DOI:
10.1039/D4SC02103G
(Edge Article)
Chem. Sci., 2024,
15, 13234-13239
Helicity control of a polyaromatic coordination capsule through stereoselective CH–π interactions†
Received
30th March 2024
, Accepted 29th June 2024
First published on 5th July 2024
Abstract
Although square-planar ML4 units are essential building blocks for coordination cages and capsules, the non-covalent control of the chirality and helicity of the resultant nanostructures is quite difficult. Here we report the helicity control of an M2L4 polyaromatic capsule, formed from metal ions with square-planar coordination geometry and bent bispyridine ligands, through stereoselective CH–π interactions with monosaccharide derivatives. Thanks to host–guest CH–π multi-interactions, one molecule of various permethylated monosaccharides is quantitatively bound by the capsule in water (Ka up to >108 M−1). In the polyaromatic cavity, among them, the selective binding of a β-glucose derivative (>80
:
20 ratio) is demonstrated from a mixture of the α/β-glucoses, through the equatorial-selective recognition of the anomeric (C1) group. A similar stereoselective binding is accomplished from an α/β-galactose mixture. Interestingly, single equatorial/axial configurations on the bound monosaccharides can regulate the helical conformation of the capsule in water, confirmed by CD, NMR, and theoretical analyses. An intense capsule-based Cotton effect is exclusively observed upon encapsulation of the permethylated α-glucose (>20-fold enhancement as compared to the β-glucose derivative), via the induction of a single-handed host helicity to a large extent. Inverse capsule helicity is induced by the binding of a β-galactose derivative under the same conditions.
Introduction
Precise control of the chirality and helicity of supramolecular architectures is receiving increasing attention from the viewpoints of chiroptical synthetic, materials, and analytical chemistry.1,2 Square-planar ML4 units (M = metal ion, L = monodentate ligand) are indispensable building blocks for the design and creation of supramolecular cages and capsules.3 The M(pyridyl)4 unit (Fig. 1a) has been predominantly employed among them, due to suitable binding strength and stability.3–5 Whereas the metal hinge can adopt propeller-like helical conformations, the right- and left-handed forms are in rapid equilibrium in solution (Fig. 1b), in marked contrast to Δ/Λ octahedral
units (L′ = bidentate chelating ligand; Fig. 1c) as non-equilibrium chiral building blocks.6 Therefore, the majority of square-planar metal hinge-based MnLm cages and capsules are obtained as racemic mixtures in solution.7 Their racemization has been exceptionally regulated by covalently embedding chiral or helical units into the host frameworks.8 However, non-covalent interactions, e.g., via solvent/temperature change, counter ion exchange, and guest addition, are typically ineffective for the regulation of such racemic nanostructures,9 owing to the low inversion energies of the square-planar metal sites.
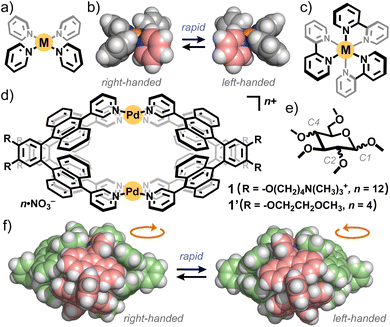 |
| Fig. 1 (a) Representative square-planar ML4 unit and (b) its right/left-handed forms. (c) Representative octahedral unit as a chiral building block. (d) Polyaromatic coordination capsule 1 with ionic side chains (this work) and its analogue 1′ (previous work). (e) Permethylated monosaccharides as water-soluble chiral guests. (f) Right/left-handed helical frameworks of 1′ (R = H for clarity).13b | |
Here we realize the helicity control of M2L4 polyaromatic capsule 1 (Fig. 1d) through non-covalent, stereoselective CH–π multi-interactions with monosaccharide derivatives in water. The coordination capsule provides right- and left-handed helical structures, based on the square-planar metal hinges, as a racemic mixture (Fig. 1f). For the regulation of the capsule helicity, permethylated monosaccharides (Fig. 1e) are employed as chiral guests to maximize host–guest CH–π interactions in the spheroidal polyaromatic cavity. Monosaccharides are ubiquitous biosubstrates with equatorial/axial hydroxy groups.10 Their permethylated derivatives,11 facilely accessed from natural saccharides, are thus suitable guests to study stereoselective CH–π recognition and interactions.12 Using water-soluble capsule 1 (Fig. 1d), we herein report the selective binding of a β-glucose derivative (81
:
19 ratio) from a mixture of α/β-glucoses in water, through the equatorial recognition of a methoxy group on the anomeric (C1) position. A similar stereoselective binding is accomplished from an α/β-galactose mixture. The C4 and C2 groups are also recognized by the capsule, from mixtures of β-glucose/β-galactose and α-glucose/α-mannose derivatives, respectively, with high equatorial selectivity (up to 89
:
11 ratio). Notably, only single equatorial/axial configurations on the monosaccharides can control the right- and left-handed conformations of the capsule, via the efficient guest-induced helicity control of the host framework, which are revealed by the combination of circular dichroism (CD), NMR, and theoretical studies.
