chem. Eng. J., 2023, 468, 143794 CrossRef CAS
.
- S. Sharafkhani and M. Kokabi, Composites, Part B, 2021, 222, 109060 CrossRef CAS
.
- S. Liu, S. Liao, K. Wei, L. Deng, L. Zhao and H. Zou, Battery Energy, 2023, 20230005 CrossRef CAS
.
- Y. Long, J. Qiu, X. He, Q. Chang, Z. Hu and H. Liu, AIP Adv., 2017, 7, 125029 CrossRef
.
- S. Yang, J. Xu, X. Zhang, S. Fan, C. Zhang, Y. Huang, Q. Li, X. Wang, D. Cao, J. Xu and S. Li, J. Phys. D Appl. Phys., 2022, 55, 175002 CrossRef
.
- V. Annapureddy, M. Kim, H. Palneedi, H.-Y. Lee, S.-Y. Choi, W.-H. Yoon, D.-S. Park, J.-J. Choi, B.-D. Hahn, C.-W. Ahn, J.-W. Kim, D.-Y. Jeong and J. Ryu, Adv. Energy Mater., 2016, 6, 1601244 CrossRef
.
- P. D. Prasad and J. Hemalatha, J. Magn. Magn. Mater., 2021, 532, 167986 CrossRef CAS
.
- K. S. Chary, V. Kumar, C. D. Prasad and H. S. Panda, J. Australas. Ceram. Soc., 2020, 56, 1107–1117 CrossRef CAS
.
- P. D. Prasad and J. Hemalatha, J. Alloys Compd., 2021, 854, 157189 CrossRef CAS
.
- D. Ponnamma, O. Aljarod, H. Parangusan and M. Al Ali Al-Maadeed, Mater. Chem. Phys., 2020, 239, 122257 CrossRef CAS
.
- G. A. Kaur, S. Kumar and M. Shandilya, J. Mater. Sci.: Mater. Electron., 2020, 31, 20303–20314 CrossRef
.
- B. S. Athira, K. P. Surendran and A. Chandran, Sustainable Energy Fuels, 2023, 7, 5704–5713 RSC
.
- C. M. Baek, G. Lee and J. Ryu, J. Korean Inst. Electr. Eng., 2023, 36, 620–626 Search PubMed
.
- H.-S. Kim, N. Kumar, J.-J. Choi, W.-H. Yoon, S. N. Yi and J. Jang, Adv. Intell. Syst., 2024, 6, 2300474 CrossRef
.
- D. R. Patil, S. Lee, A. Thakre, A. Kumar, H. Song, D.-Y. Jeong and J. Ryu, J. Mater., 2023, 9, 735–744 Search PubMed
.
- S. H. Kim, A. Thakre, D. R. Patil, S. H. Park, T. A. Listyawan, N. Park, G.-T. Hwang, J. Jang, K.-H. Kim and J. Ryu, ACS Appl. Mater. Interfaces, 2021, 13, 19983–19991 CrossRef CAS PubMed
.
- H. E. Lee, J. H. Park, D. Jang, J. H. Shin, T. H. Im, J. H. Lee, S. K. Hong, H. S. Wang, M. S. Kwak, M. Peddigari, C. K. Jeong, Y. Min, C. H. Park, J.-J. Choi, J. Ryu, W.-H. Yoon, D. Kim, K. J. Lee and G.-T. Hwang, Nano Energy, 2020, 75, 104951 CrossRef CAS
.
- N. Ram, J. Kaarthik, S. Singh, H. Palneedi, P. D. Prasad and A. Venkateswarlu, Ceram. Int., 2024 Search PubMed
.
- X. W. Wang, B. H. Zhang, G. Feng, L. Y. Sun, Y. C. Shi, Y. C. Hu, J. Shang, S. Y. Shang, S. Q. Yin and X. E. Wang, J. Electron. Mater., 2020, 49, 880–887 CrossRef CAS
.
|
This journal is © The Royal Society of Chemistry 2024 |