DOI:
10.1039/D4SC01780C
(Edge Article)
Chem. Sci., 2024, Advance Article
Identification of two-dimensional covalent organic frameworks with mcm topology and their application in photocatalytic hydrogen evolution†
Received
16th March 2024
, Accepted 11th May 2024
First published on 13th May 2024
Abstract
Covalent organic frameworks have attracted considerable attention in recent years as a distinct class of crystalline porous organic materials. Their functional properties are inherently linked to their structural characteristics. Although hundreds of COFs have been reported so far, the types of their topologic structure are still limited. In this article, we report the identification of mcm topology for three porphyrin-based two-dimensional COFs, which are constructed from [4 + 4] imine condensation reactions. The mcm net is generated by pentagonal tiling, which has not been identified for COFs before. The structure of the COFs is elucidated by a variety of experimental characterization and structural simulations, by which their reticular frameworks exclusively composed of pentagonal pores have been confirmed. Moreover, the COFs exhibit high performance in photocatalytic hydrogen evolution from water, with the best one up to 10.0 mmol g−1 h−1 after depositing 0.76 wt% Pt as a co-catalyst. This study identifies mcm topology for COFs for the first time and highlights the potential of these COFs as promising photocatalysts for sustainable hydrogen production from water.
Introduction
Covalent organic frameworks (COFs) represent a class of crystalline porous organic polymers constructed via interconnecting organic building blocks with covalent bonds, guided by the principle of reticular chemistry.1–3 The physical and chemical properties of COFs are significantly influenced by their network structures, which are mainly classified into two categories: two-dimensional (2D) and three dimensional (3D) frameworks according to the spatial extension of interconnections of building blocks.4–7 The combination of building blocks with different symmetries may lead to the formation of COFs with various topologies, from which different shapes of pores can be generated. Currently more than 20 topologies have been experimentally realized for 3D COFs.8–10 However, as far as the types of 2D COFs are concerned, to the best of our knowledge, so far only 13 topologies, including hcb,11 hxl,12 sql,13 kgm,14 kgd,15 tth,16 mtf,17 bex,18 fxt,19 cpi,20 htb,21 cem,22 and tju23 nets, have been identified or proposed for the COFs reported in the literature. Among these topologies, most of the pore shapes are triangular, quadrangular, or hexagonal.11–19,21–23 Surprisingly, pentagonal pores are rarely observed in COFs and currently only the cpi net includes pentagonal faces but they are tiled with hexagonal faces.20 COFs with mcm topology, that is, those that are composed of only pentagonal pores, have not been identified yet, although such a topology has already been theoretically proposed for COFs and predicted to exhibit unique properties.24,25 The difficulty in the construction of such a type of COF could be attributed to the nonedge-transitive feature of mcm topology, for which both the angles and lengths of its building blocks need to match each other.16 This requirement poses a challenge to the design and construction of this kind of COF.
As a class of functional porous materials, COFs have exhibited great application potential in diverse fields, including gas storage/separation,26–30 heterogeneous catalysis,31–33 energy storage,34–37 sensors,38,39 optoelectronics,40–43 and so on. Among them, COFs as photocatalysts for the hydrogen evolution reaction (HER) from water have drawn considerable attention in recent years because of their structural advantages, such as high crystallinity, designable structure, highly accessible surface areas, abundant photo-active sites, and efficient transport of photogenerated charges.44 Since Lotsch and co-workers reported the first example of applying a COF for the HER in 2014,45 a variety of COFs have been developed for the photocatalytic HER and the efficiency of the photocatalysts has become higher and higher over the past several years.46–48 However, to explore high performance photocatalysts for the HER, increasing their structure diversity is still urgent. Herein, we report the synthesis of three porphyrin-based 2D COFs. Their topology was identified as a mcm net based on structure elucidation. Moreover, their photocatalytic performance for hydrogen evolution from water was investigated, and a high catalytic efficiency was observed.
