DOI:
10.1039/D4SC02907K
(Edge Article)
Chem. Sci., 2024,
15, 12369-12379
Understanding the role of supported Rh atoms and clusters during hydroformylation and CO hydrogenation reactions with in situ/operando XAS and DRIFT spectroscopy†
Received
2nd May 2024
, Accepted 29th June 2024
First published on 10th July 2024
Abstract
Supported Rh single-atoms and clusters on CeO2, MgO, and ZrO2 were investigated as catalysts for hydroformylation of ethylene to propionaldehyde and CO hydrogenation to methanol/ethanol with in situ/operando diffuse reflectance infrared Fourier transform spectroscopy (DRIFTS) and X-ray absorption spectroscopy (XAS). Under hydroformylation reaction conditions, operando spectroscopic investigations unravel the presence of both single atoms and clusters and detected at first propanal and then methanol. We find that the formation of methanol is associated with CO hydrogenation over Rh clusters which was further confirmed under CO hydrogenation conditions at elevated pressure. The activity of catalysts synthesized via a precipitation (PP) method over various supports towards the hydroformylation reaction follows the order: Rh/ZrO2 > Rh/CeO2 > Rh/MgO. Comparing Rh/CeO2 catalysts synthesized via different methods, catalysts prepared by flame spray pyrolysis (FSP) showed catalytic activity for the hydroformylation reaction at lower temperatures (413 K), whereas catalysts prepared by wet impregnation (WI) showed the highest stability. These results not only provide fundamental insights into the atomistic level of industrially relevant reactions but also pave the way for a rational design of new catalysts in the future.
Introduction
The hydroformylation (HF) reaction, an industrially demanding reaction for producing aldehydes via the reaction of olefin and synthesis gas, is predominantly catalyzed by Rh molecular catalysts.1,2 On the other hand, higher alcohol synthesis (HAS)3–5 proceeds only in the presence of synthesis gas and is largely catalyzed by Rh solid catalysts.5–7 Both reactions follow rather similar pathways via C–CO coupling reactions and yet the products are different. Recent studies show that Rh single-atom catalysts (SACs)8–10 can be promising alternatives for heterogeneously catalyzed hydroformylation reactions11,12 where the C–CO coupling reaction occurs like in conventional homogeneous Rh-phosphine complexes. Therefore, SACs are considered analogous to molecular catalysts.13,14 The tunable local environment around the supported single atom makes it particularly attractive to investigate how the C–CO coupling reaction takes place. A recent example shows that Rh-WOx pair site catalysts can boost the activity for gas phase hydroformylation reactions.15 However, SACs are also prone to agglomeration due to their high surface energy particularly in a highly reductive environment.16,17 Several reports claimed that Rh SACs stabilized by ionic liquids,18 functionalized with an alkyl chain over a solid support,19 synthesized by tuning surface deficiencies,20 and even with strong metal–support cooperativity21,22 show superior stability. Zhao et al. observed redispersion of Rh0 clusters inside zeolite MFI to Rh single atoms and, ultimately, the formation of Rh+(CO)2 during hydroformylation of ethylene at 50 °C, which shows that the dynamic structural change plays a vital role under operating conditions.23
In the absence of an olefin, CO hydrogenation to higher alcohols (C2+) over Rh catalysts7,24,25 is a promising route since the synthesis gas mixture can be obtained from various processes based on renewable resources such as biomass conversion,26 CO2 valorization,27 dry-reforming of CO2 with CH4,28 electrochemical conversion of CO2,29 photochemical conversion of CO2,30 conversion of plastic waste,31 and many others.32 However, until today, there has been no suitable catalyst that can achieve the desired activity and selectivity which are required for industrial scale production.33 Therefore, it is extremely important to know the effect of Rh nuclearity,24,34 support,35 adsorbate–adsorbate interaction,36 promoters,32,33 morphology,25 and particle size.37 Cu-based catalysts are also promising alternatives for CO hydrogenation to higher alcohols; however, they compete with methanol synthesis and require promoters of alkali, transition, and rare-earth metals to produce higher alcohols as selective products.38–40 The limitation of using other transition metals such as Co is that it catalyzes the competing Fischer–Tropsch process.41
A typical homogeneous HF reaction follows the classical Heck–Breslow mechanism42,43 and usually proceeds via several steps such as (a) ligand dissociation from the complex, (b) olefin coordination, (c) alkyl formation via hydride insertion, (d) CO insertion, (e) H2 oxidative addition and (f) reductive elimination. On the other hand, HAS involves (a) CO dissociation, (b) carbon–carbon bond formation, (c) CO insertion and (d) stepwise hydrogenation to the alcohol.33,44 While in the HF reaction, all the steps can potentially occur over monometallic Rh, in HAS, synergetic effects between two neighbouring sites are likely required for C–C bond formation and CO insertion steps. For monometallic catalysts, the M0 state is assumed to promote the C–C chain growth during HAS whereas Mδ+ is responsible for CO insertion like in the classical HF reaction.33,45
This brings us to the question of whether Rh SACs can be active for both types of reactions as Rh exhibits a positive charge, and in a reductive environment, Rh0 has been observed.5 There have been many reports where the co-existence of SACs and small clusters was detected simultaneously under in situ conditions such as propane dehydrogenation,46 dry-reforming of methane,47,48 or CO2 hydrogenation.49,50 On the other hand, it is well known that Rh forms Rh(CO)2+-carbonyls in the presence of CO due to the strong interaction of CO with Rh.51 In many of these catalytic processes, the presence of two species led to two different reaction pathways and hence two different products were observed. A recent study by Wu et al. claimed that the dynamic evolution of Rh single atoms to clusters over CeO2 led to good activity for CO hydrogenation to ethanol, where the presence of Rh single atoms and clusters was found during the catalytic activity test.34
To understand these two reactions in detail, which can occur in parallel, we have synthesized Rh SACs and cluster catalysts over various supports (CeO2, MgO, and ZrO2) via precipitation,16 atom trapping,52,53 pyrolysis of molecular complexes54 and flame spray pyrolysis (FSP).55 These supports are chosen due to their variable acidity, reducibility, and, most importantly, variation in reactivity during the catalytic HF reaction as reported in the literature.9 The as-synthesized catalysts were characterized ex situ by various state-of-the-art characterization methods and the dynamic behaviour56 of Rh catalysts was tracked by in situ X-ray absorption spectroscopy (XAS)57 and diffuse reflectance Fourier transform infrared spectroscopy (DRIFTS).16 The products of the two reactions, (a) hydroformylation of ethylene to propanal and (b) CO hydrogenation to methanol/ethanol were monitored using an online mass spectrometer (MS).