A spheroidal polyaromatic cavity (1.0 × 1.5 × 1.5 nm3) is provided by coordination capsule 1′ (Fig. 1d), formed by the quantitative assembly from two Pd(II) ions with square-planar coordination geometry and four bispyridine ligands, bearing two anthracene panels linked by an ortho-phenylene spacer.13a The capsule framework, which is a tightly wound polyaromatic shell, adopts right- and left-handed twist forms in a 1
:
1 ratio, with partially overlapped anthracene units (Fig. 1f). In the polyaromatic cavity, one molecule of planar and bowl-shaped aromatic molecules (e.g., coronene and sumanene) are efficiently encapsulated through multiple aromatic CH–π interactions in organic solvents.13b The shape of the aromatic molecules is selectively recognized through the number of the host–guest interactions in the racemic cavity.13c To facilitate aliphatic CH–π interactions with bio-related substrates, we designed analogous capsule 1 with water solubility (Fig. 1d), featuring eight ionic side chains on the polyaromatic shell. Monosaccharides, focused in this study, adopt multiple stereoisomers derived from the five equatorial/axial substituents on the six-membered ring. Whereas monomethylated β-glucose can be favourably bound over its α-isomer by synthetic covalent cages,14 the equatorial/axial-selective binding of oligomethylated glucose and other saccharides remains to be accomplished so far.3,15
Results and discussion
Preparation of a water-soluble polyaromatic capsule
To study host–guest interactions under aqueous conditions, polyaromatic capsule 1 was prepared from Pd(NO3)2 and dicationic ligand 2, through the simple attachment of pendant trimethylammonium groups on bent bispyridine ligand 3MOE (Fig. 2a and S1–14†).16,17 The quantitative formation of the new coordination capsule, with an M2L4 composition, was confirmed by NMR and MS analyses (Fig. S15–18†).16,17 In the 1H NMR spectra, although ligand 2 showed complicated signals derived from the rotational isomers based on the pyridyl groups, capsule 1 provided highly symmetrical signals with 13 aromatic signals (Ha–i, 8.8–6.5 ppm) and 5 aliphatic signals (Hj–n, 4.2–2.0 ppm; Fig. 2b and c). The desymmetrisation of the anthracene-based signals (He,e′,f,f′,g,g′,h,h′) suggested the existence of right- and left-handed helical capsules, owing to the partial anthracene stacks. The ESI-TOF MS spectrum of 1 showed molecular ion peaks at m/z = 557.53, 660.95, 805.36, and 1022.42, assignable to [1 − n·NO3−]n+ ions (n = 7–4; Fig. 2d and S18†). In the optimized structure of 1, the helical, hydrophobic capsular flamework is surrounded by eight hydrophilic groups (Fig. 2e). Whereas previous capsule 1′ is insoluble in water even at elevated temperature,13b the present one showed adequate water solubility (>10 mM) at room temperature.