Results and discussion
The synthesis of the three COFs was performed through the Schiff-base reaction of C4 symmetric tetraamine tetrakis(4-aminophenyl)porphyrin with different metal ions (TAPP-M) and D2h symmetric tetraaldehyde 4,4′,4′′,4′′′-([1,1′-biphenyl]-4,4′-diylbis(azanetriyl))tetrabenzaldehyde (BATA) under solvothermal conditions (Scheme 1). The reaction conditions were optimized by screening various solvents and aqueous acetic acid solutions to afford the COFs as microcrystallites with high crystallinity. Specifically, COF-BATA-TAPP-H2 was obtained as crystalline purple powder by heating the monomers in a mixture of mesitylene and n-butanol with 6 M aqueous acetic acid as a catalyst at 120 °C for 72 h, while COF-BATA-TAPP-Ni and COF-BATA-TAPP-Zn were synthesized by a similar method but with different organic solvents and different concentrations of aqueous acetic acid (see the ESI† for details). The Fourier transform infrared (FT-IR) spectra of these COFs showed a significant decrease in the intensity of the bands corresponding to C
O and N–H stretching vibrations (1695 and 3360 cm−1, respectively) in comparison with those of the monomers BATA and TAPP (Fig. S1†). Meanwhile, the appearance of C
N stretching vibration at 1626 cm−1 in the FT-IR spectra of the three COFs indicated the successful polymerization and the consumption of the monomers. Moreover, the formation of C
N bonds in the polymers was also supported by the peaks with chemical shifts at ∼160 ppm in the solid-state 13C cross-polarization magic angle spinning (CP/MAS) NMR spectra of the COFs (Fig. S2–S4†). In addition, X-ray photoelectron spectroscopy (XPS) measurements were carried out to evaluate the content and valence state of metals in COF-BATA-TAPP-M. The experimental values of metal content for the two COFs are 1.2 at%, which matched well with the theoretical values (Table S1†). The strong peaks in the XPS spectrum of COF-BATA-TAPP-Ni, which appeared at 855.46 eV and 872.69 eV, should be attributed to Ni 2p3/2 and Ni 2p1/2. The peaks that appeared at 1021.87 eV and 1044.88 eV in the XPS spectrum of COF-BATA-TAPP-Zn should be attributed to Zn 2p3/2 and Zn 2p1/2. These results indicated that the valence state of Ni and Zn in the COFs was +2 (Fig. S5–7†). The morphology of the COFs was observed with transmission electron microscopy (TEM). The recorded TEM images show that all three COFs exhibit a sheet-like morphology (Fig. S8–S10†), consistent with their 2D structures. Their thermal stability was investigated with thermogravimetric analysis. Their 5% weight loss was observed at 540, 504, and 448 °C for COF-BATA-TAPP-H2, COF-BATA-TAPP-Ni, and COF-BATA-TAPP-Zn, respectively, indicating that they all have high thermal stability (Fig. S11†).
 |
| Scheme 1 Synthesis of COF-BATA-TAPP-M. | |
After the acquisition of the polymers, structural simulations and powder X-ray diffraction (PXRD) analysis were conducted to elucidate their crystal structures. In principle, two types of frameworks with bex topology and mcm topology, respectively, could be produced from the assembly of a cross-like C4 symmetric building block (I) and a D2h symmetric building block (II) (Fig. 1a). The difference in the spatial locations of the building blocks between the bex net and mcm net lies in the different arrangement of the building block II. In a COF with bex topology, the building block II adopts a parallel array. However, in a COF with mcm topology, the building block II is arranged in a perpendicular position. It should be noted that structures with bex topology have been depicted for all the previously reported COFs constructed from the combination of these two kinds of building blocks,49–59 including two COFs synthesized from the same monomers as the ones in this work but under different solvothermal conditions.60,61 Indeed, most of the COFs in these previous studies should hold bex topology, not mcm, because the length ratio of their building blocks does not meet the requirement for the formation of the mcm net, which is explained as follows. As shown in Fig. 1a, the mcm net has 2 kinds of vertex (A and B), 2 kinds of edge (m and n), 3 kinds of corner (α, β, and γ), and one kind of face. The connection number of vertex A is 3 and the connection number of vertex B is 4. According to the structure of vertex B, the angle of γ should be 90°. For the angles of α and β, they can vary but need to keep 2α + β = 360°. To favor the design of building blocks in COFs, both the angles of α and β are fixed to 120°, because organic molecules with such a structure are readily synthesized. For this design, the as-proposed pentagon pore has three 120° corners and two 90° corners, with four edge m and one edge n. On the basis of the geometry of this structure, the length ratio of edge m and edge n (rm/n) is calculated to be 1.37. Therefore, to construct a mcm net, the building blocks must be judiciously selected to make rm/n equal or close to 1.37. The values of rm/n of some building blocks were calculated and are shown in Fig. 1b. The results show that the former three cannot form COFs with mcm topology because the values of rm/n are far from 1.37. Indeed, the second and third monomers experimentally led to the formation of COFs with bex topology,52,56 an edge-transitive net for which only angle matching is required.16 In the case of the combination of TAPP and BATA, the rm/n is 1.56, which is close to the theoretical value of 1.37. As a result, a COF with mcm topology can be expected from their condensation under suitable conditions.