Results and discussion
Synthesis and characterization of catalysts
The Rh catalysts synthesized via different routes have different metal contents in the range between 0.8 and 1.5 wt% as confirmed by the inductively coupled plasma optical emission spectroscopy (ICP-OES) analysis. A plot of BET surface area as a function of metal content in the catalysts is shown in Fig. S1 of the ESI.† The surface area varies greatly depending on the support and synthesis procedure. For example, Rh/CeO2 catalysts synthesized via FSP showed the highest specific surface area (133 m2 g−1) in comparison to the one synthesized by wet impregnation (56 m2 g−1) or precipitation methods (93 m2 g−1). This is due to the fast quenching of the resulting solid synthesized by the FSP method which minimizes the particle growth, as explained elsewhere.55 Among the catalysts prepared over different supports (CeO2, ZrO2 and MgO), ZrO2 (synthesized by the precipitation method) showed the highest specific surface area of 201 m2 g−1, which makes it attractive for application in catalysis.
The powder X-ray diffraction (XRD) patterns of the as-synthesized catalysts showed highly crystalline structures (Fig. S2–S7 in ESI†) in all cases. There is no Rh reflection (at 2θ = 40°) observed, confirming the high dispersion of Rh over different supports. Even when the content of Rh is increased from 0.6 wt% to 5 wt% over the CeO2 support, we did not observe any reflection associated with Rh metal or RhOx clusters as shown in Fig. S2.† The FSP method was also successful in preventing the formation of Rh nano-particles with long-range order (Fig. S3†), as also reported in the literature for other metals.58–60 Aberration-corrected (Cs) high-angle annular dark-field (HAADF) scanning transmission electron microscopy (STEM) was used to visualize the nuclearity of Rh in the as-synthesized state of the catalysts over different supports. The images and the corresponding Energy Dispersive X-ray (EDX) maps are shown in Fig. 1 and in Fig. S8–S10.† The EDX maps confirm that Rh is uniformly distributed in all the catalysts except the Rh catalyst supported over CeO2 synthesized by the pyrolysis method. In this case, RhP was formed, which showed nano-particle formation. The presence of some clusters (<2 nm) in the FSP synthesized catalyst cannot be entirely ignored, as observed in the EDX maps (Fig. S8†).
 |
| Fig. 1 HAADF-STEM images of Rh catalysts and the corresponding EDX maps. Rh/CeO2, Rh/ZrO2, Rh/MgO are synthesized via the precipitation method, and RhP/CeO2 is synthesized via the pyrolysis route. | |
We further conducted X-ray absorption spectroscopy (XAS) to elucidate the local structure of the Rh catalysts as shown in Fig. 2. The extended X-ray absorption fine structure analysis (EXAFS) showed that Rh species over CeO2, ZrO2, and MgO are highly dispersed with Rh–O as the first coordination shell (without any Rh–Rh scattering) whereas the RhP/CeO2 catalyst exhibits predominantly the Rh–Rh shell as shown in Fig. 2(a). The X-ray absorption near edge structure (XANES) spectra at the Rh K-edge showed that Rh is in an oxidation state of +3 over CeO2, ZrO2 and MgO and in a partially reduced state in the RhP/CeO2 catalyst as shown in Fig. 2(b). The spectra of Rh catalysts synthesized over ceria with various methods are shown in ESI Fig. S11,† and the corresponding free parameters derived from the fit are shown in Table S2.† The X-ray photoelectron spectroscopy (XPS) investigation further supported the results obtained from XAS. All the catalysts except RhP/CeO2 contain RhIII as the predominant species on the surface as shown in Fig. S12 of the ESI.† The components present and their percentages are shown in Table S2.†
 |
| Fig. 2 (a) Fourier transform of the k3-weighted EXAFS spectra and (b) XANES spectra at the Rh K-edge of the Rh catalysts supported over CeO2, ZrO2, and MgO. Rh2O3 and Rh foil are shown as references for comparison. | |
XAS57 is an averaging technique over all species present and has certain limitations when used on heterogeneous samples. For example, over the oxide-supported catalyst, EXAFS cannot distinguish between single site and clusters of a few atoms as explained by Finzel et al.61 Therefore, as a complementary technique, we carried out diffuse reflectance Fourier transform infrared spectroscopy (DRIFTS) investigations with CO as a probe molecule to elucidate the nuclearity of the Rh catalysts synthesized over different supports as shown in Fig. 3. CO adsorbed on Rh shows characteristic symmetric and anti-symmetric CO vibrational frequencies of single site RhI(CO)2 in the range of 2070–2090 cm−1 and 2000–2020 cm−1 respectively.16,49,62,63 Rh/CeO2 synthesized via precipitation (PP), flame spray pyrolysis (FSP) and wet impregnation (WI) exhibit Rh single sites as the predominant species as shown in Fig. 3(a), (d) and (e) respectively. Similarly, Rh/ZrO2 (PP) also showed the presence of symmetrical and asymmetrical CO vibrational frequencies at 2078 and 2007 cm−1 respectively.64 On the other hand, the RhP/CeO2 catalyst synthesized via the pyrolysis method showed the presence of CO adsorption over the Rh clusters in the lower frequency region (1850–1950 cm−1)16 as shown in Fig. 3(c). The spectra are also shown on the logarithmic scale in Fig. S13.