DOI:
10.1039/D3SC06998B
(Review Article)
Chem. Sci., 2024,
15, 5897-5915
Single-atom and cluster catalysts for thermocatalytic ammonia synthesis at mild conditions
Received
30th December 2023
, Accepted 7th March 2024
First published on 8th March 2024
Abstract
Ammonia (NH3) is closely related to the fields of food and energy that humans depend on. The exploitation of advanced catalysts for NH3 synthesis has been a research hotspot for more than one hundred years. Previous studies have shown that the Ru B5 sites (step sites on the Ru (0001) surface uniquely arranged with five Ru atoms) and Fe C7 sites (iron atoms with seven nearest neighbors) over nanoparticle catalysts are highly reactive for N2-to-NH3 conversion. In recent years, single-atom and cluster catalysts, where the B5 sites and C7 sites are absent, have emerged as promising catalysts for efficient NH3 synthesis. In this review, we focus on the recent advances in single-atom and cluster catalysts, including single-atom catalysts (SACs), single-cluster catalysts (SCCs), and bimetallic-cluster catalysts (BCCs), for thermocatalytic NH3 synthesis at mild conditions. In addition, we discussed and summarized the unique structural properties and reaction performance as well as reaction mechanisms over single-atom and cluster catalysts in comparison with traditional nanoparticle catalysts. Finally, the challenges and prospects in the rational design of efficient single-atom and cluster catalysts for NH3 synthesis were provided.
1. Introduction
The excessive consumption of conventional fossil fuels in the modern industrial world has led to the increasing pressure of energy crisis and environmental pollution on human society.1 To achieve the goal of zero carbon emissions in 2060, there is an urgent need to develop and utilize renewable and environmentally friendly energy sources so as to move towards a low-carbon society and economy. However, sustainable and renewable energy face a compatibility issue with the current large-scale and established energy infrastructure because of their intrinsic intermittence and fluctuation. The application of energy storage carrier is an efficient route to solve the utilization of renewable energy. It is general knowledge that hydrogen serves as a clean energy. Recently, with the green generation of electricity using renewable sources (such as hydro, wind, solar and tidal), it becomes economically acceptable to use the H2 produced by water electrolysis. However, high pressure transportation (∼20 MPa) currently hinders its large-scale application.2 Therefore, it is important to develop hydrogen production, storage and transportation systems that allow hydrogen to be distributed and utilized in a safe manner.3
Ammonia (NH3) is an important chemical product with a wide range of applications in modern society, such as the production of fertilizers, pharmaceuticals, explosives, and other nitrogen-containing compounds.4–6 In addition, NH3 is also a promising carbon-free energy storage carrier for storing intermittent renewable energy sources.7,8 Compared to liquid hydrogen and other organic liquid hydrogen energy carriers (such as toluene and cyclohexane),3 NH3 is preferred as an energy carrier due to its high energy density (4.32 kW h L−1), high gravimetric (17.6 wt%) and volumetric (121 kg m−3) hydrogen densities, and ease of liquefaction under mild conditions.9–12 Moreover, the storage and transportation systems of liquid NH3 are mature and safe.9 Currently, the industrial production of NH3 is mainly through the Haber–Bosch (HB) process using Fe-based catalysts at high temperatures (490–510 °C) and high pressures (15–30 MPa).13,14 To meet the global demand for NH3, approximately 200 million tons of NH3 are produced annually using the HB process, which consumes about 1–2% of the global annual energy supply and also results in significant carbon dioxide emissions.5,15–17 With the maturity of water electrolysis technology driven by renewable electric power, the carbon emission problem in NH3 synthesis will be solved properly by coupling water electrolysis for H2 production technology with the electrolysis-driven HB (eHB) process.18–20 The present bottleneck is that NH3 cannot be synthesized under mild conditions, resulting in mismatch with the pressure of the electrolysis system employed for H2 production (<5 MPa, mostly in the range of 1.0–3.2 MPa). Accordingly, the design and development of efficient NH3 synthesis catalysts under mild conditions are keenly needed.
The main bottleneck for the synthesis of NH3 is the activation of the inert N2, primarily due to its high N
N triple bond energy (941 kJ mol−1), lack of a permanent dipole, a large HOMO–LOMO gap (10.8 eV) and a high ionization energy (15.58 eV),23,24 making it difficult to activate under mild conditions. At present, the activation route of N2 has been widely studied, which involves either the associative or dissociative route. In both photocatalytic and electrocatalytic synthesis of NH3, the mild reaction conditions cannot realize the direct dissociation of the N
N triple bond while enabling the hydrogenation of N2via an associative mechanism with the assistance of an external field (Fig. 1a and b).25–27 For alternate hydrogenation, the two N atoms are hydrogenated by protons to form *NH–NH and *NH2–NH2 species, whereas for distal hydrogenation, protons are bonded to one N atom to form the *N–NH2 and *N–NH3 species. Comparatively, due to the existence of certain temperature and pressure in the thermocatalytic NH3 synthesis, N2 is more likely to form NH3via the dissociative mechanism, whereby the adsorbed N2 dissociates directly into the adsorbed N atoms (2N), followed by stepwise hydrogenation to form NH3 (Fig. 1c). As early as 1934, Emmett and Brunauer speculated that the formation rate of NH3 should be approximately equal to the dissociation rate of N2 on the surface of the iron-based catalysts.28 Subsequently, Ertl and his colleagues further confirmed this viewpoint through a series of surface characterization techniques on Fe-based catalysts, and they revealed the process of NH3 synthesis via a dissociative mechanism (Fig. 1d).22,29,30 Concurrently, the dissociative adsorption of N2 is generally considered as the rate-determining step (RDS) for NH3 synthesis, which is closely related to unique active sites on the transition metal surface, such as the C7 sites of Fe.31,32 The so-called C7 active sites mean seven adjacent Fe atoms on the surface of an Fe single crystal.33,34 Somorjai and Strongin et al. studied the high-pressure NH3 synthesis on different crystal faces of Fe single crystal and found that the NH3 synthesis activity presented in the following order: Fe (111) > Fe (211) > Fe (100) > Fe (210) > Fe (110).31,34–37 The catalytic activity of Fe (111) and (100) surfaces was ca. 418 and 25-fold higher than that of tightly packed Fe (110) surfaces, respectively, indicating that the NH3 synthesis over Fe catalysts is a structure-sensitive reaction. The high activity of NH3 synthesis at Fe (111) was mainly attributed to the presence of a large number of C7 active sites, which facilitated the significant weakening of the π bond in N
N and promoted its dissociation.31 The dissociative mechanism on the Fe (111) surface was also further verified by Nørskov et al. using density functional theory (DFT) calculations.38
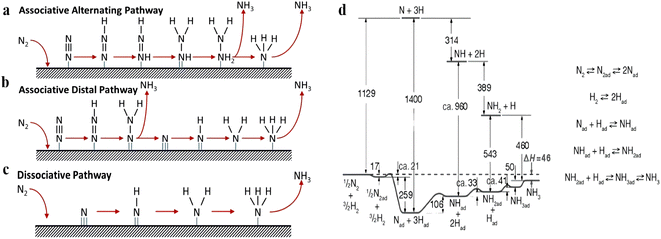 |
| Fig. 1 (a) Associative alternating pathway, (b) associative distal pathway, and (c) dissociative pathway for NH3 synthesis on heterogeneous catalysts.21 (d) Mechanism and potential energy diagram of NH3 synthesis on the iron surface.22 | |
Compared with Fe-based catalysts, the NH3 synthesis over Ru is considered to be more structurally sensitive through DFT calculations and extensive studies on Ru single crystals.39–41 Moreover, N2 dissociation on Ru (0001) surface was considered to be entirely dominated by the B5 sites,39,40 which exposed a three-fold hollow site with a bridge site in close proximity to assure two nitrogen atoms of N2 not bonding to the same Ru atom. The concentration of the B5 sites strongly depends on the size and shape of Ru nanoparticles (Ru NPs), with the highest concentration of B5 active sites in 1.8–2.5 nm Ru NPs, whereas almost no B5 active sites exist when the particle size of Ru NPs is less than 1.8 nm.33,42,43 In the past few decades, numerous efforts have been made to develop various high-performance transition metal catalysts to lower the dissociation energy of N2, but only a few novel catalysts have been able to shift the bottleneck from slow N2 dissociation to the formation of N–Hx (x = 1–3).44–46 In recent years, theoretical and experimental studies have demonstrated that in thermocatalytic NH3 synthesis, when the particle size of metal nanoparticles is reduced to the sub-nanometer clusters, atomic clusters or even single atom level, in which effective sites for N2 dissociation cannot be formed; N2 is inclined to be activated via an associative mechanism for NH3 synthesis.47–49 It has been shown that controlling active metal in the form of subnano clusters or single atoms is an efficient method to improve their catalytic activity and utilization.49–51 Therefore, transition metal catalysts at the nanoscale, sub-nanoscale, especially at the atomic level, have received widespread attention in the field of NH3 synthesis in recent years.
In this review, the recent progress in single-atom and cluster catalysts, including SACs, SCCs, and BCCs, for thermocatalytic NH3 synthesis is presented for both the theoretical development and experimental practice (Schemes 1 and 2). The unique properties and reaction mechanisms over single-atom and cluster catalysts compared with traditional nanoparticle catalysts are discussed and summarized. In addition, we also discuss the current challenges and future prospects of the design of single-atom and cluster catalysts for NH3 synthesis under mild conditions. The purpose of this review is to deepen the fundamental understanding and give guidance for the rational design of advanced catalysts for NH3 synthesis.