 |
| Fig. 1 (a) Illustration for the formation of COFs with mcm (left) and bex (right) topologies from the assembly of building blocks I and II, and the relationship between the vertex, edge, and angle in mcm topology (middle). (b) Illustration to show the length ratios of the edges to fit or unfit the formation of a COF with mcm topology. | |
To elucidate the crystal structures of the as-synthesized polymers, theoretical PXRD patterns of the COFs with both bex and mcm nets were simulated and compared with the experimentally observed PXRD data. As shown in Fig. 2a, although the two structures with different topologies display similar theoretical PXRD patterns, a noticeable difference can still be identified at the position of 2θ = 5.46°, where the mcm structure has a clear diffraction peak but such a peak is absent for the bex structure. The experimental PXRD pattern collected for COF-BATA-TAPP-Zn displays an intense peak at 4.87° (200 facet). Notably, a peak was observed at 5.46°, which corresponds to the (210) facet of the COF with mcm topology, strongly suggesting that the as-obtained COF possesses a mcm net. In addition, diffraction peaks were also observed at 2.50°, 3.48°, and 9.85°, which are assigned to (100), (110), and (400) facets, respectively. Pawley refinement performed on its experimental PXRD pattern against an eclipsed stacking model (Fig. 2b) afforded unit cell parameters of a = 37.60 Å, b = 37.17 Å, c = 4.91 Å, and α = β = γ = 90° (Rwp = 5.40% and Rp = 4.12%). COF-BATA-TAPP-H2 and COF-BATA-TAPP-Ni displayed PXRD patterns similar to that of COF-BATA-TAPP-Zn. Although their (210) peaks are weak, they could still be identified (Fig. S12 and S13†), suggesting that they also hold a mcm net. The cell parameters of COF-BATA-TAPP-H2 and BATA-TAPP-Ni were also obtained by Pawley refinement, which are a = 37.70 Å, b = 37.96 Å, c = 4.22 Å, and α = β = γ = 90° (Rwp = 4.65% and Rp = 3.65%) for the former, and a = 37.66 Å, b = 37.43 Å, c = 4.95 Å, and α = β = γ = 90° (Rwp = 4.08% and Rp = 3.15%) for the latter, respectively.
 |
| Fig. 2 (a) Experimental (black) and Pawley refined (red) PXRD patterns, and their difference (gray), and simulated PXRD patterns for BATA-TAPP-Zn with the mcm net (blue) and bex net (green). (b) Lattice structure of BATA-TAPP-Zn from top and side views (gray, carbon and zinc; blue, nitrogen; white, hydrogen). (c) Nitrogen adsorption–desorption isotherms of the three COFs. (d) Pore size distribution profile of COF-BATA-TAPP-Zn. | |
Another piece of evidence for the formation of the COFs with mcm topology was provided by experimental results from nitrogen adsorption–desorption measurements. As shown in Fig. 2c, all of the three COFs displayed typical type-I sorption isotherms and steep nitrogen uptakes in the low-pressure range (P/P0 = 0–0.01), indicating a permanent microporous feature. Brunauer–Emmett–Teller (BET) surface areas were calculated to be 1696, 1280, and 1417 m2 g−1 for COF-BATA-TAPP-H2, COF-BATA-TAPP-Ni, and COF-BATA-TAPP-Zn, respectively (Fig. S14†). Pore size distributions calculated based on the quenched solid density functional theory (QSDFT) method showed narrow distributions at 16.1 Å (COF-BATA-TAPP-Zn), 16.7 Å (COF-BATA-TAPP-H2), and 16.1 Å (COF-BATA-TAPP-Ni) (Fig. 2d and S15†). These values agree well with the theoretical pore sizes of the COFs with mcm topology (15.0 Å, Scheme 1), but are different from that predicted for the COFs with bex topology, which possess two different kinds of pores with apertures of 10.2 and 16.6 Å (Fig. S16†).
The orderly arranged porphyrin arrays in the crystalline frameworks might endow them with excellent photocatalytic activity. Thus the potential of the three COFs as photocatalysts was investigated. For this purpose, their optical and electronic properties were studied first. Their ultraviolet-visible (UV-vis) diffuse reflectance spectra show that light across the UV to visible spectrum could be absorbed by the COFs, with absorption edges ranging from 600 to 700 nm (Fig. 3a). The optical band gaps of COF-BATA-TAPP-H2, COF-BATA-TAPP-Ni, and COF-BATA-TAPP-Zn were calculated to be 1.79, 1.91, and 1.87 eV, respectively, based on the corresponding Tauc plots (Fig. S17†). The narrow range of these values (1.79–1.91 eV) suggests that the optical band gaps of these COFs are not significantly influenced by the different metalation of the porphyrin unit. The Mott–Schottky plots reveal that the three COFs are n-type semiconductors and their flat band potentials were calculated to be −1.38, −1.40, and −1.17 V (vs. Ag/AgCl) for COF-BATA-TAPP-H2, COF-BATA-TAPP-Ni, and COF-BATA-TAPP-Zn, respectively (Fig. 3b–d). An energy level diagram is presented in Fig. 3e. The lowest unoccupied molecular orbital (LUMO) energy levels were calculated to be approximately −3.28 to −3.49 eV, higher than the potential of the HER, indicating that these COFs have the potential for the photocatalytic HER from water. In addition, the valence band XPS analysis of the three COFs gave similar results (Fig. S18 and Table S2†).