† These results are in agreement with the TEM observations (Fig. 1), which indicate the presence of Rh clusters as RhP/CeO2. The DRIFT spectra of Rh/CeO2 (5 wt%) with adsorbed CO showed various features (2126, 2035 cm−1), which can be attributed to the weakly adsorbed CO over Rh3+ or Rh2+ species present in the form of RhOx clusters.23 Upon flowing Ar, two distinct CO absorption frequencies at 2023 and 2092 cm−1 were observed, as shown in Fig. S14,† which we believe arise from the fraction of Rh single atoms present in this high loading catalyst. Moreover, the presence of a broad CO adsorption peak between 1850 and 1900 cm−1 (Fig. S14†) infers the presence of Rh clusters in this catalyst. The Rh/MgO catalyst did not show any CO vibrational frequencies in the range 1800–2100 cm−1, which indicates that Rh supported on MgO is coordinatively saturated as also found previously.9,65 The CO absorption frequencies of Rh catalysts over various supports and their assignments are shown in Fig. 3(g) as well as in Table S4 of the ESI.†
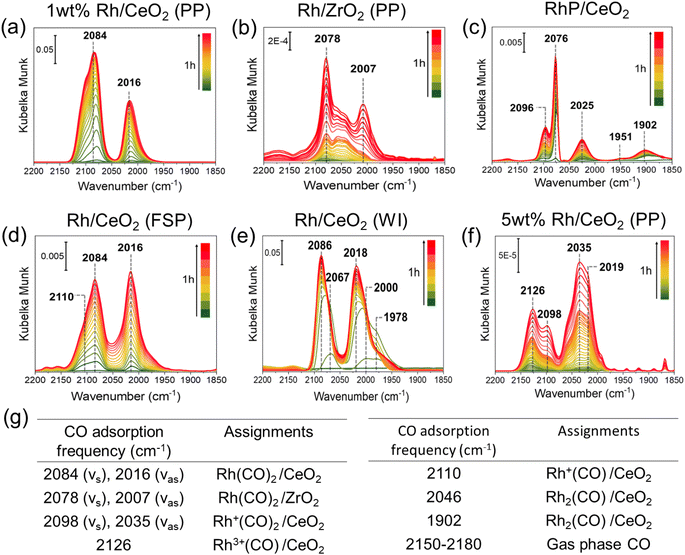 |
| Fig. 3 CO-DRIFTS spectra at room temperature over various Rh catalysts. (a) Rh/CeO2 (PP), (b) Rh/ZrO2 (PP), (c) RhP/CeO2, (d) Rh/CeO2 (FSP), (e) Rh/CeO2 (WI), and (f) 5 wt% Rh/CeO2 (PP). (g) Table of CO absorption frequency with assignments of Rh species. PP: precipitation, FSP: flame spray pyrolysis, and WI: wet impregnation. νs and νas stands for symmetric and anti-symmetric CO vibrational frequencies. The green to red color bar represents the experiment over 1 hour at room temperature. | |
In situ XAS investigations
In order to understand the electronic state of Rh under operating conditions, we further carried out in situ XAS studies at the Rh K-edge for hydroformylation of ethylene (experimental setup is shown in S15 of the ESI†). The catalysts were exposed to a mixture of a 1
:
1
:
1 ratio of ethylene
:
CO
:
H2 while heating from room temperature to 573 K (2 K min−1 and 30 min dwell time) at atmospheric pressure with a total flow of 40 mL min−1. The gas mixture at the outlet was monitored with an online mass spectrometer. The temperature-dependent in situ XANES spectra were collected continuously at the Rh K-edge from room temperature to 573 K in the presence of the reaction mixture as shown in Fig. 4(a)–(d). All Rh catalysts were reduced under HF conditions with a change in the oxidation state of Rh from Rh3+ to Rh0. Linear combination analysis (LCA) was used to derive the oxidized and reduced components during the reaction as shown in Fig. 4 (e)–(h). The trend of the reduction temperature from the highest to the lowest follows the order of Rh/MgO > Rh/ZrO2 > Rh/CeO2 > RhP/CeO2. However, the catalytic activity does not follow the same trend. Both in Rh/CeO2 and Rh/ZrO2, we observed propanal at around a temperature of 500 K, which is the product formed via the HF reaction as shown in Fig. 4(i) and (j). At this stage, almost 80–90% of the Rh species are already reduced which indicates the possible involvement of clusters during catalysis. Interestingly, when all the Rh is reduced, methanol is detected in the MS. This indicates that when Rh forms clusters, the pathway for CO hydrogenation is enhanced as also shown by Wu et al.34 This is supported by our catalytic test over the RhP/CeO2 catalyst which contains a significant fraction of Rh clusters, for which formation of methanol was detected almost parallel to the formation of propanal (Fig. 4(l)). Rh/MgO did not show any activity even in the presence of reduced rhodium, which indicates that the basic support does not promote HF or methanol formation. Among the Rh/CeO2 catalysts, Rh synthesized by FSP showed catalytic activity at a lower temperature (ca. 410 K) compared to the catalysts synthesized by precipitation or wet impregnation methods, as shown in Fig. S16 of the ESI.† This might be due to better accessibility of the Rh atoms deposited on high surface area CeO2 when synthesized via the FSP method.55
 |
| Fig. 4
In situ XANES spectra at the Rh K-edge of catalysts prepared over different supports ((a)–(d)), the fraction of components (oxidized and reduced) derived from linear combination fitting ((e)–(h)) during the hydroformylation reaction of ethylene, and selectivity of different products monitored using an online mass spectrometer (i)–(l). The dotted lines are a guide to the eye. PP stands for precipitation. Reaction conditions: 25 mg catalysts, 1 : 1 : 1 mixture of ethylene : CO : H2, T = RT-573 K (ramp rate 2 K min−1), P = 1 bar, flow rate: 40 mL min−1. | |
In order to find the origin of methanol formation, we further carried out the reaction in the absence of ethylene (CO
:
H2 of 1
:
1) in a quartz capillary micro-reactor at 10 bar pressure in the temperature range of RT-573 K. The temperature dependent XANES spectra and the linear combination analysis of the oxidized and reduced components during the reaction are shown in Fig. 5. The mass spectrometer (MS) detected both methanol and ethanol over Rh/CeO2 synthesized via the precipitation method (Fig. 5(i)). This further indicates that the nuclearity of Rh plays a crucial role in obtaining methanol and ethanol products as previously reported.24,34 The Rh/ZrO2 catalyst showed only methanol in the product stream and no ethanol was observed. Rh/MgO did not show any activity as was also the case for the HF reaction. RhP/CeO2 showed initially only methanol, and ethanol was only observed at higher temperatures (550 K) when a significant portion of Rh0 formed. The Fourier transformed k3-weighted EXAFS spectra after the HF reaction and CO hydrogenation reaction are shown in Fig. S17 of the ESI.† The predominant presence of the Rh–Rh shell indicates that most of the catalysts were reduced completely at the end of the reaction except Rh/MgO and Rh/ZrO2, which showed a significant proportion of the oxidic species (presence of the Rh–O shell). We further carried out fitting of the Rh–Rh shell to calculate the coordination number of Rh in the spent catalysts. The fits and the free parameters are shown in ESI Fig. S18 and in Table S5.† The coordination numbers obtained from the fits are in the range between 1 and 6. Nevertheless, such low coordination values indicate that the size of the reduced Rh species is extremely small.66,67 To further confirm whether the concentration of Rh plays a significant role during the reaction, we have conducted the CO hydrogenation reaction over Rh/CeO2 with three different Rh loadings (1.1, 2.2 and 4.5 wt%). The results are shown in Fig. S19 of the ESI.† The catalytic results indicate that upon increasing Rh content over the CeO2 support, the formation of methanol and ethanol is enhanced. 4.5 wt% Rh/CeO2 showed the formation of methanol and ethanol simultaneously like the RhP/CeO2 catalyst, which indicates that for a highly loaded sample, Rh easily forms nano-particles which can promote the C–CO coupling reaction. These results further signify the role of clusters during HF and CO hydrogenation reactions.
 |
| Fig. 5
In situ XANES spectra at the Rh K-edge of catalysts prepared over different supports ((a)–(d)), the fraction of components (oxidized and reduced) derived from linear combination fitting ((e)–(h)) during CO hydrogenation, and selectivity of different products monitored using an online mass spectrometer (i)–(l). The dotted lines are a guide to the eye. PP stands for precipitation. Reaction conditions: 25 mg catalysts, 1 : 1 mixture of CO : H2, T = RT-573 K (ramp rate 2 K min−1), P = 10 bar, flow rate: 40 mL min−1. | |
Based on our results, three summary points can be hypothesized for the reaction mechanism. (a) Rh single atom catalysts form the active center upon treatment with syngas and actively take part in the hydroformylation reaction, (b) when Rh clusters are formed, it mainly favors methanol synthesis, and (c) for ethanol formation, both atoms and clusters participate during the reaction as the CO insertion step requires Rhδ+ species for C–C chain propagation. These points are summarized in Fig. S20 of the ESI.†
In situ DRIFTS investigations
In order to investigate the change in the state of Rh, we carried out DRIFTS experiments under various conditions, as shown in Fig. 6. As discussed before, at room temperature Rh/CeO2 showed CO vibrational frequencies at 2084 and 2016 cm−1 that correspond to symmetrical and anti-symmetrical vibrational modes of CO of RhI (CO)2 species. The change in the state of Rh is already visible at 453 K under 1% (CO + H2) as a new CO vibrational frequency appears at 2046 cm−1 (Fig. 6(b)), which corresponds to CO adsorbed on Rh clusters. Upon increasing the temperature to 523 K, clusters of Rh formed and an additional feature at 2036 cm−1 was observed (Fig. 6(c)), which we believe to occur due to the formation of Rh(CO)H species as reported in the literature.37 This trend continued when ethylene was introduced to the reaction mixture. However, even under such a reductive atmosphere, there is still the presence of isolated Rh species (2030 cm−1) as shown in Fig. 6(d). These results correlate with the in situ XAS studies and further hint that Rh atoms and clusters formed under reaction conditions play a crucial role during the HF reaction. Additionally, we carried out DRIFTS experiments on the Rh/CeO2 catalyst synthesized via the wet impregnation method, which showed higher stability based on our in situ XAS studies (Fig. S16(c)†). The DRIFTS results showed no obvious formation of clusters, unlike for the catalyst synthesized via the precipitation method, as shown in Fig. S21 of the ESI.† A comparison of the H2-temperature programmed reduction (TPR) profile and the DRIFT spectra collected at 453 K with the catalyst prepared over various supports (CeO2, ZrO2, and MgO) is shown in Fig. S23 of the ESI.† The results showed that based on the support, Rh exhibits different reduction temperatures, and it follows the order of Rh/CeO2 < Rh/ZrO2 < Rh/MgO. The DRIFT spectra recorded at 453 K under CO + H2 indicated that Rh/ZrO2 did not show any Rh cluster formation. On the other hand, Rh/MgO did not show any feature regarding CO adsorption, which infers that Rh is not easily available on the surface of MgO and may be located in the highly coordinated bulk environment.