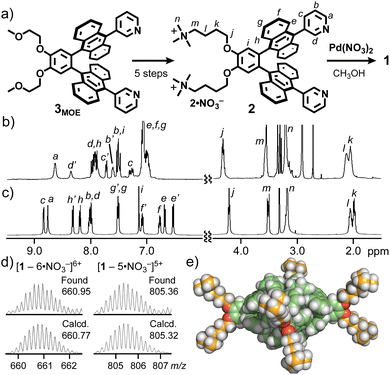 |
| Fig. 2 (a) Synthesis of cationic ligand 2 and the formation of water-soluble polyaromatic capsule 1 as a racemic mixture. 1H MNR spectra (500 MHz, CD3OD, r.t.) of (b) 2 and (c) 1. (d) ESI-TOF MS spectra (CH3OH) of 1 and the calculated isotope patterns. (e) Optimized structure of 1 (DFT calculation, CAM-B3LYP/3-21G). | |
Equatorial-selective binding of monosaccharides in water
The equatorial and axial configuration on D-glucose derivatives could be selectively distinguished by polyaromatic capsule 1 through competitive binding experiments in water. When a mixture of hydrophilic permethylated α-D-glucose (αGlcMe) and β-D-glucose (βGlcMe; 0.20 μmol each) was combined with capsule 1 (0.10 μmol) in D2O (0.5 mL) at 80 °C for 10 min, 1
:
1 host–guest complexes 1·αGlcMe and 1·βGlcMe were formed in 19 and 81% yields based on 1, respectively (Fig. 3a). In the 1H NMR spectra, the multiple aromatic signals of empty 1 (8.9–6.6 ppm) were fully converted to those of the new host–guest complexes (Fig. 3b and c, left), suggesting the quantitative binding of the water-soluble substrates. The complete encapsulation of the substrates was indicated by the appearance of all the methyl groups on αGlcMe (five signals) and βGlcMe (ten signals) in the range of 0.00 to −0.87 ppm (Fig. 3b and c, right). The remarkable upfield shifts (Δδmax = −4.4 ppm) of the signals, relative to those of the free substrates in D2O (3.6–3.4 ppm), are derived from the strong shielding effect in the polyaromatic cavity of 1. On the basis of the methyl signals of 1·αGlcMe and 1·βGlcMe, prepared separately from 1 and αGlcMe or βGlcMe (Fig. 3d and S19–20†), the selective formation of 1·βGlcMe (81
:
19 ratio) was readily estimated by their non-overlapping signal integrals (Fig. S28c†).16 The result revealed that the anomeric (C1) group on the monosaccharides is equatorial-selectively recognized by the present capsule through spheroidal cavity-directed host–guest interactions. The quantitative binding was also performed even at 40 °C for 2 h, with a similar equatorial-selectivity (i.e., an 82
:
18 ratio; Fig. S28d†).16 The selectivity coefficient value (K(βGlcMe)/K(αGlcMe)) of 1 was calculated to be 6.5.
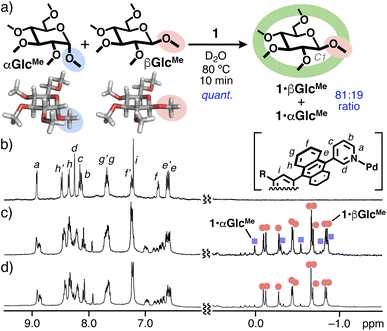 |
| Fig. 3 (a) Selective binding of βGlcMe by capsule 1 in water from a 1 : 1 mixture of αGlcMe and βGlcMe. 1H NMR spectra (500 MHz, D2O, r.t.) of (b) 1, (c) the resultant host–guest complexes obtained from a mixture of 1, αGlcMe, and βGlcMe, and (d) 1·βGlcMe. | |
Similar equatorial-selective interactions were observed between α-D-galactose (αGalMe) and β-D-galactose derivatives (βGalMe) as well as βGlcMe and βGalMe within the polyaromatic capsule. Under the same conditions of the competitive binding study with α/βGlcMe, the treatment of a 1
:
1 mixture of αGalMe and βGalMe with 1 in D2O led to the formation of host–guest complexes 1·βGalMe and 1·αGalMe in an 86
:
14 ratio (Fig. 4a), owing to the recognition of the C1 group. Methyl proton signals derived from 1·βGalMe and 1·αGalMe were again observed in the highly upfield region (from 0.31 to −1.2 ppm; Fig. 4f and S29†). Notably, further high equatorial-preference was demonstrated from a mixture of βGlcMe and βGalMe under the same conditions. The unusual recognition of the C4 group allowed 1 to generate 1·βGlcMe and 1·βGalMe in an 89
:
11 ratio (Fig. 4b, g and S30†).18 Equatorial-selectivity for the C2 group was also found within 1 for an αGlcMe and α-D-mannose (αManMe) mixture (Fig. 4c, h, and S31†).16 Capsule 1 bound αGalMe over mixed α/β-D-fructopyranoses (α/βFruMe), bearing three axial groups, in a 71
:
29 ratio under the same conditions (Fig. 4d, i, and S35†). Notably, the observed high selectivity toward βGlcMe was also supported by a dilution experiment of 1·βGlcMe with water, where the binding constant (Ka) was roughly estimated to >108 M−1 (Fig. S27†).16 The preferential binding ability of the capsule toward βGlcMe over αGlcMe and the other tested substrates is most probably derived from the shape fitting between the spheroidal host cavity and the comparatively planar guest structure (Fig. 3a and 4e).