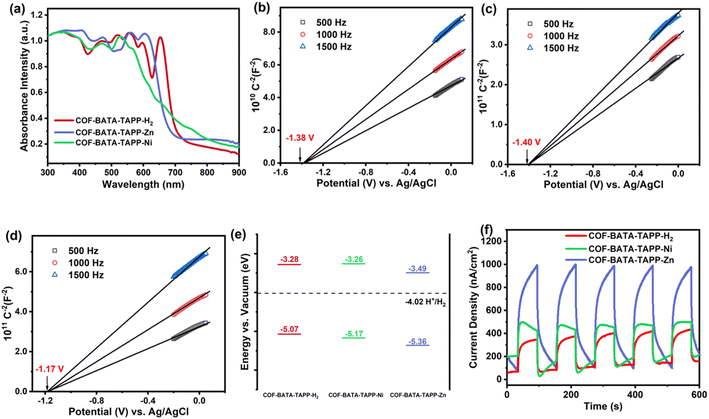 |
| Fig. 3 (a) UV-vis adsorption spectra of COF-BATA-TAPP-H2 (red), COF-BATA-TAPP-Ni (green), and COF-BATA-TAPP-Zn (blue). Mott–Schottky plots of (b) COF-BATA-TAPP-H2, (c) COF-BATA-TAPP-Ni, and (d) COF-BATA-TAPP-Zn. (e) Comparison of the frontier orbital energies of the COFs and the redox potential of water. (f) Photocurrent of COF-BATA-TAPP-H2 (red), COF-BATA-TAPP-Ni (green), and COF-BATA-TAPP-Zn (blue). | |
To further investigate the carrier separation and migration behaviour of the three COFs, photoelectrochemical measurements were performed. According to the results obtained from electrochemical impedance spectroscopy (EIS, Fig. S19†), COF-BATA-TAPP-Zn has the smallest arc radius among the three COFs, indicating the lowest charge-transfer resistance. The arc radius of all three COFs decreases after irradiation, which is attributed to the generation of photoinduced charge carriers. From the results of the linear sweep voltammetry (LSV) experiment (Fig. S20†), COF-BATA-TAPP-Zn displays a much higher current density than the other two COFs in the dark and under visible-light. The current density of all three COFs is enhanced after light irradiation, which is concordant with the EIS results. Photocurrent tests were further conducted for all three COFs to evaluate their photoelectric responses. The experimental results show that the photoelectric responses display an order of COF-BATA-TAPP-Zn > COF-BATA-TAPP-Ni > COF-BATA-TAPP-H2 (Fig. 3f). Besides that, the intensity enhancement of the signals in the electron paramagnetic resonance (EPR) spectra of COF-BATA-TAPP-H2 and COF-BATA-TAPP-Zn under light also indicated the generation of photoinduced charge carriers (Fig. S21 and S22†). These outcomes suggest that the introduction of metal ions into the porphyrin unit improves the charge carrier transport ability of the COFs, with COF-BATA-TAPP-Zn displaying the most efficient charge carrier transport.