 |
| Fig. 6
In situ DRIFTS spectra collected for 1 hour under various conditions of the catalyst Rh/CeO2 synthesized via precipitation method. (a) 1% CO at room temperature, (b) 1% (CO + H2) at 453 K, (c) 1% (CO + H2) at 523 K and (d) 1% (ethylene + CO + H2) at 523 K. | |
Conclusions
In conclusion, we have found that highly dispersed Rh catalysts supported over CeO2, ZrO2, and MgO showed co-existence of supported single Rh atoms and clusters under conditions of HF of ethylene and CO hydrogenation reactions. The in situ XAS studies infer the formation of reduced Rh particles/clusters while both propanal and methanol were observed during the HF reaction. In addition, the in situ DRIFTS studies showed vibrational frequencies of CO adsorbed over Rh atoms and clusters under reaction conditions, which further supports that such unprecedented reactivity might have occurred due to the presence of both species. The formation of a significant amount of ethanol was observed during the CO hydrogenation reaction over the RhP/CeO2 catalyst, which also showed the presence of nano-particles (>2 nm) already in the as-synthesized catalyst. Moreover, by increasing the concentration of Rh from 1.1 wt% to 4.5 wt% over the CeO2 support, we observed the CO hydrogenation reaction at a relatively low temperature. This indicates that a certain size of Rh nano-particles is important to promote the C–C coupling reaction during higher alcohol synthesis. Comparisons of catalytic activity over various supports showed that acidic supports, such as ZrO2, promote the hydroformylation reaction, whereas basic supports, such as MgO, result in negligible catalytic activity. The varying reactivity towards hydroformylation of ethylene is dependent on the preparation method and support, as summarized in Fig. 7. Among the catalysts synthesized over CeO2 with various methods, catalysts prepared via flame spray pyrolysis showed activity at low temperatures which indicates that the method of preparation plays a vital role during the reaction. This study will pave the way for further development of catalysts for such an industrially relevant reaction. In the future, we plan to investigate the CO insertion mechanism of the two reactions in more detail by introducing promoters and extending the reactions over further industrially relevant supports.
 |
| Fig. 7 Summary of reactivity towards the hydroformylation reaction of ethylene with supported Rh catalysts on CeO2, ZrO2, and MgO. PP, FSP, and WI stand for precipitation, flame spray pyrolysis, and wetness impregnation, respectively. | |
Data availability
Data supporting the article (experimental protocols, XRD, XPS, BET, and TPR results) are available in the ESI.† The raw data can be provided upon request to the corresponding author.
Author contributions
BBS: conceptualization; investigation, data curation & formal analysis (catalyst preparation & catalytic testing, including in situ XAS and DRIFTS); formal analysis (XAS, DRIFT, and XRD); writing the original draft. DN: data curation (XAS and XPS) and formal analysis (XPS); writing-review and editing. DD: data curation and formal analysis (XAS); writing-review and editing. ALN: data curation and formal analysis (TEM); writing-review and editing. LB: data curation (XAS); writing-review and editing. BK: data curation and formal analysis (XPS); writing-review and editing. JDG: overall supervision, funding acquisition, resources, writing–discussion–review and editing.
Conflicts of interest
The authors declare no conflicts of interest.
Acknowledgements
The authors would like to thank Dr Eduardo Barbosa and Angela Deutsch for nitrogen physisorption measurements and Armin Lautenbach for ICP-OES analysis. Alisa Sinigalia is thanked for her assistance during the XPS measurements. Andrea De Giacinto and Dr Linda Klag are thanked for their assistance with the FSP synthesis. Michael Borchers is thanked for the H2-TPR measurements. Dr Lu Chen (University of Cambridge, UK) and Prof. Philippe Serp (LCC, CNRS, France) are thanked for the fruitful discussion during the preparation of the manuscript. We acknowledge DESY (Hamburg, Germany), a member of the Helmholtz Association HGF, for the provision of beamtime, allocated to the proposals I-20200891 and I-20211473 and KIT light source (A2020-031-018713). We would like to thank Dr Edmund Welter (P65, DESY) and Dr Anna Zimina (CATACT, KIT Light Source and IKFT) for their assistance in setting up the beamline. HAADF-STEM imaging and EDX imaging were carried out with the support of the Karlsruhe Nano Micro Facility (KNMFi, https://www.knmf.kit.edu), a Helmholtz Research Infrastructure at the Karlsruhe Institute of Technology (KIT, https://www.kit.edu). Support from the Helmholtz Association and Deutsche Forschungsgemeinschaft (DFG, German Research Foundation) – SFB 1441 – Project-ID 426888090 is gratefully acknowledged. BBS would like to thank CNRS for startup funding.
Notes and references
- R. Franke, D. Selent and A. Börner, Applied Hydroformylation, Chem. Rev., 2012, 112, 5675–5732 CrossRef CAS PubMed.
- M. Sparta, K. J. Børve and V. R. Jensen, Activity of Rhodium-Catalyzed Hydroformylation: Added Insight and Predictions from Theory, J. Am. Chem. Soc., 2007, 129, 8487–8499 CrossRef CAS PubMed.
- P. Forzatti, E. Tronconi and I. Pasquon, Higher Alcohol Synthesis, Catal. Rev., 1991, 33, 109–168 CrossRef CAS.
- X. Xiaoding, E. B. M. Doesburg and J. J. F. Scholten, Synthesis of higher alcohols from syngas – recently patented catalysts and tentative ideas on the mechanism, Catal. Today, 1987, 2, 125–170 CrossRef.
- H. T. Luk, C. Mondelli, D. C. Ferré, J. A. Stewart and J. Pérez-Ramírez, Status and prospects in higher alcohols synthesis from syngas, Chem. Soc. Rev., 2017, 46, 1358–1426 RSC.
- G. van der Lee, B. Schuller, H. Post, T. L. F. Favre and V. Ponec, On the selectivity of Rh catalysts in the formation of oxygenates, J. Catal., 1986, 98, 522–529 CrossRef CAS.
- M. Suvarna, P. Preikschas and J. Pérez-Ramírez, Identifying Descriptors for Promoted Rhodium-Based Catalysts for Higher Alcohol Synthesis via Machine Learning, ACS Catal., 2022, 12, 15373–15385 CrossRef CAS PubMed.
- R. Lang, T. Li, D. Matsumura, S. Miao, Y. Ren, Y. T. Cui, Y. Tan, B. Qiao, L. Li, A. Wang, X. Wang and T. Zhang, Hydroformylation of Olefins by a Rhodium Single-Atom Catalyst with Activity Comparable to RhCl(PPh3)3, Angew. Chem., Int. Ed., 2016, 55, 16054–16058 CrossRef CAS PubMed.