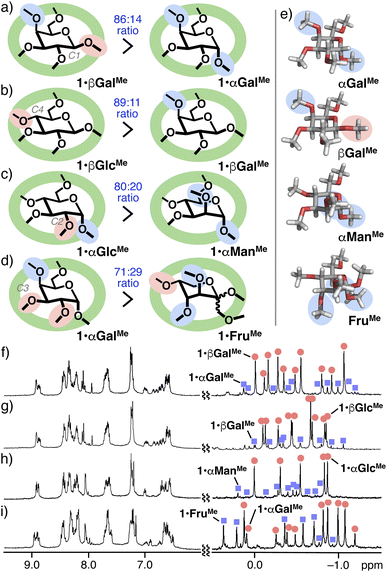 |
| Fig. 4 Selective binding abilities of capsule 1 toward (a) βGalMe from a mixture of βGalMe and αGalMe, (b) βGlcMe from a mixture of βGlcMe and βGalMe, (c) αGlcMe from a mixture of αGlcMe and αManMe, and (d) αGalMe from a mixture of αGalMe and FruMe in water. (e) Optimized structures of αGalMe, βGalMe, αManMe, and FruMe (DFT calculation, B3LYP/6-31G(d,p)). 1H NMR spectra (500 MHz, D2O, r.t.) of host–guest complexes obtained from mixtures of (f) βGalMe and αGalMe, (g) βGlcMe and βGalMe, (h) αGlcMe and αManMe, and (i) αGalMe and FruMe with 1 in water after 10 min at 80 °C. | |
Multiple CH–π interactions in the polyaromatic cavity
To understand the observed quantitative binding of the monosaccharide derivatives with unusually high equatorial-selectivity, host–guest interactions within polyaromatic capsule 1 were quantified by DFT calculations.19 In the optimized structure of 1·βGlcMe, the planar framework of βGlcMe is fully accommodated in the spheroidal cavity of 1 and surrounded by multiple polyaromatic panels (Fig. 5a). The five methyl groups of bound βGlcMe are located close to the eight anthracene rings of 1, with contact distances of ≤3.6 Å (Fig. 5b).19 On the basis of the distances, the presence of 19 CH–π interactions was indicated in the polyaromatic cavity. The optimized structure of 1·αGlcMe, with a slightly hindered axial-methoxy group, also suggested the existence of multiple CH–π interactions (Fig. S37†). The close contacts between the host frameworks (e.g., Hd,e,f) and the guest methyl groups (e.g., HC,D) in the cavity were supported by the NOESY studies of 1·αGlcMe (Fig. S19c†). These host–guest contacts are consistent with the huge upfield shifts of the methyl signals of βGlcMe and αGlcMe within 1 in the 1H NMR spectra (Fig. 3c).
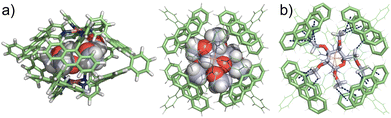 |
| Fig. 5 (a) Optimized structure of (P)-1·βGlcMe (side and top views; R = H for clarity) and (b) the highlighted guest (methyl groups)-host (anthracene panels) CH–π interactions in the cavity as blue dashed lines (distance: ≤3.6 Å). | |
Helicity control through stereoselective host–guest interactions
Notably, unlike previously reported coordination cages with square-planar metal hinges,4,5,7–9 the right/left-handed twists of the present capsule (i.e., (P)/(M)-helical structures) could be exclusively controlled through encapsulation of permethylated α-glucose αGlcMe, which was confirmed by CD, NMR, and theoretical analyses. The UV-visible spectra of 1, 1·αGlcMe, and 1·βGlcMe in water showed comparable absorption bands in the range of 320 to 450 nm (Fig. 6a), derived from the anthracene-based framework of the capsule. In the CD spectra in the range of 300–500 nm, whereas no signal was detected from both empty capsule 1 and free α/βGlcMe, prominent CD signals, with a negative peak at 421 nm and positive peaks at 406, 387, 368 and 330 nm, were observed from host–guest complex 1·αGlcMe (Fig. 6b). The observed, relatively strong Cotton effect in the UV-visible region (θmax = +79.5, λ = 387 nm) is attributed to the helical host framework of 1, induced by binding of the chiral guest in the cavity. In sharp contrast, a relatively weak Cotton effect (θmax = +3.3, λ = 387 nm) was observed in the CD spectrum of 1·βGlcMe under the same conditions. The maximum peak intensity of 1·αGlcMe is >20-fold higher than that of 1·βGlcMe. Therefore, the equatorial or axial configuration on the single glucose C1 group largely controlled the helicity of the capsular polyaromatic framework. The CD studies of host–guest complexes 1·α/βGalMe also revealed the helicity switch of the capsule using galactoses αGalMe and βGalMe. Interestingly, intense positive and negative Cotton effects at 380–420 nm were detected for 1·αGalMe (θmax = +56.4, λ = 386 nm) and 1·βGalMe (θmin = −25.1, λ = 389 nm), respectively (Fig. 6c).