The photocatalytic performance of the three COFs was evaluated for hydrogen evolution from water under a visible-light source (Xe-lamp 300 W, λ > 400 nm) in the presence of ascorbate acid and H2PtCl6, with ascorbate acid employed as a sacrificial reagent and H2PtCl6 serving as a precursor of the co-catalyst. Because of its best photocurrent performance, COF-BATA-TAPP-Zn was selected for optimizing the conditions for photocatalytic H2 evolution. A certain amount of Pt, which facilitated the dissociation of photogenerated exciton and proton coupling, was in situ photodeposited on the COF surface during the reaction. It was found that the H2 evolution rate of COF-BATA-TAPP-Zn was significantly influenced by the amount of Pt (Fig. S23†). When Pt was absent as the co-catalyst, no obvious H2 evolution was detected by gas chromatography. With the amount of Pt was increased from 0 to 0.76 wt%, the H2 evolution rate of the COF gradually rose to 10.0 mmol g−1 h−1. However, upon further increasing the amount of Pt to 5.71 wt%, the H2 evolution rate of COF-BATA-TAPP-Zn dropped to 3.3 mmol g−1 h−1. Such a reduction in the H2 evolution rate was attributed to the excessive Pt loading, which would hinder light absorption and often serve as a major site for charge-carrier recombination.62 The content of deposited Pt on COF-BATA-TAPP-Zn was optimized to be 0.76 wt% to achieve a maximum H2 evolution rate. Subsequently, the H2 evolution performance of the other two COFs was measured under the same conditions. The trend in H2 evolution performance was observed as COF-BATA-TAPP-Zn (10.0 mmol g−1 h−1) > COF-BATA-TAPP-Ni (3.2 mmol g−1 h−1) > COF-BATA-TAPP-H2 (1.7 mmol g−1 h−1) (Fig. 4a), consistent with the results of the photoelectric response experiment. Compared with other COF and POP-based HER photocatalysts previously reported, COF-BATA-TAPP-Zn displayed a good HER ability with a low amount of Pt co-catalyst (Table S3†). Furthermore, the photocatalytic stability of COF-BATA-TAPP-Zn was evaluated by adding a certain amount of ascorbic acid into the reaction system every 4 hours. It was observed that the reaction system maintained robust durability in photocatalytic hydrogen evolution over the course of five recycle experiments (Fig. 4b), indicating that the COF exhibited good photocatalytic stability. Moreover, a comparison of the FT-IR spectra of COF-BATA-TAPP-Zn before and after the photocatalytic reaction showed no obvious difference, indicating structural integration of the COF during photocatalysis (Fig. S24†).
 |
| Fig. 4 (a) Time course for photocatalytic H2 production under visible irradiation for COF-BATA-TAPP-H2 (red), COF-BATA-TAPP-Ni (green), and COF-BATA-TAPP-Zn (blue). (b) Photocatalytic H2 production with COF-BATA-TAPP-Zn in five recycles. | |
In these COFs, the eclipsed interlayer stacking results in well-ordered porphyrin columns, forming macrocycle-on-macrocycle and metal-on-metal channels, which play pivotal roles in charge carrier separation and migration. In the case of COF-BATA-TAPP-Zn, holes could readily migrate via the macrocycle-on-macrocycle channel to the surface of the COF, while electrons could transfer via the Zn⋯Zn chain.63 In COF-BATA-TAPP-Ni, the decrease of d-electrons in the central metal-ion (3d8 for Ni2+) introduces the possibility of the ligand-to-metal charge transfer (LMCT) process, which would restrain hole migration via the macrocycle-on-macrocycle channel. This increases the possibility of charge recombination. For COF-BATA-TAPP-H2, photogenerated electron and hole migration would proceed solely via the macrocycle-on-macrocycle pathway due to the absence of metal ions, leading to the highest probability of charge recombination.63 Therefore, COF-BATA-TAPP-Zn displayed a much higher H2 evolution ability than COF-BATA-TAPP-Ni and COF-BATA-TAPP-H2. Additionally, TEM analysis showed that Pt nanoparticles (NPs) in situ formed with the COFs under light irradiation. Remarkably, COF-BATA-TAPP-Zn displayed a significantly higher abundance of Pt NPs, with an average size of about 1.5 nm. This size is notably smaller than the average size of Pt NPs observed for COF-BATA-TAPP-H2 and COF-BATA-TAPP-Ni (about 3 nm, Fig. S25–27†). The superior H2 evolution ability of COF-BATA-TAPP-Zn over the other two COFs could partly be attributed to the co-existence of the uniform dispersion of Pt NPs.
Conclusions
In summary, mcm topology, whose structure is solely tiled by pentagons, has been identified for COFs for the first time. Three COFs were constructed through imine condensation reactions of a porphyrin-based tetraamines and a D2h symmetric tetraaldehyde. It is the matched angle and length of the building blocks that lead to the formation of the 2D COFs with a mcm net. The COFs comprise only pentagonal pores, which is a new shape complement to the COFs with homogeneous porosity, which mainly comprise triangular, quadrilateral, or hexagonal pores. Thanks to the well-ordered arrays of the porphyrin units in the COFs and the conjugated 2D frameworks, the COFs exhibit impressive photocatalytic ability for hydrogen evolution from water, with the highest hydrogen evolution rate up to 10.0 mmol g−1 h−1. These findings not only increase the diversity of the topological structure of COFs, but also underscore the significance of structural design for COFs with targeted topology and function, which should be instructive for the development of novel and high-performance crystalline porous materials.
Data availability
The data have been provided in ESI.†
Author contributions
X. Z., P. T. and X. H. conceived and designed the experiments. P. T. and X. H. performed the experiments. Q. Q. aided in the structural simulations. X. Z. and P. T. wrote the manuscript. X. Z. directed the research.
Conflicts of interest
There are no conflicts to declare.