- J. Amsler, B. B. Sarma, G. Agostini, G. Prieto, P. N. Plessow and F. Studt, Prospects of Heterogeneous Hydroformylation with Supported Single Atom Catalysts, J. Am. Chem. Soc., 2020, 142, 5087–5096 CrossRef CAS PubMed.
- L. Wang, W. Zhang, S. Wang, Z. Gao, Z. Luo, X. Wang, R. Zeng, A. Li, H. Li, M. Wang, X. Zheng, J. Zhu, W. Zhang, C. Ma, R. Si and J. Zeng, Atomic-level insights in optimizing reaction paths for hydroformylation reaction over Rh/CoO single-atom catalyst, Nat. Commun., 2016, 7, 14036 CrossRef CAS PubMed.
- C. Li, W. Wang, L. Yan and Y. Ding, A mini review on strategies for heterogenization of rhodium-based hydroformylation catalysts, Front. Chem. Sci. Eng., 2018, 12, 113–123 CrossRef CAS.
- S. Hanf, L. A. Rupflin, R. Glaser and S. A. Schunk, Current State of the Art of the Solid Rh-Based Catalyzed Hydroformylation of Short-Chain Olefins, Catalysts, 2020, 10, 1–36 CrossRef.
- X.-F. Yang, A. Wang, B. Qiao, J. Li, J. Liu and T. Zhang, Single-Atom Catalysts: A New Frontier in Heterogeneous Catalysis, Acc. Chem. Res., 2013, 46, 1740–1748 CrossRef CAS PubMed.
- A. Wang, J. Li and T. Zhang, Heterogeneous single-atom catalysis, Nat. Rev. Chem., 2018, 2, 65–81 CrossRef CAS.
- I. Ro, J. Qi, S. Lee, M. Xu, X. Yan, Z. Xie, G. Zakem, A. Morales, J. G. Chen, X. Pan, D. G. Vlachos, S. Caratzoulas and P. Christopher, Bifunctional hydroformylation on heterogeneous Rh-WOx pair site catalysts, Nature, 2022, 609, 287–292 CrossRef CAS PubMed.
- B. B. Sarma, J. Jelic, D. Neukum, D. E. Doronkin, X. Huang, S. Bernart, F. Studt and J.-D. Grunwaldt, Tracking and Understanding Dynamics of Atoms and Clusters of Late Transition Metals with In Situ DRIFT and XAS Spectroscopy Assisted by DFT, J. Phys. Chem. C, 2023, 127, 3032–3046 CrossRef CAS.
- L. Liu, D. M. Meira, R. Arenal, P. Concepcion, A. V. Puga and A. Corma, Determination of the Evolution of Heterogeneous Single Metal Atoms and Nanoclusters under Reaction Conditions: Which Are the Working Catalytic Sites?, ACS Catal., 2019, 9, 10626–10639 CrossRef CAS PubMed.
- S. Ding, J. Hülsey Max, H. An, Q. He, H. Asakura, M. Gao, J.-y. Hasegawa, T. Tanaka and N. Yan, Ionic Liquid-Stabilized Single-Atom Rh Catalyst Against Leaching, CCS Chem., 2021, 3, 1814–1822 CrossRef CAS.
- G. Zakem and P. Christopher, Active Site Entropy of Atomically Dispersed Rh/Al2O3 Catalysts Dictates Activity for Ethylene Hydroformylation, ACS Catal., 2023, 13, 5502–5515 CrossRef CAS.
- Y. Zheng, Q. Wang, Q. Yang, S. Wang, M. J. Hülsey, S. Ding, S. Furukawa, M. Li, N. Yan and X. Ma, Boosting the Hydroformylation Activity of a Rh/CeO2 Single-Atom Catalyst by Tuning Surface Deficiencies, ACS Catal., 2023, 13, 7243–7255 CrossRef CAS.
- L. Qi, S. Das, Y. Zhang, D. Nozik, B. C. Gates and A. T. Bell, Ethene Hydroformylation Catalyzed by Rhodium Dispersed with Zinc or Cobalt in Silanol Nests of Dealuminated Zeolite Beta, J. Am. Chem. Soc., 2023, 145, 2911–2929 CrossRef CAS PubMed.
- I. Ro, M. Xu, G. W. Graham, X. Pan and P. Christopher, Synthesis of Heteroatom Rh–ReOx Atomically Dispersed Species on Al2O3 and Their Tunable Catalytic Reactivity in Ethylene Hydroformylation, ACS Catal., 2019, 9, 10899–10912 CrossRef CAS.
- M. Zhao, C. Li, D. Gómez, F. Gonell, V. M. Diaconescu, L. Simonelli, M. L. Haro, J. J. Calvino, D. M. Meira, P. Concepción and A. Corma, Low-temperature hydroformylation of ethylene by phosphorous stabilized Rh sites in a one-pot synthesized Rh-(O)-P-MFI zeolite, Nat. Commun., 2023, 14, 7174 CrossRef CAS PubMed.
- M. Schumann, M. R. Nielsen, T. E. L. Smitshuysen, T. W. Hansen, C. D. Damsgaard, A.-C. A. Yang, M. Cargnello, J.-D. Grunwaldt, A. D. Jensen and J. M. Christensen, Rationalizing an Unexpected Structure Sensitivity in Heterogeneous Catalysis—CO Hydrogenation over Rh as a Case Study, ACS Catal., 2021, 11, 5189–5201 CrossRef CAS.
- N. Yang, A. J. Medford, X. Liu, F. Studt, T. Bligaard, S. F. Bent and J. K. Nørskov, Intrinsic Selectivity and Structure Sensitivity of Rhodium Catalysts for C2+ Oxygenate Production, J. Am. Chem. Soc., 2016, 138, 3705–3714 CrossRef CAS PubMed.
- J. J. Spivey and A. Egbebi, Heterogeneous catalytic synthesis of ethanol from biomass-derived syngas, Chem. Soc. Rev., 2007, 36, 1514–1528 RSC.