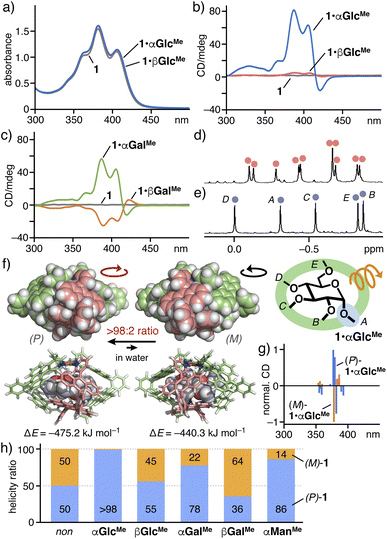 |
| Fig. 6 (a) UV-visible spectra (water, r.t., 0.2 mM based on 1) of 1·αGlcMe, 1·βGlcMe, and 1. CD spectra (water, r.t., 0.2 mM based on 1) of (b) 1·αGlcMe, 1·βGlcMe, and 1, and (c) 1·αGalMe, 1·βGalMe, and 1. 1H NMR spectra (500 MHz, D2O, r.t.) of (d) 1·βGlcMe and (e) 1·αGlcMe. (f) (P)/(M)-helicity control of capsule 1 upon encapsulation of αGlcMe and its optimized structures and energies (R = H for clarity).19 (g) Theoretical CD spectra of (P/M)-1·αGlcMe, calculated by TD-DFT methods (CAM-B3LYP + D3BJ/LanL2DZ (Pd), 6-31G(d,p) (others), PCM (water) level of theory). (h) (P)/(M)-helicity ratio of 1 with αGlcMe, βGlcMe, αGalMe, βGalMe, and αManMe encapsulated in the cavity. | |
The degree and direction of the capsule helicity of 1·αGlcMe were further estimated by the 1H NMR integrals of the methyl signals, derived from the host–guest complexes, and theoretical CD studies. Whereas the proton spectrum of 1·βGlcMe displayed two sets of the bound guest signals (total 10 CH3 signals) in a 1
:
1.2 ratio (Fig. 6d),20 only a single set of those (total 5 CH3 signals; HA–E) appeared in the spectrum of 1·αGlcMe (Fig. 6e), indicating the generation of a single helical structure (>98
:
2 (P)/(M) ratio based on precision for NMR analysis). The 1H NMR spectra of 1·αGalMe, 1·βGalMe, and 1·αManMe showed two sets of the bound guest signals (total 10) in 1
:
3.5, 1.8
:
1, and 1
:
6.2 ratios, respectively (Fig. S21–S23†).16 These results unequivocally revealed the guest-induced formation of 1·αGlcMe as a single-handed helical product to a large extent in water.