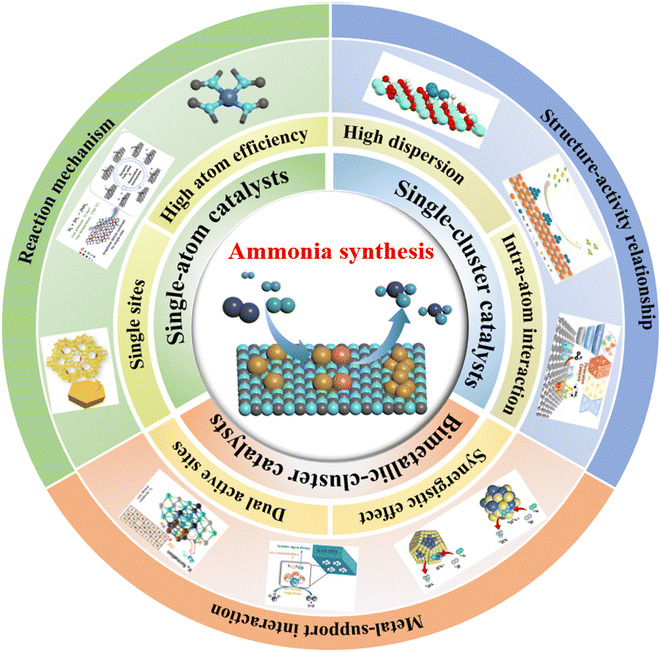 |
| Scheme 1 Schematic for single-atom and cluster catalysts for thermocatalytic NH3 synthesis. | |
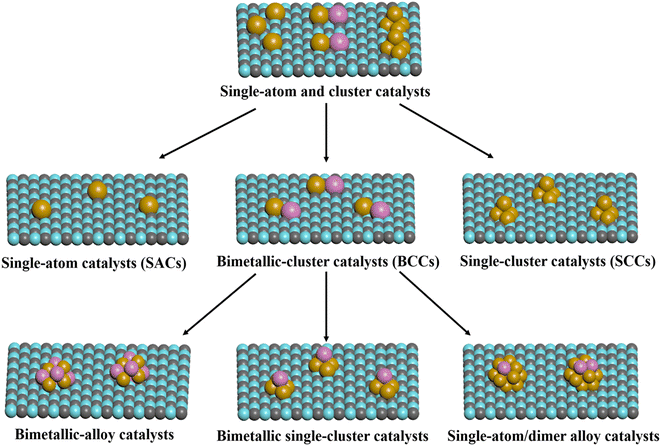 |
| Scheme 2 Schematic diagram of classification over single-atom and cluster catalysts. | |
2. SACs for NH3 synthesis
In recent years, SACs have garnered widespread attention in the field of heterogeneous catalysis for their maximum atom efficiency and excellent catalytic performance, mainly due to the significant advantages of SACs in terms of activity, selectivity, and tunable interactions between metal atoms and supports.52–54 Currently, there are various synthesis methods for SACs, such as high-temperature pyrolysis,55 co-precipitation,56 one-pot synthesis,57 and atomic layer deposition (ALD).58 However, isolated metal atoms with high surface free energy are prone to migrate and aggregate under reaction conditions. Therefore, various supports including carbon materials, nitrogen-doped carbon-based materials, metal oxides, and zeolites are typically employed in the preparation process to disperse and stabilize metal atoms.59–61 Besides, according to the property of active metal in SACs for NH3 synthesis, it can be simply classified as noble metal SACs and non-noble metal SACs.
2.1 Noble metal SACs
Noble metals, which are active for NH3 synthesis, are mainly represented by Os and Ru. Therein, the research and application of Os is limited because of its scarcity, while Ru-based catalysts are regarded as the second-generation catalysts for NH3 synthesis. Due to the high cost of the noble metal Ru, developing Ru SACs can maximize its atomic efficiency while providing well-defined single-atom sites for mechanism study.62 Qiu et al. prepared pure siliceous zeolite-supported Ru single-atom catalyst (Ru SAs/S-1) using hydrothermal and vacuum decomposition methods (Fig. 2a).63 At 523–648 K and 0.1 MPa, the NH3 synthesis rate of Ru SAs/S-1 was higher than that of the traditional Cs–Ru/MgO catalyst with similar Ru content (Fig. 2b), indicating that the single Ru active site exhibited excellent catalytic performance, which was associated with its distinct N2 activation pathway. The N2 reaction order of Ru SAs/S-1 was determined to be 0.15, which was much lower than that of traditional Ru-based catalysts (close to unity), indicating that the dissociation of N2 was no longer the rate-determining step.64 Moreover, the H2 reaction order over Ru SAs/S-1 is a positive value (0.36), which was different from the generally negative value for conventional Ru catalysts. DFT calculations indicated that N2 molecules can be linearly adsorbed in the exposed Ru single-atom active sites and reacted with H2 molecules physically adsorbed in the zeolite channels to form NH3via a distal pathway of associative mechanism (Fig. 2c). Similarly, Li et al. employed HZSM-5 (HZ) zeolite with a rich microporous structure to serve as an anchoring site for stabilizing Ru single atoms (Ru/HZ SAC) by integrating a microwave single mode and ion exchange method.65 Ru/HZ SAC with 0.2 wt% Ru exhibited excellent NH3 synthesis rate of 4.67 mmolNH3 gcat−1 h−1 at 350 °C and 1 MPa, which was approximately 20-fold higher than that of Ru/HZ NCs (Fig. 2d). Moreover, the apparent activation energy for NH3 synthesis over Ru/HZ SAC (38 kJ mol−1) (Fig. 2e) was significantly less than that of the conventional Ru-based catalysts (80–130 kJ mol−1),66,67 indicating the different reaction mechanism over the Ru/HZ SAC catalyst for NH3 synthesis. DFT calculations indicated that the direct dissociation of N2 into 2N on a single Ru site required a high energy barrier of 2.90 eV, while the hydrogenation via a distal pathway of associative mechanism to form *NNH2 required only 1.12 eV (Fig. 2f), indicating that the dissociation adsorption pathway on Ru SACs was not favorable for NH3 synthesis.
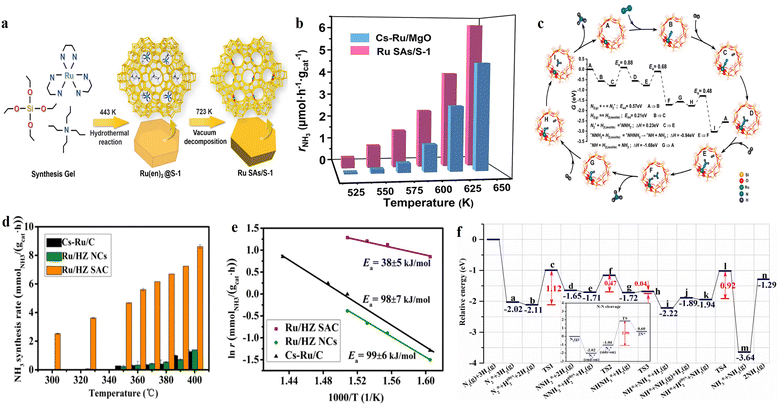 |
| Fig. 2 (a) Synthesis procedure of Ru SAs/S-1. (b) Temperature dependence of NH3 synthesis rate over Ru SAs/S-1 and Cs–Ru/MgO varying from 523 to 648 K. (c) DFT calculation of NH3 synthesis reaction pathway over the Ru SAs/S-1 catalyst.63 (d) NH3 synthesis rate and (e) Arrhenius plots of Ru/HZ SAC and Ru/HZ NCs catalysts as well as the Cs–Ru/C reference. (f) Potential energy diagrams for NH3 synthesis on Ru/HZ SAC via the formation of NNH2* as intermediates.65 | |
2.2 Non-noble metal SACs
Compared with noble metal Ru-based catalysts, the development of non-noble metal single-atom catalysts are more attractive for industrial applications. Notably, carbon materials or N-doped carbon materials have been frequently reported as excellent supports for SACs due to their large specific surface area, tunable electronic structure, and electron transfer capability.68 Wang et al. reported the N-doped carbon-supported Co SACs (Co–N–C) for NH3 synthesis under mild conditions.48 At 350 °C and 1 MPa, the NH3 synthesis rate of the Co–N–C catalyst was 4.34 mmolNH3 gcat−1 h−1, which was 11-and 17-fold higher than that of Co/C and N–C, respectively (Fig. 3a). The outstanding performance of Co–N–C for NH3 synthesis was attributed to the synergistic effect of dynamic and steady-state dual-active sites. The stable Co1–N3.5 active site formed by the coordination of Co with the pyrrolic N in the support facilitated the activation of N2via the alternate pathway of associative mechanism, resulting in the stepwise hydrogenation to form *HNNH, *NH–NH3, and *NH2–NH4 species, followed by the cleavage of the N–N bond in *NH2–NH4 species to release NH3 (Fig. 3b). Moreover, the pyridine N species, which interacted less strongly with Co, can react with adsorbed H2 to form NH3via a chemical looping pathway (Fig. 3c). To investigate the influence of different coordination environments in SACs on NH3 synthesis performance, Zhou et al. prepared atomically dispersed Co-based catalysts with different Co–N coordination numbers by varying the pyrolysis temperatures.69 With the increase in the pyrolysis temperature, the coordination number of Co–N decreased gradually. Co SACs with Co–N4, Co–N3, and Co–N2 coordination structures were obtained at 450, 550 and 650 °C, respectively (Fig. 3d and e). Compared with Co–N4 and Co–N3 catalysts, the Co–N2 catalyst with the lowest coordination number exhibited the best catalytic activity, with an NH3 synthesis rate of 85.3 mmolNH3 gCo−1 h−1 at 300 °C and 1 MPa (Fig. 3f). Various characterizations and DFT calculations elucidated that Co SAC with low coordination number could generate more unoccupied Co 3d charges and tetrahedral cobalt(II) sites, which can promote the electron transfer for N2 activation and the desorption N-containing intermediate species, respectively, thus resulting in a high NH3 synthesis rate. Similarly, Li et al. studied Co SACs with different Co–N coordination numbers and also showed that Co–N2 catalysts with two coordination N atoms exhibited the best NH3 synthesis performance,70 which was attributed to the fact that Co SACs with low Co–N coordination were conducive to N2 adsorption and activation.
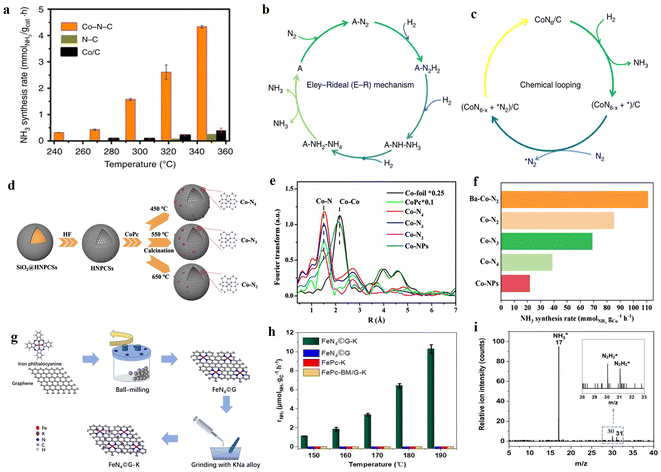 |
| Fig. 3 (a) NH3 synthesis rate for Co–N–C, Co/C, and N–C under 1 MPa. (b) NH3 synthesis pathway on single Co sites in the form of steady-state Co1–N3.5 (A represents the active sites); (c) NH3 production on dynamic cyclic sites via the chemical-looping pathway (x is in the range of 0 < x ≤ 1.5 and VN* represents an anionic nitrogen vacancy).48 (d) Schematic diagram of the preparation process of Co–Nx with different CNs. (e) Co K-edge EXAFS spectra of Co–Nx catalysts. (f) NH3 synthesis rate over the as-synthesized Co–Nx catalysts at 1 MPa and 300 °C. (g) Scheme for the preparation of the graphene lattice-confined Fe-single-site catalyst FeN4©G-K. (h) Production rates of FeN4©G-K, FeN4©G, FePc-K, and FePc-BM/G-K as a function of reaction temperature at 0.1 MPa. (i) The effluents monitored by in NH3 synthesis over FeN4©G-K at 180 °C, 0.1 MPa, and WHSV of 30 L gc−1 h−1.71 | |
In addition to Co-based single-atom catalysts, Fe- or Mo-based single-atom catalysts also exhibited excellent NH3 synthesis performance. Chen et al. successfully confined the FeN4 structure to the lattice of graphene by co-milling FePc and graphene at 450 rpm for 20 h (Fig. 3g).71 The FeN4©G-K catalyst with 24.5 wt% K showed an excellent catalytic activity under mild conditions, with NH3 synthesis rates of 1.1 and 10.3 μmolNH3 gc−1 h−1 at 150 °C and 190 °C, respectively (Fig. 3h). This study results revealed that the effective active site of the FeN4©G-K catalyst for N2 activation was FeN3, and NH3 was formed by gradual hydrogenation via an associative mechanism. Interestingly, the real-time detection of N2H2+ and N2H3+ intermediate species by time-of-flight mass spectrometer (TOF-MS) further experimentally verified the synthesis of NH3 by associative mechanism at the single atomic active site (Fig. 3i). Azofra et al. fabricated single Mo active sites confined in Si-based materials and applied it in the synthesis of NH3.72 At 400 °C and atmospheric pressure, the NH3 synthesis rate reached 1.3 mmol gMo−1 h−1. Theoretical calculations indicated that N2 spontaneously adsorbed and was activated on the [(
Si–O–)MoH3] site via an associative mechanism, where the rate-determining step is the further hydrogenation of the adsorbed *NHNH2 to form NH3.