- S. R. Foit, I. C. Vinke, L. G. J. de Haart and R.-A. Eichel, Power-to-Syngas: An Enabling Technology for the Transition of the Energy System?, Angew. Chem., Int. Ed., 2017, 56, 5402–5411 CrossRef CAS PubMed.
- D. Pakhare and J. Spivey, A review of dry (CO2) reforming of methane over noble metal catalysts, Chem. Soc. Rev., 2014, 43, 7813–7837 RSC.
- W. Sheng, S. Kattel, S. Yao, B. Yan, Z. Liang, C. J. Hawxhurst, Q. Wu and J. G. Chen, Electrochemical reduction of CO2 to synthesis gas with controlled CO/H2 ratios, Energy Environ. Sci., 2017, 10, 1180–1185 RSC.
- E. Lam and E. Reisner, A TiO2-Co(terpyridine)2 Photocatalyst for the Selective Oxidation of Cellulose to Formate Coupled to the Reduction of CO2 to Syngas, Angew. Chem., Int. Ed., 2021, 60, 23306–23312 CrossRef CAS PubMed.
- M. He, B. Xiao, Z. Hu, S. Liu, X. Guo and S. Luo, Syngas production from catalytic gasification of waste polyethylene: Influence of temperature on gas yield and composition, Int. J. Hydrogen Energy, 2009, 34, 1342–1348 CrossRef CAS.
- V. R. Surisetty, A. K. Dalai and J. Kozinski, Alcohols as alternative fuels: an overview, Appl. Catal., A, 2011, 404, 1–11 CAS.
- M. Ao, G. H. Pham, J. Sunarso, M. O. Tade and S. Liu, Active Centers of Catalysts for Higher Alcohol Synthesis from Syngas: A Review, ACS Catal., 2018, 8, 7025–7050 CrossRef CAS.
- D. Wu, S. Liu, M. Zhong, J. Zhao, C. Du, Y. Yang, Y. Sun, J. Lin, S. Wan, S. Wang, J. Huang, Y. Yao, Z. Li and H. Xiong, Nature and Dynamic Evolution of Rh Single Atoms Trapped by CeO2 in CO Hydrogenation, ACS Catal., 2022, 12, 12253–12267 CrossRef CAS.
- M. Schumann, J.-D. Grunwaldt, A. D. Jensen and J. M. Christensen, Investigations of mechanism, surface species and support effects in CO hydrogenation over Rh, J. Catal., 2022, 414, 90–100 CrossRef CAS.
- M. Deimel, H. Prats, M. Seibt, K. Reuter and M. Andersen, Selectivity Trends and Role of Adsorbate–Adsorbate Interactions in CO Hydrogenation on Rhodium Catalysts, ACS Catal., 2022, 12, 7907–7917 CrossRef CAS.
- S. M. McClure, M. J. Lundwall and D. W. Goodman, Planar oxide supported rhodium nanoparticles as model catalysts, Proc. Natl. Acad. Sci. U. S. A., 2011, 108, 931–936 CrossRef CAS PubMed.
- M. Gupta, M. L. Smith and J. J. Spivey, Heterogeneous Catalytic Conversion of Dry Syngas to Ethanol and Higher Alcohols on Cu-Based Catalysts, ACS Catal., 2011, 1, 641–656 CrossRef CAS.
- K. Fang, D. Li, M. Lin, M. Xiang, W. Wei and Y. Sun, A short review of heterogeneous catalytic process for mixed alcohols synthesis via syngas, Catal. Today, 2009, 147, 133–138 CrossRef CAS.
- R. G. Herman, Advances in catalytic synthesis and utilization of higher alcohols, Catal. Today, 2000, 55, 233–245 CrossRef CAS.
- K. Xiao, Z. Bao, X. Qi, X. Wang, L. Zhong, K. Fang, M. Lin and Y. Sun, Advances in bifunctional catalysis for higher alcohol synthesis from syngas, Chin. J. Catal., 2013, 34, 116–129 CrossRef CAS.
- D. S. Breslow and R. F. Heck, Mechanism of the Hydroformylation of Olefins, Chem. Ind., 1960, 467 CAS.
- R. F. Heck and D. S. Breslow, The Reaction of Cobalt Hydrotetracarbonyl with Olefins, J. Am. Chem. Soc., 1961, 83, 4023–4027 CrossRef.
- S. S. C. Chuang, R. W. Stevens and R. Khatri, Mechanism of C2+ oxygenate synthesis on Rh catalysts, Top. Catal., 2005, 32, 225–232 CrossRef CAS.
- S. S. C. Chuang and S. I. Pien, Infrared study of the CO insertion reaction on reduced, oxidized, and sulfided Rh/SiO2 catalysts, J. Catal., 1992, 135, 618–634 CrossRef CAS.
- H. Xiong, S. Lin, J. Goetze, P. Pletcher, H. Guo, L. Kovarik, K. Artyushkova, B. M. Weckhuysen and A. K. Datye, Thermally Stable and Regenerable Platinum–Tin Clusters for Propane Dehydrogenation Prepared by Atom Trapping on Ceria, Angew. Chem., Int. Ed., 2017, 56, 8986–8991 CrossRef CAS PubMed.
- M. Akri, S. Zhao, X. Li, K. Zang, A. F. Lee, M. A. Isaacs, W. Xi, Y. Gangarajula, J. Luo, Y. Ren, Y.-T. Cui, L. Li, Y. Su, X. Pan, W. Wen, Y. Pan, K. Wilson, L. Li, B. Qiao, H. Ishii, Y.-F. Liao, A. Wang, X. Wang and T. Zhang, Atomically dispersed nickel as coke-resistant active sites for methane dry reforming, Nat. Commun., 2019, 10, 5181 CrossRef PubMed.
- B. B. Sarma, G. Agostini, M. G. Farpón, C. Marini, N. Pfänder and G. Prieto, Bottom-up assembly of bimetallic nanocluster catalysts from oxide-supported single-atom precursors, J. Mater. Chem. A, 2021, 9, 8401–8415 RSC.