The host (P)/(M)-helicity of 1·αGlcMe was elucidated by the theoretical studies of 1·αGlcMe and empty 1. The calculated CD spectrum of (P)-1·αGlcMe obtained by a TD-DFT method displayed intense positive and negative signals at 378–380 and 382 nm, respectively (Fig. 6g). The signal pattern is similar to that of the calculated spectrum of (P)-1 (Fig. S38†).16 Inverse CD signals were found for (M)-1·αGlcMe and (M)-1 (Fig. 6g and S38†). The theoretical and experimental CD analyses indicated that product 1·αGlcMe adopts a (P)/(M)-helical structure in >98
:
2 ratio (Fig. 6f and h). The calculated stabilization energies for the formation of the host–guest complexes (ΔE(1·αGlcMe) = E(1·αGlcMe) – (E(1) + E(αGlcMe))) are in the order of (P)-1·αGlcMe < (M)-1·αGlcMe (ΔΔE(1·αGlcMe) = −34.9 kJ mol−1; Fig. 6f and Table S1†), supporting the predominant generation (>99
:
1 ratio) of the right-handed twist product. These theoretical studies suggested that the (P)-helicity of capsule 1 is also induced by βGlcMe (55
:
45 (P)/(M) ratio based on 1H NMR integrals), αGalMe (78
:
22 ratio),21 and αManMe (86
:
14 ratio), whereas the (M)-helicity is induced by βGalMe (64
:
36 (M)/(P) ratio), as major conformations (Fig. 6h).
Conclusions
We have realized the helicity control of a polyaromatic coordination capsule through stereoselective CH–π interactions with permethylated monosaccharides in water. The aqueous polyaromatic capsule with a spheroidal nanocavity was prepared, from metal ions with square-planar coordination geometry and bent bipyridine ligands with cationic groups, as a racemic mixture. Competitive binding studies revealed that the new capsule encapsulates one molecule of permethylated β-glucose with high selectivity (>80
:
20 ratio), from a mixture of α/β-glucoses, due to the equatorial-selective recognition ability. A similar equatorial-selectivity was observed in the binding of β-galactose over α-galactose and β-glucose over β-galactose derivatives by the capsule. The single equatorial/axial substituent configurations on the saccharides could regulate the (P)/(M)-helicity of the capsule in water. Particularly, an intense Cotton effect was generated upon encapsulation of the α-glucose derivative, unlike the β-glucose, via nearly quantitative induction of the (P)-helical capsule framework. These results deepen the understanding of helicity control of supramolecular nanostructures through aliphatic CH–π multi-interactions, which will expedite the development of novel chiroptical composites and materials. Moreover, we expect that the combination of the present recognition system with fluorophores holds promise for the creation of highly sensitive receptors toward complex chiral biomolecules in water.
Data availability
The experimental procedures and analytical data are available in the ESI.†
Author contributions
N. K., H. S., T. S., and M. Y. designed the work, carried out research, analysed data, and wrote the paper. M. Y. is the principal investigator. All authors discussed the results and commented on the manuscript.
Conflicts of interest
There are no conflicts to declare.
Acknowledgements
This work was supported by JSPS KAKENHI (Grant No. JP22H00348/JP23K17913) and JST-PRESTO (JPMJPR20A7). Theoretical calculations were performed with the support of Dr Yuya Tanaka (Tokyo Institute of Technology) using computers at the Research Center for Computational Science, Okazaki, Japan (23-IMS-C063). N. K. thanks the JSPS for a Research Fellowship for Young Scientists.
Notes and references
- E. Yashima, N. Ousaka, D. Taura, K. Shimomura, T. Ikai and K. Maeda, Chem. Rev., 2016, 116, 13752–13990 CrossRef CAS PubMed.
-
(a) L.-J. Chen, H.-B. Yang and M. Shionoya, Chem. Soc. Rev., 2017, 46, 2555–2576 CAS;
(b) M. Pan, K. Wu, J.-H. Zhang and C.-Y. Su, Coord. Chem. Rev., 2019, 378, 333–349 CrossRef CAS;
(c) F. Begato, G. Licini and C. Zonta, Angew. Chem., Int. Ed., 2023, 62, e202311153 CrossRef CAS.
- Recent reviews:
(a) C. J. Brown, F. D. Toste, R. G. Bergman and K. N. Raymond, Chem. Rev., 2015, 115, 3012–3035 CrossRef CAS;
(b) M. Yoshizawa and L. Catti, Acc. Chem. Res., 2019, 52, 2392–2404 CAS;
(c) A. B. Grommet, M. Feller and R. Klajn, Nat. Nanotechnol., 2020, 15, 256–271 CrossRef CAS PubMed;
(d) E. G. Percastegui, T. K. Ronson and J. R. Nitschke, Chem. Rev., 2020, 120, 13480–13544 CrossRef CAS PubMed;
(e) W. Liu and J. F. Stoddart, Chem, 2021, 7, 919–947 CrossRef CAS;
(f) L. Escobar and P. Ballester, Chem. Rev., 2021, 121, 2445–2514 CrossRef CAS;
(g) H. Takezawa and M. Fujita, Bull. Chem. Soc. Jpn., 2021, 94, 2351–2369 CrossRef CAS;
(h) L. Catti, R. Sumida and M. Yoshizawa, Coord. Chem. Rev., 2022, 460, 214460 CrossRef CAS.