In summary, SACs possess a unique geometric and electronic structure as well as complete metal dispersion compared with nanoparticle catalysts. The single-atom sites over SACs cannot realize the direct dissociation of N2 while enabling N2 activation via an associative route for NH3 synthesis. The distinct reaction mechanism on SACs in comparison with nanoparticle catalysts is conducive to realizing higher NH3 synthesis rate at mild conditions. Nevertheless, the disadvantages of SACs for NH3 synthesis are also obvious. On the one hand, due to the high surface free energy, the single atoms are easy to migrate and agglomerate. Moreover, the metal loading of SACs is generally low, which limits the catalytic activity of NH3 synthesis. On the other hand, it is difficult for a single-atom active site to activate both N2 and H2 molecules at the same time, which may result in hydrogen poisoning phenomena, such as the observation of negative H2 reaction order in Ru1/CeO2.73 Therefore, developing high-loading SACs or combining single atoms with other active centers are effective strategies to further improve the NH3 synthesis performance.
3. SCCs for NH3 synthesis
Unlike SACs where single atoms are highly dispersed on the support in isolation, a number of atoms assembled in the form of individual clusters can form single-cluster catalysts, which results in unique geometric and electronic structure. The cluster catalysts can not only provide multiple metal atoms as catalytic sites but also possess high metal dispersion. The single-cluster catalyst can be simply classified as noble metal SCCs and non-noble metal SCCs.
3.1 Noble metal SCCs
It is well known that NH3 synthesis is a size-sensitive reaction, where the size of Ru has a significant impact on the activity of NH3 synthesis. According to DFT calculation based on the model of Wulff construction, when the particle size was between 1.8 and 2.5 nm, the number of Ru B5 sites was enriched, which was conducive to the adsorption and dissociation of N2.33 Jacobsen et al. also indicated that the optimal Ru particle size was approximately 2 nm, which can maximize the proportion of B5 sites to improve NH3 synthesis performance. When the size of Ru particle was less than 2 nm, the proportion of corner sites increased along with the decreased in the number of B5 sites. Therefore, previous research predicted that the small Ru clusters in the absence of B5 sites were supposed to be inactive in NH3 synthesis.74
Nevertheless, recent studies have shown that the activity of NH3 synthesis increases with the decrease in Ru particle size. For instance, Zhou et al. synthesized a series of Ru/BaCeO3 catalysts with various Ru particle sizes (1.1–3.0 nm) using size-controlled Ru colloids as precursors.75 The experimental results showed that the NH3 synthesis rate significantly increased as the Ru particle size decreased from 3.0 nm to 1.1 nm. At 400 °C and 1 MPa, the TOFRu total of Ru1.1/BaCeO3 can reach up to 0.124 s−1, which was about 6-fold higher that of Ru3.0/BaCeO3 (Fig. 4a). It was revealed that the small Ru clusters were conducive to the formation of Ce3+ and Ov species in BaCeO3, which promoted the electron transfer from Ru metal to N2, thereby accelerating the dissociation of N2. In addition, the small Ru clusters enhanced hydrogen spillover from Ru to BaCeO3 support to alleviate hydrogen poisoning, resulting in efficient NH3 synthesis. Similarly, Peng et al. used N-doped carbon as a carrier and prepared a series of Ru catalysts with sizes ranging from 1.4 to 5.0 nm using colloidal Ru deposition method.19 The study found that the TOF of 5 nm Ru NPs was 2.53 × 10−2 s−1. When the particle size decreased from 5.0 nm to 1.4 nm, the TOF increased to 5.23 × 10−2 s−1, mainly due to the increase in the corner sites of the catalyst (Fig. 4b), which was beneficial for reducing the work function and promoting N2 activation. These results unravel the size-dependent effect of Ru-based catalysts and highlight the superior activity of Ru cluster catalysts in NH3 synthesis.
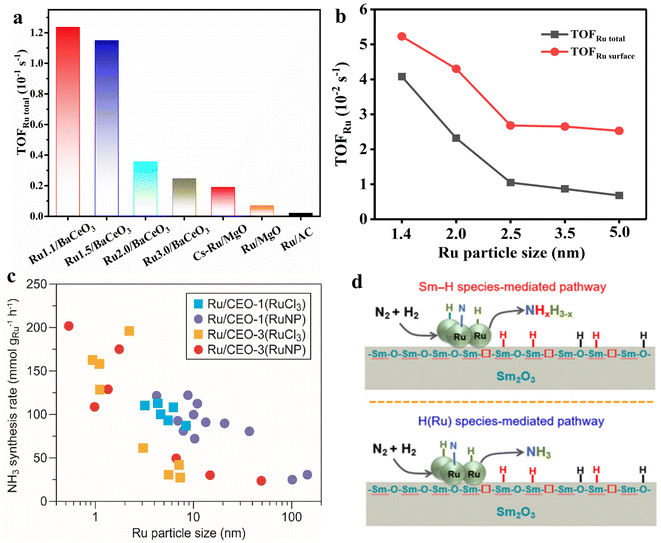 |
| Fig. 4 (a) TOFRu total of the RuxBeCeO3 catalysts at 400 °C and 1 MPa.75 (b) TOFRu of Ru NCs at 400 °C and 1 MPa.19 (c) NH3 synthesis rate of Ru/CEO-1 and Ru/CEO-3 catalysts at 400 °C and 0.1 MPa as a function of the mean Ru particle size.80 (d) Schematic illustration of chemisorbed H(Ru) species-mediated and Sm–H species-mediated reaction pathways on the Ru/Sm2O3 catalyst. Color code for different kinds of H species: H(Ru), Sm–H and O–H species are shown in blue, red and black, respectively.81,82 | |
In recent years, an increasing number of Ru cluster catalysts have been reported to exhibit high NH3 synthesis performance. Li et al. found that the activity of Ru/MgO-MIL with small Ru clusters (1.0 nm) in NH3 synthesis was approximately 19 times that of traditional 2–4 nm Ru/MgO catalyst and 7.7 times that of Ru@MIL-101.76 The superior mass activity was largely due to the efficient utilization of Ru atomic clusters. Meanwhile, the authors believed that the theoretical model of Ru particles was based on the Wulff construction, while under realistic conditions, small clusters might deviate from the ideal thermodynamic model and generate B5 sites on the surface.77,78 Feng et al. synthesized CeO2 nanorods-supported subnano Ru clusters for NH3 synthesis at mild conditions.79 The Ru clusters/CeO2 exhibited a much higher activity in NH3 synthesis than the Ru nanoparticle counterpart. It was revealed that the sub-nanometer Ru clusters species facilitated the activation and dissociation of H2 and N2 molecules, mainly responsible for its intrinsic activity in NH3 synthesis. Similarly, Hirabayashi et al. also found that the Ru small clusters over Ru/CeO2 exhibited a superior NH3 synthesis performance (Fig. 4c).80 Furthermore, the Sm2O3-supported sub-nanometer Ru cluster catalyst was developed and exhibited superior NH3 synthesis performance. Mechanistic studies and theoretical calculations showed that for the activated Ru/Sm2O3, a large amount of surface Sm–H species could be generated on Sm2O3 (Fig. 4d). The Sm–H species not only can cooperate with Ru clusters to reduce the energy barrier of nitrogen dissociation but also can directly participate in the formation of NH3 on Ru clusters.81,82
Theoretical calculations in recent years also support the idea that cluster catalysts promote NH3 synthesis more effectively. Taking the Ru3 clusters as an example, Wang et al. investigated the Ru3 single clusters anchored on the defective g-C3N4 nanosheet (Ru3/Nv-g-C3N4) for NH3 synthesis based on DFT calculations and microscopic kinetic simulations.83 Under the industrial reaction conditions of NH3 synthesis at 673 K and 100 bar, the TOF of Ru3/Nv-g-C3N4 is 5.8 times that of the Ru (0001) step surface. DFT calculations revealed that the reaction proceeded parallelly on Ru3/Nv-g-C3N4 through both dissociative and alternative associative mechanisms. With increasing temperatures or decreasing pressures, the dissociative mechanism gradually prevailed and the associative mechanism receded (Fig. 5a and b). Furthermore, Cheng used Ru19 clusters as an example to calculate the free energy distribution of a typical catalytic reaction84 based on ab initio molecular dynamics (AIMD) and free energy methods. It was found that the reaction free energies (DrG) and barrier (DGa) of the Ru19 clusters were quite different from those obtained from static geometric optimization methods, indicating that the dynamic fluctuations of clusters configurations significantly affected the reaction free energy and potential barrier. Notably, the phase transition of sub-nanometer clusters can accelerate the N2 activation due to the reduction of reaction free energy and enabling NH3 synthesis at low temperatures.