Acknowledgements
We thank the National Natural Science Foundation of China (No. 21632004) and State Key Laboratory of Advanced Technology for Materials Synthesis and Processing (Wuhan University of Technology) for financial support (2023-KF-6). We would like to thank Professor Shengqiang Xiao (Wuhan University of Technology) for assisting with the PXRD measurements.
Notes and references
- S. Kandambeth, K. Dey and R. Banerjee, J. Am. Chem. Soc., 2019, 141, 1807–1822 CrossRef CAS PubMed.
- K. Geng, T. He, R. Liu, S. Dalapati, K. T. Tan, Z. Li, S. Tao, Y. Gong, Q. Jiang and D. Jiang, Chem. Rev., 2020, 120, 8814–8933 CrossRef CAS PubMed.
- R.-R. Liang, S.-Y. Jiang, R.-H. A and X. Zhao, Chem. Soc. Rev., 2020, 49, 3920–3951 RSC.
- B. Gui, H. Ding, Y. Cheng, A. Mal and C. Wang, Trends Chem., 2022, 4, 437–450 CrossRef CAS.
- A. M. Evans, M. J. Strauss, A. R. Corcos, Z. Hirani, W. Ji, L. S. Hamachi, X. Aguilar-Enriquez, A. D. Chavez, B. J. Smith and W. R. Dichtel, Chem. Rev., 2022, 122, 442–564 CrossRef CAS PubMed.
- X. Guan, F. Chen, Q. Fang and S. Qiu, Chem. Soc. Rev., 2020, 49, 1357–1384 RSC.
- Please note that a few one-dimensional (1D) COFs have also been reported recently. For examples, please see: C. Jia, A. Duan, C. Liu, W.-Z. Wang, S.-X. Gan, Q.-Y. Qi, Y. Li, X. Huang and X. Zhao, Small, 2023, 19, 2300518 CrossRef CAS PubMed , and the references therein..
- W. Liu, K. Wang, X. Zhan, Z. Liu, X. Yang, Y. Jin, B. Yu, L. Gong, H. Wang, D. Qi, D. Yuan and J. Jiang, J. Am. Chem. Soc., 2023, 145, 8141–8149 CrossRef CAS PubMed.
- X. Guan, F. Chen, S. Qiu and Q. Fang, Angew. Chem., Int. Ed., 2023, 62, e202213203 CrossRef CAS PubMed.
- L. Liao, X. Guan, H. Zheng, Z. Zhang, Y. Liu, H. Li, L. Zhu, S. Qiu, X. Yao and Q. Fang, Chem. Sci., 2022, 13, 9305–9309 RSC.
- A. P. Côté, A. I. Benin, N. W. Ockwig, M. O'Keeffe, A. J. Matzger and O. M. Yaghi, Science, 2005, 310, 1166–1170 CrossRef PubMed.
- S. Dalapati, M. Addicoat, S. Jin, T. Sakurai, J. Gao, H. Xu, S. Irle, S. Seki and D. Jiang, Nat. Commun., 2015, 6, 7786 CrossRef CAS PubMed.
- E. L. Spitler and W. R. Dichtel, Nat. Chem., 2010, 2, 672–677 CrossRef CAS PubMed.
- T.-Y. Zhou, S.-Q. Xu, Q. Wen, Z.-F. Pang and X. Zhao, J. Am. Chem. Soc., 2014, 136, 15885–15888 CrossRef CAS PubMed.
- S.-Q. Xu, R.-R. Liang, T.-G. Zhan, Q.-Y. Qi and X. Zhao, Chem. Commun., 2017, 53, 2431–2434 RSC.
- B. Zhang, H. Mao, R. Matheu, J. A. Reimer, S. A. Alshmimri, S. Alshihri and O. M. Yaghi, J. Am. Chem. Soc., 2019, 141, 11420–11424 CrossRef CAS PubMed.
- H. L. Nguyen, N. Hanikel, S. J. Lyle, C. Zhu, D. M. Proserpio and O. M. Yaghi, J. Am. Chem. Soc., 2020, 142, 2218–2221 CrossRef CAS PubMed.
- T. Banerjee, F. Haase, S. Trenker, B. P. Biswal, G. Savasci, V. Duppel, I. Moudrakovski, C. Ochsenfeld and B. V. Lotsch, Nat. Commun., 2019, 10, 2689 CrossRef PubMed.
- C. Qian, Q.-Y. Qi, G.-F. Jiang, F.-Z. Cui, Y. Tian and X. Zhao, J. Am. Chem. Soc., 2017, 139, 6736–6743 CrossRef CAS PubMed.
- S.-Y. Jiang, S.-X. Gan, X. Zhang, H. Li, Q.-Y. Qi, F.-Z. Cui, J. Lu and X. Zhao, J. Am. Chem. Soc., 2019, 141, 14981–14986 CrossRef CAS PubMed.