-
(a) N. B. Debata, D. Tripathy and D. K. Chand, Coord. Chem. Rev., 2012, 256, 1831–1945 CrossRef CAS;
(b) K. Harris, D. Fujita and M. Fujita, Chem. Commun., 2013, 49, 6703–6712 RSC;
(c) M. Yoshizawa and L. Catti, Acc. Chem. Res., 2019, 52, 2392–2404 CrossRef CAS PubMed;
(d) S. Pullen, J. Tessarolo and G. H. Clever, Chem. Sci., 2021, 12, 7269–7293 RSC;
(e) L. K. Moree, L. A. V. Faulkner and J. D. Crowley, Chem. Soc. Rev., 2024, 53, 25–46 RSC;
(f) E. Benchimol, J. Tessarolo and G. H. Clever, Nat. Chem., 2024, 16, 13–21 CrossRef CAS PubMed.
- Recent outstanding examples:
(a) D. Fujita, Y. Ueda, S. Sato, H. Yokoyama, N. Mizuno, T. Kumasaka and M. Fujita, Chem, 2016, 1, 91–101 CrossRef CAS;
(b) D. Fujita, Y. Ueda, S. Sato, N. Mizuno, T. Kumasaka and M. Fujita, Nature, 2016, 540, 563–566 CrossRef CAS;
(c) S. Samantray, S. Krishnaswamy and D. K. Chand, Nat. Commun., 2020, 11, 880 CAS;
(d) K. Wu, E. Benchimol, A. Baksi and G. H. Clever, Nat. Chem., 2024, 16, 584–591 CrossRef CAS PubMed.
-
A. von Zelewsky, Stereochemistry of Coordination Compounds, John Wiley & Sons, 1996 Search PubMed.
- Representative M2L4 cages as racemic mixtures:
(a) D. A. McMorran and P. J. Steel, Angew. Chem., Int. Ed., 1998, 37, 3295–3297 CrossRef CAS;
(b) J. D. Crowley and E. L. Gavey, Dalton Trans., 2010, 39, 4035–4037 RSC;
(c) N. Kishi, Z. Li, K. Yoza, M. Akita and M. Yoshizawa, J. Am. Chem. Soc., 2011, 133, 11438–11441 CrossRef CAS PubMed;
(d) W. M. Bloch, S. Horiuchi, J. J. Holstein, C. Drechsler, A. Wuttke, W. Hiller, R. A. Mata and G. H. Clever, Chem. Sci., 2023, 14, 1524–1531 RSC.
-
(a) C. Gütz, R. Hovorka, C. Klein, Q.-Q. Jiang, C. Bannwarth, M. Engeser, C. Schmuck, W. Assenmacher, W. Mader, F. Topić, K. Rissanen, S. Grimme and A. Lützen, Angew. Chem., Int. Ed., 2014, 53, 1693–1698 CrossRef;
(b) K. Wu, K. Li, Y. J. Hou, M. Pan, L. Y. Zhang, L. Chen and C. Y. Su, Nat. Commun., 2016, 7, 10487 CrossRef CAS;
(c) G. H. Clever, T. R. Schulte and J. J. Holstein, Angew. Chem., Int. Ed., 2019, 58, 5562–5566 CrossRef PubMed;
(d) X. Tang, H. Jiang, Y. Si, N. Rampal, W. Gong, C. Cheng, X. Kang, D. Fairen-Jimenez, Y. Cui and Y. Liu, Chem, 2021, 7, 2771–2786 CrossRef CAS.
- Coordination (counter ion)-induced helical cages: I. Regeni, B. Chen, M. Frank, A. Baksi, J. J. Holstein and G. H. Clever, Angew. Chem., Int. Ed., 2021, 60, 5673–5678 CrossRef CAS.
-
T. K. Lindhorst, Essentials of Carbohydrate Chemistry and Biochemistry, John Wiley & Sons, 2007 Search PubMed.
- Permethylated monosaccharide synthesis: T.-R. Li, F. Huck, G. Piccini and K. Tiefenbacher, Nat. Chem., 2022, 14, 985–995 CrossRef PubMed.