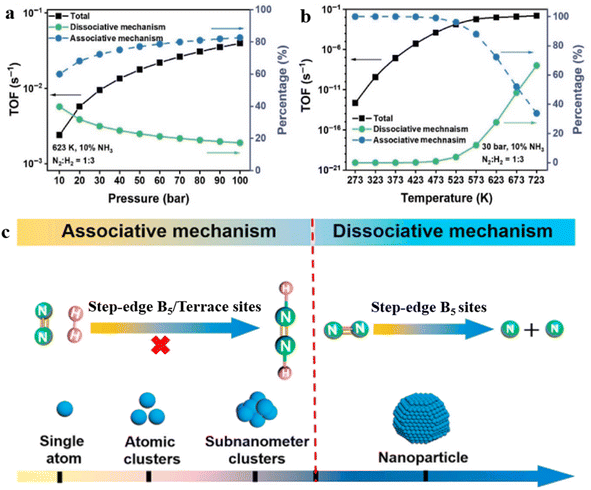 |
| Fig. 5 TOFs and contributions from dissociative and associative mechanisms as functions of (a) pressure under constant temperature of 623 K, (b) temperature under constant pressure of 30 bar.83 (c) NH3 synthesis pathway over Ru catalysts with different sizes.49 | |
Due to the absence of Ru B5 sites over Ru clusters, the reaction mechanism over Ru clusters may be different from Ru nanoparticle catalysts. Zhou et al. reported the design of sub-nanocluster Ru clusters (0.8 nm) anchored on hollow N-doped carbon spheres (Ru-SNCs) catalysts.85 Under 400 °C and 3 MPa conditions, the TOFRu of 0.5Ru-SNC (0.067 s−1) was 3.5 times higher than that of the 1.2Ru-NPs catalyst (0.019 s−1). The UV-vis and in situ infrared experiments showed that the N2H4 species was the main intermediate for NH3 synthesis on the Ru-SNCs catalyst. It demonstrated that the Ru–SNCs catalyst can follow an associative route for N2 activation, which circumvented the direct dissociation of N2 and resulted in highly efficient NH3 synthesis at mild conditions. Furthermore, Li et al. synthesized a series of Ru catalysts with Ru sizes ranging from single atoms, atomic clusters, sub-nanometric clusters, to nanoparticles.49 Researches showed that with the size decrease of Ru nanoparticle to sub-nanometric and atomic level, the activation pathway of N2 changed from dissociative mechanism to associative mechanism (Fig. 5c). At the sub-nanometric level, the enhanced intra-cluster interaction of atomic clusters up-shifted the Ru d-band center toward the Fermi level, thus significantly promoting N2 activation via the associative mechanism with extremely small reaction energy barriers. In addition, the synergistic effect of single atom and cluster for NH3 synthesis was investigated. Sivan et al. synthesized a series of Ru single atoms, clusters, and nanoparticles using a one-pot method to study the effect of Ru size on NH3 synthesis.86 The order of TOF was as follows: 2.8Ru–CeO2 (Ru single atom and cluster) > 1.4Ru–CeO2 (Ru single atom) > 4.0Ru–CeO2 (Ru nanoparticle) > 5.3RuCeO2 (Ru nanoparticle). It was suggested that the 2.8Ru–CeO2 catalyst, which contained both Ru single atoms and clusters, can trigger NH3 synthesis through the integration of dissociative and associative routes, resulting in excellent catalytic activity and stability. This work indicates that the combination of Ru single atom and cluster is a promising strategy to improve NH3 synthesis performance.
In summary, recent researches have shown that the Ru cluster catalysts usually exhibit higher NH3 synthesis rates than Ru nanoparticle catalysts. The potential reasons are summarized as follow: (1) the Ru cluster catalysts possess a higher metal dispersion and utilization than Ru nanoparticle catalysts; (2) the strong intra-cluster interaction of the Ru cluster can enhance the adsorption and activation of N2 molecules; (3) the distinct reaction mechanism over Ru cluster catalysts compared with Ru nanoparticle catalysts enables facile NH3 synthesis under mild conditions. Nevertheless, the associative mechanism over Ru cluster catalysts is still controversial, which still require solid evidence to support this opinion. Therefore, there is an urgent need to develop more advanced characterization techniques such as time-of-flight secondary ion mass spectrometry (TOF-SIMS) and neutron technology to detect intermediate species and elucidate the reaction mechanism.
3.2 Non-noble metal SCCs
Theoretical calculations indicate that an ideal metal catalyst for NH3 synthesis should have moderate adsorption energy of N atoms and NHx desorption energy, namely, near the top position of the volcano diagram, such as the Ru metal.87,88 In consideration of the scarcity and high price of Ru metal, there is an urgent need to develop catalysts with excellent catalytic performance, cost-effective or rich in Earth for NH3 synthesis at mild conditions.89 Liu et al. proposed an active center of Fe3 clusters anchored on the Al2O3 (010) surface through first-principles calculations and microkinetic analysis (Fig. 6a).47 The study found that the first hydrogenation of N2 to generate NNH on the Fe3/θ-Al2O3 (010) surface was much faster than the dissociative mechanism. The subsequent NNH dissociation also had a low energy barrier of 0.45 eV, thus bypassing the BEP relationship and the limitation on one the side of the volcano curve (Fig. 6b). Correspondingly, the calculated TOF of NH3 synthesis on Fe3/θ-Al2O3 (010) was comparable to that of Ru B5 sites and was two orders of magnitude faster than the C7 sites of Fe. These results indicated that surface-anchored metal trimers and/or multinuclear clusters might serve as efficient catalysts for NH3 synthesis at mild conditions. Meanwhile, Luo et al. conducted a systematic study of NH3 synthesis on triatomic metal clusters (M3) of 20 transition metals.90 The three key processes including N2 dissociation, hydrogenation, and NH3 desorption were calculated to evaluate the catalytic roles of these M3 clusters. The results showed that the transition metals can be divided into three categories (Fig. 6c): (1) N2 can spontaneously dissociate on TMI-M3 metals (i.e., Sc3, Ti3, V3, Y3, Zr3, and Nb3); (2) obvious activation barriers needed to be overcome on TMII-M3 metal clusters (i.e., Cr3, Mn3, Fe3, Co3, Mo3, Tc3, Ru3, and Rh3); (3) and the activation process on TMIII-M3 clusters (i.e., Ni3, Cu3, Zn3, Pd3, Ag3, and Cd3) was not feasible. Considering the three key processes for N2 reduction to NH3, four M3 clusters in the TMI group (Y3, Sc3, Zr3, and Nb3) were proposed as ideal candidate clusters catalyst for NH3 synthesis.
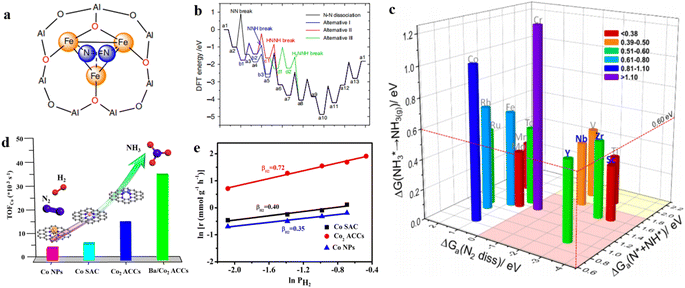 |
| Fig. 6 (a) Schematic representation of N2 coordinated with heterogeneous Fe3/θ-Al2O3(010) in the same configuration; (b) the dissociative mechanism, and three pathways of associative mechanism with N–N bond dissociation at *NNH, *HNNH, and *HNNH2 intermediates by the alternating hydrogenation route.47 (c) Summary of the free energy of transition state for N2 dissociation, hydrogenation barrier of *N + *NH formation, and desorption energy of NH3 on 14 three-atom metal clusters.90 (d) Turnover frequencies (TOFCo total) at 400 °C and 1 MPa. (e) Reaction orders of H2 over different catalysts at 400 °C and 1 MPa.20 | |
Beyond theoretical calculations, Peng et al. conducted research on the synthesis of NH3 using non-precious metal cluster catalysts.20 The cobalt atomic dimers on a nitrogen–carbon support (Co2 ACCs) were synthesized for NH3 synthesis and using the Co SAC and Ru NPS as references. Under 400 °C and 1 MPa, Co2 ACCs exhibited a very high NH3 synthesis rate of 8.54 mmolNH3 gcat−1 h−1, which is ∼1.8- and 3.3-fold that of the Co SAC (4.60 mmolNH3 gcat−1 h−1) and Co NPs (2.55 mmolNH3 gcat−1 h−1), respectively. The low N2 reaction order and the bands associated with N2Hx intermediates in the in situ diffuse reflectance infrared Fourier-transform spectroscopy (DRIFTS) suggested that N2 was not directly dissociated while it underwent the associative route for NH3 synthesis (Fig. 6d and e).
Although there is currently limited research on non-precious metal cluster catalysts in NH3 synthesis, theoretical studies indicate that non-precious metal cluster catalysts exhibit significant potential in this regard. The key challenge is to prepare uniform non-precious metal cluster catalysts with a specific number of atoms. Current methods for preparing atomic clusters include (1) using precursors containing specific atomic clusters; (2) anchoring atomic clusters on carrier materials with defect sites, such as C3N4 carriers; and (3) the application of atomic layer deposition (ALD). Besides, developing more efficient methods for preparing atomic cluster catalysts is of great significance.
4. BCCs for NH3 synthesis
Apart from single-atom and single-cluster catalysts, bimetallic clusters have emerged as one of the important categories of heterogeneous catalyst. In many cases, bimetallic catalysts exhibit higher catalytic activity or selectivity than their monometallic counterpart due to the strong synergistic effect between different sites.91,92 In NH3 synthesis, it was reported that the dual-site strategy can effectively improve NH3 synthesis performance owing to the separated sites for N2 and H2 activation, which can not only avoid the hydrogen poisonous over active sites caused by competition adsorption of N2 and H2, but also provide the possibility to circumvent the scale relations in NH3 synthesis.93,94 In addition, the bimetallic-cluster catalysts exhibit quite distinct properties in electronic and geometric structure compared with monometallic catalysts, which has a great impact on the reaction mechanism in NH3 synthesis.95 In general, according to the composition and structure of bimetallic catalysts, it can be classified into bimetallic-alloy catalysts, bimetallic single-cluster catalysts, and single-atom/dimer alloy catalysts.
4.1 Bimetallic-alloy catalysts
Because of high surface free energy, the Ru clusters are thermodynamically unstable and tend to sinter at high temperatures during NH3 synthesis. In order to increase the stability of Ru clusters, Ni et al. reported a method to confine CoxRuy nanoparticles in the pores of N-doped carbon through the guiding of benzoic acid to restrict the deposition location of Ru and Co species.96 The results of high-angle annular dark field scanning transmission electron microscopy (HAADF-STEM) showed that the CoxRuy alloy with an average size of 2.2–2.6 nm was confined inside the pores of N-doped carbon spheres. The catalytic activity test displayed that Co1Ru2/NC had a high NH3 synthesis rate of 18.9 mmolNH3 gcat−1 h−1 at 400 °C and 3 MPa, which is 3.2-fold that of the Ru/NC catalyst (Fig. 7a). In addition, Co1Ru2/NC presented a relatively higher stability in NH3 synthesis than Co1Ru2/NC-IWI prepared by traditional incipient wetness impregnation method (Fig. 7b), demonstrating that the confinement of Ru and Co NPs inside the hollow pores of N-doped carbon spheres can enhance the stability of RuCo alloy. In comparison with Ru–Co nanoparticles, Yang et al. developed a bimetallic Ru–Co clusters/N–C catalyst derived from a confined alloying process within zeolite–imidazolate frameworks (ZIF).97 As shown in Fig. 7c, Ru3(CO)12 was introduced into the cavity of ZIFs that use the Co-bearing metal–organic frameworks as the host. After decomposition at 900 °C, the bimetallic Ru–Co clusters can be in situ formed. Fig. 7d showed that Ru–Co clusters@N–C possessed the highest NH3 synthesis rate among Ru–Co NPs@N–C, Ru clusters@N–C, and Ru NPs@N–C catalysts. Compared with the Ru–Co NPs catalyst, the bimetallic Ru–Co clusters exhibited a higher density of low-coordination and unsaturated active sites, which may favor the adsorption and activation of N2 molecules. Besides, alloying with Co atoms would tailor the local structure of Ru clusters owing to the synergistic effect. As a result, the NH3 synthesis rate over Ru–Co clusters@N–C is 2.4 times higher than that of Ru clusters@N–C. These results elucidate that bimetallic Ru–Co alloy can not only improve NH3 synthesis rate but also increase the thermal stability compared with Ru nanoparticle catalysts.