- S.-L. Cai, Z.-H. He, X.-L. Li, K. Zhang, S.-R. Zheng, J. Fan, Y. Liu and W.-G. Zhang, Chem. Commun., 2019, 55, 13454–13457 RSC.
- X. Wang, X. Han, C. Cheng, X. Kang, Y. Liu and Y. Cui, J. Am. Chem. Soc., 2022, 144, 7366–7373 CrossRef CAS PubMed.
- Z. Yang, W. Hao, X. Su, T. Zhang, W. Chen, G. Zhang and L. Chen, Chem. Mater., 2022, 34, 5888–5895 CrossRef CAS.
- Y. Lan, X. Han, M. Tong, H. Huang, Q. Yang, D. Liu, X. Zhao and C. Zhong, Nat. Commun., 2018, 9, 5274 CrossRef PubMed.
- H. Q. Pham and N.-N. Pham-Tran, Chem. Mater., 2021, 33, 4488–4499 CrossRef CAS.
- Y. Zeng, R. Zou and Y. Zhao, Adv. Mater., 2016, 28, 2855–2873 CrossRef CAS PubMed.
- J. Fu, S. Das, G. Xing, T. Ben, V. Valtchev and S. Qiu, J. Am. Chem. Soc., 2016, 138, 7673–7680 CrossRef CAS PubMed.
- C. J. Doonan, D. J. Tranchemontagne, T. G. Glover, J. R. Hunt and O. M. Yaghi, Nat. Chem., 2010, 2, 235–238 CrossRef CAS.
- Q. Gao, L. Bai, X. Zhang, P. Wang, P. Li, Y. Zeng, R. Zou and Y. Zhao, Chin. J. Chem., 2015, 33, 90–94 CrossRef CAS.
- L. Liu, Y. Hu, S. Huang, Y. Jin, J. Cui, W. Gong and W. Zhang, Chem. Sci., 2021, 12, 13316–13320 RSC.
- X. Han, Q. Xia, J. Huang, Y. Liu, C. Tan and Y. Cui, J. Am. Chem. Soc., 2017, 139, 8693–8697 CrossRef CAS PubMed.
- Z. Fu, C. Shu, X. Wang, L. Chen, X. Wang, L. Liu, K. Wang, R. Clowes, S. Y. Chong, X. Wu and A. I. Cooper, CCS Chem., 2023, 5, 2290–2300 CrossRef CAS.
- W. Wang, H. Wang, X. Tang, J. Huo, Y. Su, C. Lu, Y. Zhang, H. Xu and C. Gu, Chem. Sci., 2022, 13, 8679–8685 RSC.
- M. Dogru, M. Handloser, F. Auras, T. Kunz, D. Medina, A. Hartschuh, P. Knochel and T. Bein, Angew. Chem., Int. Ed., 2013, 52, 2920–2924 CrossRef CAS.
- Y. Du, H. Yang, J. M. Whiteley, S. Wan, Y. Jin, S. H. Lee and W. Zhang, Angew. Chem., Int. Ed., 2016, 55, 1737–1741 CrossRef CAS PubMed.
- S. Wan, J. Guo, J. Kim, H. Ihee and D. Jiang, Angew. Chem., Int. Ed., 2008, 47, 8826–8830 CrossRef CAS PubMed.
- S. Wang, Q. Wang, P. Shao, Y. Han, X. Gao, L. Ma, S. Yuan, X. Ma, J. Zhou, X. Feng and B. Wang, J. Am. Chem. Soc., 2017, 139, 4258–4261 CrossRef CAS.
- S. Dalapati, S. Jin, J. Gao, Y. Xu, A. Nagai and D. Jiang, J. Am. Chem. Soc., 2013, 135, 17310–17313 CrossRef CAS PubMed.
- S.-Y. Ding, M. Dong, Y.-W. Wang, Y.-T. Chen, H.-Z. Wang, C.-Y. Su and W. Wang, J. Am. Chem. Soc., 2016, 138, 3031–3037 CrossRef CAS.
- N. Huang, X. Ding, J. Kim, H. Ihee and D. Jiang, Angew. Chem., Int. Ed., 2015, 54, 8704–8707 CrossRef CAS.
- M. Dogru and T. Bein, Chem. Commun., 2014, 50, 5531–5546 RSC.
- R. Li, G. Xing, H. Li, S. Li and L. Chen, Chin. Chem. Lett., 2023, 34, 107454 CrossRef CAS.
- D. W. Burke, R. R. Dasari, V. K. Sangwan, A. K. Oanta, Z. Hirani, C. E. Pelkowski, Y. Tang, R. Li, D. C. Ralph, M. C. Hersam, S. Barlow, S. R. Marder and W. R. Dichtel, J. Am. Chem. Soc., 2023, 145, 11969–11977 CrossRef CAS PubMed.