-
(a)
M. Nishio, M. Hirota and Y. Umezawa, The CH/π Interaction: Evidence, Nature, and Consequences, Wiley-VCH, 1998 Search PubMed;
(b) H. Takahashi, S. Tsuboyama, Y. Umezawa, K. Honda and M. Nishio, Tetrahedron, 2000, 56, 6185–6191 CrossRef CAS;
(c) M. Nishio, Phys. Chem. Chem. Phys., 2011, 13, 13873–13900 RSC.
-
(a) L. Catti, N. Kishida, T. Kai, M. Akita and M. Yoshizawa, Nat. Commun., 2019, 10, 1948 CrossRef;
(b) N. Kishida, K. Matsumoto, Y. Tanaka, M. Akita, H. Sakurai and M. Yoshizawa, J. Am. Chem. Soc., 2020, 142, 9599–9603 CrossRef CAS;
(c) N. Kishida, Y. Tanaka and M. Yoshizawa, Chem. - Eur. J., 2022, 28, e202202075 CrossRef CAS PubMed.
-
(a) C. Ke, H. Destecroix, M. P. Crump and A. P. Davis, Nat. Chem., 2012, 4, 718–723 CrossRef CAS;
(b) R. A. Tromans, T. S. Carter, L. Chabanne, M. P. Crump, H. Li, J. V. Matlock, M. G. Orchard and A. P. Davis, Nat. Chem., 2019, 11, 52–56 CrossRef CAS PubMed;
(c) W. Liu, Y. Tan, L. O. Jones, B. Song, Q.-H. Guo, L. Zhang, Y. Qiu, Y. Feng, X.-Y. Chen, G. C. Schatz and J. F. Stoddart, J. Am. Chem. Soc., 2021, 143, 15688–15700 CrossRef CAS PubMed.
- M. Yamashina, M. Akita, T. Hasegawa, S. Hayashi and M. Yoshizawa, Sci. Adv., 2017, 3, e1701126 CrossRef.
- See the ESI.†.
- Dicationic ligand 2 was synthesized from previously reported ligand 3MOE in 27% overall yield (5 steps) using etherification, halogen exchange, and Menschutkin reaction. Capsule 1 formed upon mixing ligand 2 (31 μmol), PdCl2(DMSO)2 (16 μmol), and AgNO3 (33 μmol) in methanol (0.5 mL) for 3 h at 60 °C.
- Selective binding of biomolecules:
(a) M. Yamashina, T. Tsutsui, Y. Sei, M. Akita and M. Yoshizawa, Sci. Adv., 2019, 5, eaav3179 CrossRef CAS PubMed;
(b) K. Niki, T. Tsutsui, M. Yamashina, M. Akita and M. Yoshizawa, Angew. Chem., Int. Ed., 2020, 59, 10489–10492 CrossRef CAS;
(c) R. Sumida, Y. Tanaka, K. Niki, Y. Sei, S. Toyota and M. Yoshizawa, Chem. Sci., 2021, 12, 9946–9951 RSC;
(d) M. Shuto, R. Sumida, M. Yuasa, T. Sawada and M. Yoshizawa, JACS Au, 2023, 3, 2905–2911 CrossRef CAS PubMed;
(e) R. Sumida, L. Catti and M. Yoshizawa, ACS Org. Inorg., 2024 DOI:10.1021/acsorginorgau.4c00013.
- The optimized structures of 1, 1·αGlcMe, and 1·βGlcMe were obtained by DFT calculations (R = H; CAM-B3LYP+D3BJ/LanL2DZ (Pd), 3-21G (others) level of theory). The CH⋯π distances were estimated by measuring the distances between the hydrogen atoms of guest CH groups and the ring centres of the host anthracene panels in the optimized structures.
- No correlation signals between the guests within the (P)/(M)-helical capsules were observed in the EXSY NMR spectra of 1·βGalMe in D2O at room temperature, because of no (P)–(M) equilibrium (slower than 1.0 s–1 inversion rate) under the NMR conditions (mixing time: 1.0, 0.1, and 0.01 s; Fig. S39†).
- The CD intensity-based (P)/(M) ratios of 1·βGlcMe and 1·αGalMe were estimated to be 52
:
48 and 85
:
15, respectively, which are comparable to their (P)/(M) ratios based on the 1H NMR integrals (Fig. 6h).
|
This journal is © The Royal Society of Chemistry 2024 |