- J. C. Matsubu, V. N. Yang and P. Christopher, Isolated Metal Active Site Concentration and Stability Control Catalytic CO2 Reduction Selectivity, J. Am. Chem. Soc., 2015, 137, 3076–3084 CrossRef CAS PubMed.
- A. Aitbekova, L. Wu, C. J. Wrasman, A. Boubnov, A. S. Hoffman, E. D. Goodman, S. R. Bare and M. Cargnello, Low-Temperature Restructuring of CeO2-Supported Ru Nanoparticles Determines Selectivity in CO2 Catalytic Reduction, J. Am. Chem. Soc., 2018, 140, 13736–13745 CrossRef CAS PubMed.
- J.-D. Grunwaldt, L. Basini and B. S. Clausen,
In Situ EXAFS Study of Rh/Al2O3 Catalysts for Catalytic Partial Oxidation of Methane, J. Catal., 2001, 200, 321–329 CrossRef CAS.
- J. Jones, H. Xiong, A. T. DeLaRiva, E. J. Peterson, H. Pham, S. R. Challa, G. Qi, S. Oh, M. H. Wiebenga, X. I. Pereira Hernández, Y. Wang and A. K. Datye, Thermally stable single-atom platinum-on-ceria catalysts via atom trapping, Science, 2016, 353, 150 CrossRef CAS PubMed.
- B. B. Sarma, J. Kim, J. Amsler, G. Agostini, C. Weidenthaler, N. Pfänder, R. Arenal, P. Concepción, P. Plessow, F. Studt and G. Prieto, One-Pot Cooperation of Single-Atom Rh and Ru Solid Catalysts for a Selective Tandem Olefin Isomerization–Hydrosilylation Process, Angew. Chem., Int. Ed., 2020, 59, 5806–5815 CrossRef CAS PubMed.
- C. Galdeano-Ruano, C. W. Lopes, D. Motta Meira, A. Corma and P. Oña-Burgos, Rh2P Nanoparticles Stabilized by Carbon Patches for Hydroformylation of Olefins, ACS Appl. Nano Mater., 2021, 4, 10743–10753 CrossRef CAS.
- R. Koirala, S. E. Pratsinis and A. Baiker, Synthesis of catalytic materials in flames: opportunities and challenges, Chem. Soc. Rev., 2016, 45, 3053–3068 RSC.
- B. B. Sarma and J.-D. Grunwaldt,
Operando Spectroscopy to Understand Dynamic Structural Changes of Solid Catalysts, Chimia, 2024, 78, 288–296 CrossRef CAS PubMed.
- B. B. Sarma, F. Maurer, D. E. Doronkin and J.-D. Grunwaldt, Design of Single-Atom Catalysts and Tracking Their Fate Using Operando and Advanced X-ray Spectroscopic Tools, Chem. Rev., 2023, 123, 379–444 CrossRef CAS PubMed.
- G. Cavusoglu, D. Miao, H. Lichtenberg, H. W. P. Carvalho, H. Xu, A. Goldbach and J.-D. Grunwaldt, Structure and activity of flame made ceria supported Rh and Pt water gas shift catalysts, Appl. Catal., A, 2015, 504, 381–390 CrossRef CAS.
- V. Muravev, G. Spezzati, Y.-Q. Su, A. Parastaev, F.-K. Chiang, A. Longo, C. Escudero, N. Kosinov and E. J. M. Hensen, Interface dynamics of Pd–CeO2 single-atom catalysts during CO oxidation, Nat. Catal., 2021, 4, 469–478 CrossRef CAS.
- W. J. Stark, J.-D. Grunwaldt, M. Maciejewski, S. E. Pratsinis and A. Baiker, Flame-Made Pt/Ceria/Zirconia for Low-Temperature Oxygen Exchange, Chem. Mater., 2005, 17, 3352–3358 CrossRef CAS.
- J. Finzel, K. M. Sanroman Gutierrez, A. S. Hoffman, J. Resasco, P. Christopher and S. R. Bare, Limits of Detection for EXAFS Characterization of Heterogeneous Single-Atom Catalysts, ACS Catal., 2023, 13, 6462–6473 CrossRef CAS.
- L. Basini, M. Marchionna and A. Aragno, Drift and mass spectroscopic studies on the reactivity of rhodium clusters at the surface of polycrystalline oxides, J. Phys. Chem., 1992, 96, 9431–9441 CrossRef CAS.
- F. C. Meunier, Relevance of IR Spectroscopy of Adsorbed CO for the Characterization of Heterogeneous Catalysts Containing Isolated Atoms, J. Phys. Chem. C, 2021, 125, 21810–21823 CrossRef CAS.
- Y. Kwon, T. Y. Kim, G. Kwon, J. Yi and H. Lee, Selective Activation of Methane on Single-Atom Catalyst of Rhodium Dispersed on Zirconia for Direct Conversion, J. Am. Chem. Soc., 2017, 139, 17694–17699 CrossRef CAS PubMed.
- B. B. Sarma, P. N. Plessow, G. Agostini, P. Concepción, N. Pfänder, L. Kang, F. R. Wang, F. Studt and G. Prieto, Metal-Specific Reactivity in Single-Atom Catalysts: CO Oxidation on 4d and 5d Transition Metals Atomically Dispersed on MgO, J. Am. Chem. Soc., 2020, 142, 14890–14902 CrossRef CAS PubMed.
- A. M. Beale and B. M. Weckhuysen, EXAFS as a tool to interrogate the size and shape of mono and bimetallic catalyst nanoparticles, Phys. Chem. Chem. Phys., 2010, 12, 5562–5574 RSC.
- D. A. J. M. Ligthart, R. A. van Santen and E. J. M. Hensen, Influence of particle size on the activity and stability in steam methane reforming of supported Rh nanoparticles, J. Catal., 2011, 280, 206–220 CrossRef CAS.
|
This journal is © The Royal Society of Chemistry 2024 |
Click here to see how this site uses Cookies. View our privacy policy here.