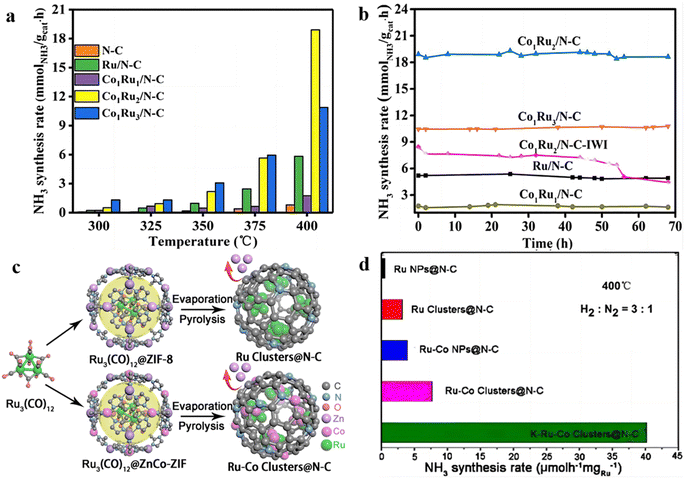 |
| Fig. 7 (a) NH3 synthesis rate over catalysts at 300–400 °C and 3 MPa. (b) Time dependence of NH3 synthesis rate at 400 °C and 3 MPa.96 (c) Schematic diagram of the preparation of Ru–Co clusters@N–C catalyst. (d) NH3 synthesis rate over the as-prepared catalysts at 400 °C and 0.1 MPa.97 | |
4.2 Bimetallic single-cluster catalysts
Bimetallic single-cluster catalysts mean that one of the elements in bimetallic clusters is atomically dispersed.98,99 The bimetallic single-cluster sites (M1An) not merely possess the advantage of SACs with complete metal dispersion but also provide the possibility to overcome scaling relation through the cooperative effects of bimetallic sites that are responsible for different elementary steps. In NH3 synthesis, Ma et al. explored the reaction mechanism of N2-to-NH3 thermal conversion on the bimetallic Rh1Co3 site using DFT calculation.100 They found that the preferred reaction pathway over the Rh1Co3 site was the associative mechanism analogous to the biological process, which was driven by both the charge buffering ability of doped metal Rh in Rh1Co3 and the complementary role of Co in catalysis (Fig. 8a). Apart from the bimetallic single-cluster anchored on a metal oxide, the supported bimetallic single-cluster catalysts are also promising for NH3 synthesis. Ru-loaded hydrides were reported to work as efficient catalysts for NH3 synthesis at low temperatures, where the formation of hydrogen vacancies (VH) at the Ru/hydrides is a key factor for NH3 synthesis. Nakao et al. investigated the VH formation and H-migration behavior at the Ru-TM/Ca2NH interface using the Ru5TM/Ca2NH model by DFT calculations (Fig. 8b).101 It was revealed that the five late TMs (Fe, Co, Rh, Os, and Ir) and eight early TMs (Sc, Ti, Y, Zr, Nb, La, Hf, and Ta) were determined to promote VH formation. In addition, Nb, Hf, Ta, Os, and Ir can also decrease the H-migration energy at the Ru5TM/Ca2NH interface when compared with that at the Ru6/Ca2NH interface. Therefore, the formation of bimetallic Ru5TM clusters over hydrides via the addition of a proper TM atom can improve the NH3 synthesis performance in terms of the VH formation and H-migration energy. To unveil the synergy of bimetallic sites, Cheng et al. studied the Fe13 cluster and bimetallic Fe12X (X = V, Cr, Mn, Co, Ni, Cu, Zn, Nb, Mo, Ru, and Rh) clusters for NH3 synthesis (Fig. 8c).102 The energies analysis showed that center substitution (X@Fe12) was favored while doping single V, Cr, Co, and Mo atoms, whereas Mn, Ni, Cu, Zn, Nb, Ru, and Rh tended to form shell-doped structures (Fe12X). Among these bimetallic single clusters, Fe12Nb exhibited the lowest activation energy for N2 dissociation and the dissociated *N species was converted into NH3 efficiently via a hydrogenation process (Fig. 8d).
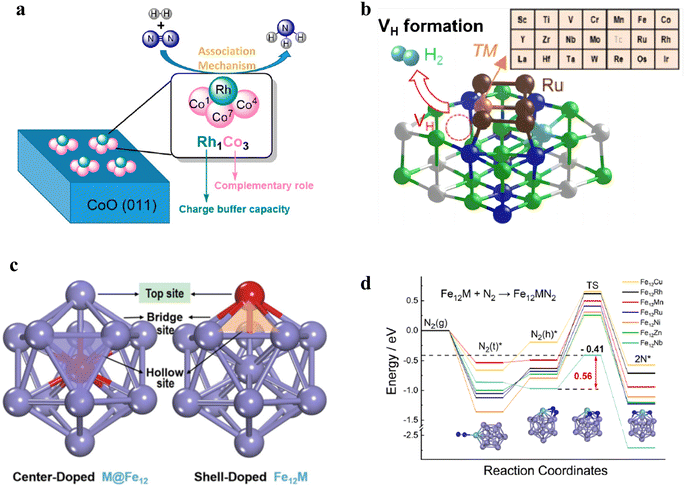 |
| Fig. 8 (a) Schematic diagram of Rh1Co3 bimetallic sites for NH3 synthesis.100 (b) Schematic diagram of the Ru5TM/Ca2NH model.101 (c) The chemical structure of heteroatom-doped Fe13 clusters. The red atoms represent the different doping positions.102 (d) Pathways of N2 adsorption and dissociation on the shell-doped Fe12TM clusters. | |
To be noted, although bimetallic single-cluster catalysts show great advantages for NH3 synthesis on the basis of DFT calculations, the synthesis and application of bimetallic single-cluster catalysts in NH3 synthesis have not been reported yet. The main challenge lies in the preparation of uniform bimetallic clusters with specific structure, which requires the development of special preparation method and the application of advanced equipment. Besides, DFT calculations demonstrated that the reaction mechanism of NH3 synthesis over bimetallic single-cluster catalysts is closely related to their composition and property. For instance, the preferred reaction pathway over the Rh1Co3 site is an associative mechanism, while that over the Fe12X (X = Mn, Ni, Cu, Zn, Nb, Ru, and Rh) site is dissociative mechanism. The real reaction mechanisms over different bimetallic single-cluster catalysts still need to be further confirmed by experimental research.
4.3 Single-atom/dimer alloy catalysts
Single-atom alloys (SAAs) are a class of bimetallic single-site heterogeneous catalyst in which small amounts of one metal are atomically dispersed in the surface of a different metal.103,104 Distinguished from traditional metal alloy, the amount of guest metal atom in SAAs is small and the sites are isolated. Due to the unique geometry of SAAs, the location of the transition state and the binding site of reaction intermediates are often decoupled, which make it possible to enable both the facile dissociation of reactants and weak binding of the intermediates. For example, Zhang at el. reported a Co1Ru SAA catalyst via the deposition of atomically dispersed Co onto the surface of Ru tiny subnanoclusters (TCs).105 It was revealed that the special structure can generate a spatial effect and induce strong interelectronic interactions between Ru and Co, which can lead to the simultaneous generation of the high-surface-unoccupied Co 3d charge and obvious upshifting of the Ru d-band center. Based on the special electronic and geometric structure, Co1Ru TCs can promote N2 activation with a low barrier energy and enable the repulsion to the adsorption of the N-containing intermediates on the catalyst surface, resulting in the weakening of the binding of *NH3 and *N2H4 intermediates on the Co1Ru TCs catalyst surface. In such a case, the scaling relation over Co1Ru TCs in NH3 synthesis was decoupled. As such, the developed Co1Ru TCs presented a much higher NH3 synthesis rate and lower reaction activation energy than those of Co1Ru dual atoms (DAs) and Co1Ru nanoparticles (NPs). Furthermore, Zhang et al. prepared a series of M1Ru SAA catalysts by the anchoring of transition-metal single atom (Fe, Co, and Ni) onto Ru nanoclusters.106 They found that Co1Ru SAA has the highest NH3 synthesis rate and the largest TOFRu value among various M1Ru SAA (Fig. 9a). Compared with the CoRu nanoparticle alloy (NPA), various characterizations elucidated that Co1Ru SAA possessed stronger electronic interaction between Co and Ru, which induced lower work function and up-shift d band center toward the Fermi level, thus accelerating the activation of N2. Meanwhile, the unique SAA structure could effectively tune the N2 activation pathway. DRIFTS technique showed that the *N2Dx intermediates can be observed over Co1Ru SAA under the atmosphere of 25% N2–75% D2, demonstrating that N2 molecules can be activated via an associative mechanism, accounting for efficient NH3 synthesis at mild conditions (Fig. 9b).