- L. Dai, A. Dong, X. Meng, H. Liu, Y. Li, P. Li and B. Wang, Angew. Chem., Int. Ed., 2023, 62, e202300224 CrossRef CAS PubMed.
- L. Stegbauer, K. Schwinghammer and B. V. Lotsch, Chem. Sci., 2014, 5, 2789–2793 RSC.
- L. Sun, M. Lu, Z. Yang, Z. Yu, X. Su, Y. Q. Lan and L. Chen, Angew. Chem., Int. Ed., 2022, 61, e202204326 CrossRef CAS PubMed.
- R. Shen, C. Qin, L. Hao, X. Li, P. Zhang and X. Li, Adv. Mater., 2023, 35, 2305397 CrossRef CAS PubMed.
- T. Zhou, L. Wang, X. Huang, J. Unruangsri, H. Zhang, R. Wang, Q. Song, Q. Yang, W. Li, C. Wang, K. Takahashi, H. Xu and J. Guo, Nat. Commun., 2021, 12, 3934 CrossRef CAS PubMed.
- Y. Li, G. Cui, X. Cai, G. Yun, Y. Zhao, L. Jiang, S. Cui, J. Zhang, M. Liu, W. Zeng, Z. Wang and J. Jiang, Chem.–Eur. J., 2024, 30, e202303688 CrossRef CAS PubMed.
- A. Chatterjee, J. Sun, K. S. Rawat, V. Van Speybroeck and P. Van Der Voort, Small, 2023, 19, 2303189 CrossRef CAS PubMed.
- L. Zhang, Y. Xiao, Q.-C. Yang, L.-L. Yang, S.-C. Wan, S. Wang, L. Zhang, H. Deng and Z.-J. Sun, Adv. Funct. Mater., 2022, 32, 2201542 CrossRef CAS.
- L. Gong, X. Yang, Y. Gao, G. Yang, Z. Yu, X. Fu, Y. Wang, D. Qi, Y. Bian, K. Wang and J. Jiang, J. Mater. Chem. A, 2022, 10, 16595–16601 RSC.
- J.-Y. Yue, Y.-T. Wang, X. Wu, P. Yang, Y. Ma, X.-H. Liu and B. Tang, Chem. Commun., 2021, 57, 12619–12622 RSC.
- Q. Wu, R.-K. Xie, M.-J. Mao, G.-L. Chai, J.-D. Yi, S.-S. Zhao, Y.-B. Huang and R. Cao, ACS Energy Lett., 2020, 5, 1005–1012 CrossRef CAS.
- H. Lv, R. Sa, P. Li, D. Yuan, X. Wang and R. Wang, Sci. China: Chem., 2020, 63, 1289–1294 CrossRef CAS.
- S. Bhunia, S. K. Das, R. Jana, S. C. Peter, S. Bhattacharya, M. Addicoat, A. Bhaumik and A. Pradhan, ACS Appl. Mater. Interfaces, 2017, 9, 23843–23851 CrossRef CAS PubMed.
- H. He, X. Fang, D. Zhai, W. Zhou, Y. Li, W. Zhao, C. Liu, Z. Li and W. Deng, Chem.–Eur. J., 2021, 27, 14390–14395 CrossRef CAS PubMed.
- S. An, C. Lu, Q. Xu, C. Lian, C. Peng, J. Hu, X. Zhuang and H. Liu, ACS Energy Lett., 2021, 6, 3496–3502 CrossRef CAS.
- Please note that some of the structures reported in ref. 49–58 look like a sql net, especially when the length of building block II is short. For example, please see ref. 55 and 57.
- M. Liu, S. Liu, C. X. Cui, Q. Miao, Y. He, X. Li, Q. Xu and G. Zeng, Angew. Chem., Int. Ed., 2022, 61, e202213522 CrossRef CAS PubMed.
- Y. Zhu, D. Zhu, Y. Chen, Q. Yan, C. Y. Liu, K. Ling, Y. Liu, D. Lee, X. Wu, T. P. Senftle and R. Verduzco, Chem. Sci., 2021, 12, 16092–16099 RSC.
- J. B. Sambur, T. Y. Chen, E. Choudhary, G. Chen, E. J. Nissen, E. M. Thomas, N. Zou and P. Chen, Nature, 2016, 530, 77–80 CrossRef CAS PubMed.
- R. Chen, Y. Wang, Y. Ma, A. Mal, X. Y. Gao, L. Gao, L. Qiao, X. B. Li, L. Z. Wu and C. Wang, Nat. Commun., 2021, 12, 1354 CrossRef CAS PubMed.
|
This journal is © The Royal Society of Chemistry 2024 |