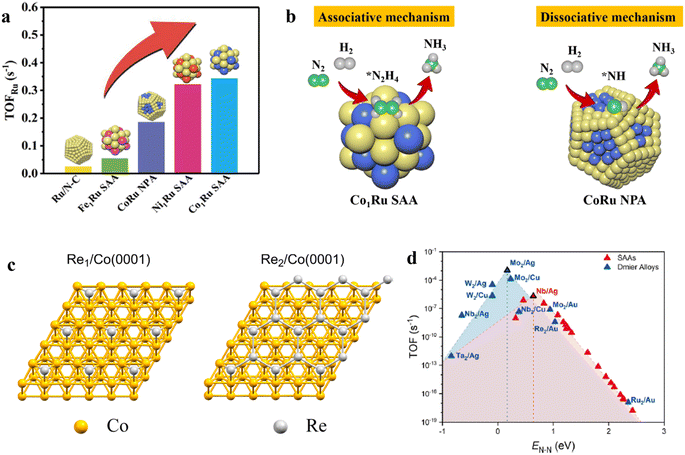 |
| Fig. 9 (a) TOFRu values over the as-synthesized catalysts at 400 °C and 1 MPa. (b) The proposed N2 activation pathway over Co1Ru SAA and CoRu NPA.106 (c) Top views of free Re1 and Re2 centers over Co(0001).107 (d) Comparison of the volcano plot of TOF for dimer alloys and SAAs as a function of *N–N transition state energies.107 The TOF is calculated at 673 K, PN2 = 24.5 bar, PH2 = 74.25 bar, PNH3 = 1 bar, and 2% conversion to NH3. TOFs of Mo2/Au, Ru2/Au, and Re2/Au dimer alloys are calculated using the DFT results reported in ref. 109. | |
In addition, Cholach et al. investigated the active centers of one or a pair of Re atoms on the Co(0001) support for NH3 synthesis using DFT calculation (Fig. 9c).107 They found that the binding N to Re and Co can significantly reduce the heat of dissociative adsorption of N2 in the cases of Re1/Co(0001), Re2/Co(0001), and Re(0001). As a result, the specific TOF on the basis of Re1/Co(0001), Re2/Co(0001), and the plane Re(0001) changed in the order of 8.0 × 103, 32.0, and 1.0, respectively. They also demonstrated that the Re single atom on the Co(0001) support exhibited a much higher NH3 synthesis performance than that of Re dual atoms. Nevertheless, when the host metal was changed into either Cu(111) or Ag(111), the transition-metal single atom or dimer atoms supported on Ag(111) or Cu(111) catalysts presented different trends. Zhang et al. revealed that the Nb/Ag SAA was located at the volcano peak of NH3 synthesis on the basis of the TM1/Ag(111) system, while the Mo2/Ag dimer alloy exhibited a much higher TOF value compared with Nb/Ag SAA (Fig. 9d).108 DFT calculations showed that the Mo2/Ag dimer alloy has a lower N2 activation energy and weaker N absorption than that of Nb/Ag SAA, i.e., the BEP relationship for N2 dissociation derived on dimer alloys is closer to the ideal limit in comparison to that obtained on SAAs, leading to the higher activities of dimer alloys for NH3 synthesis.
Although M1Ru SAAs have been reported to exhibit considerable NH3 synthesis performance experimentally, while this kind of SAAs are based on the Ru as the host metal atoms in SAAs. The development of SAAs with single-atom Ru as the guest metal atom in SAAs for NH3 synthesis is more appealing, especially for reducing the content of noble Ru metal. In addition, the predicted higher NH3 synthesis performance of dimer alloys than SAAs provides a wide platform to exploit more efficient catalysts for NH3 synthesis. Developing advanced methods for the preparation of dimer alloys is really worth exploring.
5. Conclusions and prospects
The development of efficient catalysts for NH3 synthesis under mild conditions has been a long-term endeavor in this field. The remarkable differences in the geometric and electronic structure of active sites at different scales stimulate that the single-atom and cluster catalysts exhibit significantly distinct catalytic performance and reaction mechanism compared with nanoparticle catalysts. Based on the analysis in this review, the main differences between nanoparticle catalysts, single-atom and cluster catalysts are summarized by the following three aspects:
5.1 Hydrogen poisonous
Nanoparticle catalysts usually suffer from unavoidable hydrogen poisoning due to the strong adsorption of H species on the nanoparticle surface, while the hydrogen poisonous was largely alleviated or eliminated on cluster catalysts.110–112 The probable reasons may be attributed to that the emergence of discrete d and sp bands of cluster atoms can alter the adsorption strength of N2 and H2 molecules, avoiding hydrogen poisonous or the increased interface of the metal–support can promote the hydrogen spillover to support and thus eliminate hydrogen poisonous.49,75
5.2 Reaction mechanism
The dissociative mechanism is preferred to occur on nanoparticle catalysts because of the presence of multiple atomic sites, such as Ru B5 sites or Fe C7 sites, which are highly active for the dissociation of N2 molecules.31,33 In terms of single-atom and cluster catalysts, the associative mechanism is suggested to be dominant even though satisfactory evidence is still needed to support this opinion.
5.3 Catalytic performance
By comparing the performance of different-size catalysts (Table 1), the cluster catalysts exhibit higher NH3 synthesis rates than nanoparticle catalysts and single-atom catalysts thanks to the exposure of more active sites and/or different reaction mechanisms.49,113 Concerning single-atom catalysts, the NH3 synthesis rates are generally low in spite of their high dispersion.113,114 The underlying reason could be the difficulty of simultaneous activation of N2 and H2 reactants on a single-atom site. To be noted, Ru species exist as large nanoparticles (≥2 nm) in industrial Ru-based catalysts. The higher catalytic performance of Ru clusters than Ru particles makes it possible to reduce the Ru content of commercial catalysts in the form of stable Ru clusters.
Table 1 Catalytic properties for NH3 synthesis over various SACs, SCCs, and BCCs catalysts
Catalyst |
Metal content (wt%) |
Reaction conditions |
NH3 synthesis rate (mmolNH3 gcat−1 h−1) |
Ref. |
Temperature (°C) |
Pressure (MPa) |
WHSV (mL g−1 h−1) |
SACs |
Co–N–C |
3.8 |
350 |
1.0 |
60 000 |
4.34 |
48
|
Ba–Ru SAs/S-1 |
0.27 |
400 |
0.1 |
18 000 |
1.39 |
63
|
Ru/HZ SAC |
0.2 |
300 |
1.0 |
60 000 |
2.52 |
65
|
MoHx |
2.0 |
400 |
0.1 |
12 000 |
0.026 |
72
|
Co–N2 |
3.2 |
300 |
1.0 |
60 000 |
2.7 |
69
|
FeN4©G-K |
2.1 |
190 |
0.1 |
30 000 |
10.3 × 10−3 |
71
|
Ru/CeO2(SAC) |
1.00 |
450 |
1.0 |
72 000 |
9.9 |
119
|
Ru SAC |
0.39 |
400 |
1.0 |
60 000 |
4.7 |
49
|
SCCs |
1.4 nm Ru NCs |
1.18 |
400 |
1.0 |
60 000 |
17.1 |
19
|
2.0 nm Ru NCs |
1.06 |
400 |
1.0 |
60 000 |
8.8 |
19
|
Ru3.0/BaCeO3 |
0.44 |
400 |
1.0 |
60 000 |
3.4 |
75
|
Ru1.1/BaCeO3 |
0.46 |
400 |
1.0 |
60 000 |
19.4 |
75
|
Ru/MgO-MIL |
3.00 |
400 |
1.0 |
15 000 |
4.5 |
76
|
Ru clusters/CeO2 |
5.00 |
400 |
1.0 |
— |
28.0 |
79
|
0.5Ru-SNCs |
0.49 |
400 |
3.0 |
60 000 |
11.7 |
85
|
Ru ACCs |
0.40 |
400 |
1.0 |
60 000 |
7.4 |
49
|
Ru-2.8 nm |
0.44 |
400 |
1.0 |
60 000 |
2.9 |
49
|
5% Ru/Sm2O3 (activated) |
5.00 |
400 |
1.0 |
24 000 |
32.2 |
82
|
Ru/Sm2O3 |
5.00 |
400 |
1.0 |
24 000 |
23.0 |
81
|
Co2 ACCs |
0.90 |
400 |
1.0 |
60 000 |
8.5 |
20
|
Ba/Co2 ACCs |
0.90 |
400 |
1.0 |
60 000 |
19.4 |
20
|
BCCs |
Co1Ru2/NC |
1.17Ru + 0.41Co |
400 |
3.0 |
60 000 |
18.9 |
95
|
Ru–Co clusters@N–C |
— |
400 |
1.0 |
12 000 |
0.78 |
96
|
Ru clusters@N–C |
— |
400 |
1.0 |
12 000 |
0.32 |
96
|
Co1Ru SAA |
1.05Ru + 0.30Co |
400 |
1.0 |
60 000 |
4.38 |
105
|
Fe1Ru SAA |
1.36Ru + 0.47Fe |
400 |
1.0 |
60 000 |
0.70 |
105
|
Ni1Ru SAA |
1.07Ru + 0.51Ni |
400 |
1.0 |
60 000 |
2.90 |
105
|
CoRu NPA |
0.95Ru + 0.29Co |
400 |
1.0 |
60 000 |
2.09 |
105
|
It is worth noting that the exploitation of single-atom and cluster catalysts for NH3 synthesis with high-efficiency has gained more and more attention. With the advancement of preparation method and characterization techniques, an increasing number of single-atom and cluster catalysts would be developed for NH3 synthesis. In the future, some research fields in terms of single-atom and cluster catalysts are promising to further improve the catalyst performance and deepen the fundamental understanding of structure–activity relationship as well as the reaction mechanism for NH3 synthesis.
Fabricating the uniform active sites in SCCs or BCCs is still a great challenge. In terms of performance for NH3 synthesis, SCCs or BCCs are superior to SACs and nanoparticle catalysts. Nevertheless, the preparation of uniform and exclusive structural SCCs or BCCs is much more difficult than SACs and nanoparticle catalysts, and most researches on SCCs or BCCs were based on DFT calculations. Currently, the widely used synthesis methods for SCCs or BCCs are the control of low metal loading or high-temperature pyrolysis, which are the lack of the precise manipulation of the location of metal species. With the usage of organometallic complex precursors containing atomic clusters and the application of advanced methods and instruments, it provides the possibility to precisely control the configuration and location of atomic clusters. Meanwhile, the precise construction of cluster sites can not only maximize metal utilization but also provide platforms to investigate the reaction mechanism.
To better characterize the structure of single-atom and cluster catalysts as well as to detect the reaction intermediate species, it is necessary to develop advanced characterization techniques. Nowadays, the reaction mechanisms via the associative or dissociative pathway of single-atom and cluster catalysts are still under debate. The intermediates for NH3 synthesis, such as NHx and N2Hx species, are mainly detected by the UV-vis spectrum, in situ DRIFTS, and NEXAFS spectra.85,115,116 The main reason is due to that these techniques would be possible to detect only those long-lived intermediates, which can give rise to a detectable population under steady-state conditions. Meanwhile, it is also difficult to accurately distinguish the spatial distribution of intermediates over different active sites based on in situ DRIFTS and NEXAFS spectra. The development of advanced characterization techniques, such as near ambient pressure X-ray photoelectron spectroscopy (NAP-XPS), TOF-SIMS, and neutron scattering techniques, would shed light on the analysis of catalyst structure and reaction mechanism in NH3 synthesis.
The industrial application of NH3 synthesis via an associative mechanism still faces long-term challenges, mainly focusing on the accessibility and durability of catalysts. Specifically, the application of catalysts with highly dispersed active sites is trapped in the metal agglomeration, i.e., the growth of metal size from subnanometer to nanometer in the long-period operation. As a result, the NH3 synthesis mechanism is changed from associative route to dissociative route during the reaction process, possibly accompanied by the decrease in the NH3 synthesis rate. Moreover, the large-scale preparation of single-atom and cluster catalysts in the industry is still tough because of the complicated synthetic method and the difficulty of controlling the homogeneous dispersion of low-loading metal. Therefore, more efforts are needed to solve the stability and large-scale preparation of advanced single-atom and cluster catalysts before industrial application.
Artificial intelligence (Ai)-assisted catalyst design will accelerate the development of efficient single-atom and cluster catalysts for NH3 synthesis.117,118 Traditional catalysts were discovered through trial-and-error method coupled with chemical intuition. In the early days of NH3 synthesis research, thousands of catalysts were prepared and tested to find a catalyst suitable for industrialization. Nowadays, we are still trying new types of catalysts in order to improve the NH3 synthesis activity at lower temperatures and pressure conditions. With the assistance of artificial intelligence, especially for automatic machine-learning, it shows enormous potential to accelerate the predictive discovery of novel catalyst formulations. Meanwhile, the combination of machine-learning methodology and high-throughput experimentation can greatly shorten the period of discovery of efficient catalysts for NH3 synthesis.
Author contributions
Conceptualization, investigation and writing – original draft: X. Peng, M. Zhang and T. Zhang. Conceptualization and writing – review and editing: Y. Zhou and X. Wang. Investigation: Jun Ni. Funding acquisition and writing – review and editing: X. Wang. Funding acquisition and supervision: L. Jiang.
Conflicts of interest
The authors declare no conflict of interest.
Acknowledgements
The work was supported by the National Natural Science Foundation of China (22222801, 2221005, 22038002, 92361303, 22108037), National Key Research and Development Program (2022YFA1604101, 2021YFB4000400).
References
- Z. W. Seh, J. Kibsgaard, C. F. Dickens, I. Chorkendorff, J. K. Nørskov and T. F. Jaramillo, Combining theory and experiment in electrocatalysis: insights into materials design, Science, 2017, 355, eaad4998 CrossRef PubMed.
- K. Mazloomi and C. Gomes, Hydrogen as an energy carrier: prospects and challenges, Renewable Sustainable Energy Rev., 2012, 16, 3024–3033 CrossRef CAS.
- Y. Kojima and M. Yamaguchi, Ammonia as a hydrogen energy carrier, Int. J. Hydrogen Energy, 2022, 47, 22832–22839 CrossRef CAS.
- P. Wang, H. Xie, J. Guo, Z. Zhao, X. Kong, W. Gao, F. Chang, T. He, G. Wu and M. Chen, The formation of surface lithium–iron ternary hydride and its function on catalytic ammonia synthesis at low temperatures, Angew. Chem., Int. Ed., 2017, 129, 8842–8846 CrossRef.
- T.-N. Ye, S.-W. Park, Y. Lu, J. Li, J. Wu, M. Sasase, M. Kitano and H. Hosono, Dissociative and associative concerted mechanism for ammonia synthesis over Co-based catalyst, J. Am. Chem. Soc., 2021, 143, 12857–12866 CrossRef CAS PubMed.
- C. Zamfirescu and I. Dincer, Using ammonia as a sustainable fuel, J. Power Sources, 2008, 185, 459–465 CrossRef CAS.
- C. H. Christensen, T. Johannessen, R. Z. Sørensen and J. K. Nørskov, Towards an ammonia-mediated hydrogen economy, Catal. Today, 2006, 111, 140–144 CrossRef CAS.
- N. Kuganathan, H. Hosono, A. L. Shluger and P. V. Sushko, Enhanced N2 dissociation on Ru-loaded inorganic electride, J. Am. Chem. Soc., 2014, 136, 2216–2219 CrossRef CAS PubMed.
- H. Liu, Ammonia synthesis catalyst 100 years: practice, enlightenment and challenge, Chin. J. Catal., 2014, 35, 1619–1640 CrossRef CAS.
- K. Sato and K. Nagaoka, Boosting ammonia synthesis under mild reaction conditions by precise control of the basic oxide–Ru interface, Chem. Lett., 2021, 50, 687–696 CrossRef CAS.
- J. Wang, L. Yu, L. Hu, G. Chen, H. Xin and X. Feng, Ambient ammonia synthesis via palladium-catalyzed electrohydrogenation of dinitrogen at low overpotential, Nat. Commun., 2018, 9, 1795–1802 CrossRef PubMed.
- T. Ogawa, Y. Kobayashi, H. Mizoguchi, M. Kitano, H. Abe, T. Tada, Y. Toda, Y. Niwa and H. Hosono, High electron density on Ru in intermetallic YRu2: the application to catalyst for ammonia synthesis, J. Phys. Chem. C, 2018, 122, 10468–10475 CrossRef CAS.
- A. Vojvodic, A. J. Medford, F. Studt, F. Abild-Pedersen, T. S. Khan, T. Bligaard and J. Nørskov, Exploring the limits: a low-pressure, low-temperature Haber–Bosch process, Chem. Phys. Lett., 2014, 598, 108–112 CrossRef CAS.
- M. Kitano, Y. Inoue, Y. Yamazaki, F. Hayashi, S. Kanbara, S. Matsuishi, T. Yokoyama, S.-W. Kim, M. Hara and H. Hosono, Ammonia synthesis using a stable electride as an electron donor and reversible hydrogen store, Nat. Chem., 2012, 4, 934–940 CrossRef CAS PubMed.
- J. Qian, Q. An, A. Fortunelli, R. J. Nielsen and W. A. Goddard III, Reaction mechanism and kinetics for ammonia synthesis on the Fe (111) surface, J. Am. Chem. Soc., 2018, 140, 6288–6297 CrossRef CAS PubMed.
- J. W. Erisman, M. A. Sutton, J. Galloway, Z. Klimont and W. Winiwarter, How a century of ammonia synthesis changed the world, Nat. Geosci., 2008, 1, 636–639 CrossRef CAS.
- G. Xu, C. Cai and T. Wang, Toward Sabatier optimal for ammonia synthesis with paramagnetic phase of ferromagnetic transition metal catalysts, J. Am. Chem. Soc., 2022, 144, 23089–23095 CrossRef CAS PubMed.
- J. Wang, W. Cui, Q. Liu, Z. Xing, A. M. Asiri and X. Sun, Recent progress in cobalt-based heterogeneous catalysts for electrochemical water splitting, Adv. Mater., 2016, 28, 215–230 CrossRef CAS PubMed.
- X. Peng, X. Chen, Y. Zhou, F. Sun, T. Zhang, L. Zheng, L. Jiang and X. Wang, Size-dependent activity of supported Ru catalysts for ammonia synthesis at mild conditions, J. Catal., 2022, 408, 98–108 CrossRef CAS.
- X. Peng, H. Cai, Y. Zhou, J. Ni, X. Wang, B. Lin, J. Lin, L. Zheng, C.-t. Au and L. Jiang, Studies of a highly active cobalt atomic cluster catalyst for ammonia synthesis, ACS Sustain. Chem. Eng., 2022, 10, 1951–1960 CrossRef CAS.
- M. A. Shipman and M. D. Symes, Recent progress towards the electrosynthesis of ammonia from sustainable resources, Catal. Today, 2017, 286, 57–68 CrossRef CAS.
- G. Ertl, Reactions at surfaces: from atoms to complexity (Nobel lecture), Angew. Chem., Int. Ed., 2008, 47, 3524–3535 CrossRef CAS PubMed.
- M. J. Bezdek and P. J. Chirik, Expanding boundaries: N2 cleavage and functionalization beyond early transition metals, Angew. Chem., Int. Ed., 2016, 55, 7892–7896 CrossRef CAS PubMed.
- H.-P. Jia and E. A. Quadrelli, Mechanistic aspects of dinitrogen cleavage and hydrogenation to produce ammonia in catalysis and organometallic chemistry: relevance of metal hydride bonds and dihydrogen, Chem. Soc. Rev., 2014, 43, 547–564 RSC.
- S. Zhang, Y. Zhao, R. Shi, G. I. Waterhouse and T. Zhang, Photocatalytic ammonia synthesis: recent progress and future, EnergyChem, 2019, 1, 100013 CrossRef.
- L. Ouyang, J. Liang, Y. Luo, D. Zheng, S. Sun, Q. Liu, M. S. Hamdy, X. Sun and B. Ying, Recent advances in electrocatalytic ammonia synthesis, Chin. J. Catal., 2023, 50, 6–44 CrossRef CAS.
- J. Zhao and Z. Chen, Single Mo atom supported on defective boron nitride monolayer as an efficient electrocatalyst for nitrogen fixation: a computational study, J. Am. Chem. Soc., 2017, 139, 12480–12487 CrossRef CAS PubMed.
- P. Emmett and S. Brunauer, The adsorption of nitrogen by iron synthetic ammonia catalysts, J. Am. Chem. Soc., 1934, 56, 35–41 CrossRef CAS.
- G. Ertl, Surface science and catalysis-studies on the mechanism of ammonia synthesis: the PH Emmett award address, Catal. Rev.: Sci. Eng., 1980, 21, 201–223 CrossRef CAS.
- G. Ertl, Elementary steps in heterogeneous catalysis, Angew. Chem., Int. Ed., 1990, 29, 1219–1227 CrossRef.
- D. R. Strongin, J. Carrazza, S. R. Bare and G. A. Somorjai, The importance of C7 sites and surface roughness in the ammonia synthesis reaction over iron, J. Catal., 1987, 103, 213–215 CrossRef CAS.
- K. Reuter, C. P. Plaisance, H. Oberhofer and M. Andersen, Perspective: on the active site model in computational catalyst screening, J. Chem. Phys., 2017, 146, 040901 CrossRef PubMed.
- K. Honkala, A. Hellman, I. Remediakis, A. Logadottir, A. Carlsson, S. Dahl, C. H. Christensen and J. K. Nørskov, Ammonia synthesis from first-principles calculations, Science, 2005, 307, 555–558 CrossRef CAS PubMed.
- N. Spencer, R. Schoonmaker and G. A. Somorjai, Iron single crystals as ammonia synthesis catalysts: effect of surface structure on catalyst activity, J. Catal., 1982, 74, 129–135 CrossRef CAS.
- D. Strongin, S. Bare and G. A. Somorjai, The effects of aluminum oxide in restructuring iron single crystal surfaces for ammonia synthesis, J. Catal., 1987, 103, 289–301 CrossRef CAS.
- D. Strongin and G. A. Somorjai, The effects of potassium on ammonia synthesis over iron single-crystal surfaces, J. Catal., 1988, 109, 51–60 CrossRef CAS.
- G. A. Somorjai and N. Materer, Surface structures in ammonia synthesis, Top. Catal., 1994, 1, 215–231 CrossRef CAS.
- J. J. Mortensen, L. B. Hansen, B. Hammer and J. K. Nørskov, Nitrogen adsorption and dissociation on Fe (111), J. Catal., 1999, 182, 479–488 CrossRef CAS.
- S. Dahl, A. Logadottir, R. Egeberg, J. Larsen, I. Chorkendorff, E. Törnqvist and J. K. Nørskov, Role of steps in N2 activation on Ru (0001), Phys. Rev. Lett., 1999, 83, 1814–1819 CrossRef.
- S. Dahl, E. Törnqvist and I. Chorkendorff, Dissociative adsorption of N2 on Ru (0001): a surface reaction totally dominated by steps, J. Catal., 2000, 192, 381–390 CrossRef CAS.
- S. Dahl, J. Sehested, C. Jacobsen, E. Törnqvist and I. Chorkendorff, Surface science based microkinetic analysis of ammonia synthesis over ruthenium catalysts, J. Catal., 2000, 192, 391–399 CrossRef CAS.
- J. M. G. Carballo, J. Yang, A. Holmen, S. García-Rodríguez, S. Rojas, M. Ojeda and J. L. G. Fierro, Catalytic effects of ruthenium particle size on the Fischer–Tropsch synthesis, J. Catal., 2011, 284, 102–108 CrossRef CAS.
- H. Fang, D. Liu, Y. Luo, Y. Zhou, S. Liang, X. Wang, B. Lin and L. Jiang, Challenges and opportunities of Ru-based catalysts toward the synthesis and utilization of ammonia, ACS Catal., 2022, 12, 3938–3954 CrossRef CAS.
- P. Wang, F. Chang, W. Gao, J. Guo, G. Wu, T. He and P. Chen, Breaking scaling relations to achieve low-temperature ammonia synthesis through LiH-mediated nitrogen transfer and hydrogenation, Nat. Chem., 2017, 9, 64–70 CrossRef CAS PubMed.
- Y. Gong, J. Wu, M. Kitano, J. Wang, T.-N. Ye, J. Li, Y. Kobayashi, K. Kishida, H. Abe, Y. Niwa, H. Yang, T. Tada and H. Hosono, Ternary intermetallic LaCoSi as a catalyst for N2 activation, Nat. Catal., 2018, 1, 178–185 CrossRef CAS.
- Y. Kobayashi, Y. Tang, T. Kageyama, H. Yamashita, N. Masuda, S. Hosokawa and H. Kageyama, Titanium-based hydrides as heterogeneous catalysts for ammonia synthesis, J. Am. Chem. Soc., 2017, 139, 18240–18246 CrossRef CAS PubMed.
- J.-C. Liu, X.-L. Ma, Y. Li, Y.-G. Wang, H. Xiao and J. Li, Heterogeneous Fe3 single-cluster catalyst for ammonia synthesis via an associative mechanism, Nat. Commun., 2018, 9, 1610–1619 CrossRef PubMed.
- X. Wang, X. Peng, W. Chen, G. Liu, A. Zheng, L. Zheng, J. Ni, C.-t. Au and L. Jiang, Insight into dynamic and steady-state active sites for nitrogen activation to ammonia by cobalt-based catalyst, Nat. Commun., 2020, 11, 653–663 CrossRef CAS PubMed.
- L. Li, Y.-F. Jiang, T. Zhang, H. Cai, Y. Zhou, B. Lin, X. Lin, Y. Zheng, L. Zheng and X. Wang, Size sensitivity of supported Ru catalysts for ammonia synthesis: from nanoparticles to subnanometric clusters and atomic clusters, Chem, 2022, 8, 749–768 CAS.
- J. Li, Y. Li and T. Zhang, Recent progresses in the research of single-atom catalysts, Sci. China Mater., 2020, 63, 889–891 CrossRef.
- E. C. Tyo and S. Vajda, Catalysis by clusters with precise numbers of atoms, Nat. Nanotechnol., 2015, 10, 577–588 CrossRef CAS PubMed.
- Q. Yang, Y. Jiang, H. Zhuo, E. M. Mitchell and Q. Yu, Recent progress of metal single-atom catalysts for energy applications, Nano Energy, 2023, 108404 CrossRef CAS.
- B. Qiao, A. Wang, X. Yang, L. F. Allard, Z. Jiang, Y. Cui, J. Liu, J. Li and T. Zhang, Single-atom catalysis of CO oxidation using Pt1/FeOx, Nat. Chem., 2011, 3, 634–641 CrossRef CAS PubMed.
- Y. Zhou, F. Wei, H. Qi, Y. Chai, L. Cao, J. Lin, Q. Wan, X. Liu, Y. Xing and S. Lin, Peripheral-nitrogen effects on the Ru1 centre for highly efficient propane dehydrogenation, Nat. Catal., 2022, 5, 1145–1156 CrossRef CAS.
- Q. He, Y. Meng, H. Zhang, Y. Zhang, Q. Sun, T. Gan, H. Xiao, X. He and H. Ji, Amino-metalloporphyrin polymers derived Fe single atom catalysts for highly efficient oxygen reduction reaction, Sci. China: Chem., 2020, 63, 810–817 CrossRef CAS.
- F. Chen, X. Jiang, L. Zhang, R. Lang and B. Qiao, Single-atom catalysis: bridging the homo-and heterogeneous catalysis, Chin. J. Catal., 2018, 39, 893–898 CrossRef CAS.
- Y. Wang, L. Chen, Z. Mao, L. Peng, R. Xiang, X. Tang, J. Deng, Z. Wei and Q. Liao, Controlled synthesis of single cobalt atom catalysts via a facile one-pot pyrolysis for efficient oxygen reduction and hydrogen evolution reactions, Sci. Bull., 2019, 64, 1095–1102 CrossRef CAS PubMed.
- J. Liu, H. Lu, D. W. Zhang and M. Nolan, Reactions of ruthenium cyclopentadienyl precursor in the metal precursor pulse of Ru atomic layer deposition, J. Mater. Chem. C, 2021, 9, 2919–2932 RSC.
- A. Wang, J. Li and T. Zhang, Heterogeneous single-atom catalysis, Nat. Rev. Chem, 2018, 2, 65–81 CrossRef CAS.
- F. Huang, Y. Deng, Y. Chen, X. Cai, M. Peng, Z. Jia, P. Ren, D. Xiao, X. Wen and N. Wang, Atomically dispersed Pd on nanodiamond/graphene hybrid for selective hydrogenation of acetylene, J. Am. Chem. Soc., 2018, 140, 13142–13146 CrossRef CAS PubMed.
- X. Cui, X. Dai, A.-E. Surkus, K. Junge, C. Kreyenschulte, G. Agostini, N. Rockstroh and M. Beller, Zinc single atoms on N-doped carbon: an efficient and stable catalyst for CO2 fixation and conversion, Chin. J. Catal., 2019, 40, 1679–1685 CrossRef.
- J. M. Thomas, Tens of thousands of atoms replaced by one, Nature, 2015, 525, 325–326 CrossRef CAS PubMed.
- J.-Z. Qiu, J. Hu, J. Lan, L.-F. Wang, G. Fu, R. Xiao, B. Ge and J. Jiang, Pure siliceous zeolite-supported Ru single-atom active sites for ammonia synthesis, Chem. Mater., 2019, 31, 9413–9421 CrossRef CAS.
- Á. Logadóttir and J. K. Nørskov, Ammonia synthesis over a Ru (0001) surface studied by density functional calculations, J. Catal., 2003, 220, 273–279 CrossRef.
- X. Wang, L. Li, Z. Fang, Y. Zhang, J. Ni, B. Lin, L. Zheng, C.-t. Au and L. Jiang, Atomically dispersed Ru catalyst for low-temperature nitrogen activation to ammonia via an associative mechanism, ACS Catal., 2020, 10, 9504–9514 CrossRef CAS.
- F. Rosowski, A. Hornung, O. Hinrichsen, D. Herein, M. Muhler and G. Ertl, Ruthenium catalysts for ammonia synthesis at high pressures: preparation, characterization, and power-law kinetics, Appl. Catal.,
A, 1997, 151, 443–460 CrossRef CAS.
- X. Wang, X. Peng, H. Ran, B. Lin, J. Ni, J. Lin and L. Jiang, Influence of Ru substitution on the properties of LaCoO3 catalysts for ammonia synthesis: XAFS and XPS studies, Ind. Eng. Chem. Res., 2018, 57, 17375–17383 CrossRef CAS.
- A. Han, B. Wang, A. Kumar, Y. Qin, J. Jin, X. Wang, C. Yang, B. Dong, Y. Jia and J. Liu, Recent advances for MOF-derived carbon-supported single-atom catalysts, Small Methods, 2019, 3, 1800471–1800492 CrossRef.
- Y. Zhou, C. Wang, X. Peng, T. Zhang, X. Wang, Y. Jiang, H. Qi, L. Zheng, J. Lin and L. Jiang, Boosting efficient ammonia synthesis over atomically dispersed Co-based catalyst via the modulation of geometric and electronic structures, CCS Chem., 2022, 4, 1758–1769 CrossRef CAS.
- X. Li, Y. Jiao, Y. Cui, C. Dai, P. Ren, C. Song and X. Ma, Synergistic catalysis of the synthesis of ammonia with Co-based catalysts and plasma: from nanoparticles to a single atom, ACS Appl. Mater. Interfaces, 2021, 13, 52498–52507 CrossRef CAS PubMed.
- Z. Chen, Y. Ye, T. Peng, C. Wu, H. Li, X. Pan and X. Bao, Iron-single sites confined by graphene lattice for ammonia synthesis under mild conditions, ACS Catal., 2023, 13, 14385–14394 CrossRef CAS.
- L. M. Azofra, N. Morlanés, A. Poater, M. K. Samantaray, B. Vidjayacoumar, K. Albahily, L. Cavallo and J. M. Basset, Single-site molybdenum on solid support materials for catalytic hydrogenation of N2-into-NH3, Angew. Chem., Int. Ed., 2018, 57, 15812–15816 CrossRef CAS PubMed.
- B. Lin, Y. Wu, B. Fang, C. Li, J. Ni, X. Wang, J. Lin and L. Jiang, Ru surface density effect on ammonia synthesis activity and hydrogen poisoning of ceria-supported Ru catalysts, Chin. J. Catal., 2021, 42, 1712–1723 CrossRef CAS.
- C. J. H. Jacobsen, S. Dahl, P. L. Hansen, E. Törnqvist, L. Jensen, H. Topsøe, D. V. Prip, P. B. Møenshaug and I. Chorkendorff, Structure sensitivity of supported ruthenium catalysts for ammonia synthesis, J. Mol. Catal. A: Chem., 2000, 163, 19–26 CrossRef CAS.
- Y. Zhou, J. Wang, L. Liang, Q. Sai, J. Ni, C.-t. Au, X. Lin, X. Wang, Y. Zheng, L. Zheng and L. Jiang, Unraveling the size-dependent effect of Ru-based catalysts on ammonia synthesis at mild conditions, J. Catal., 2021, 404, 501–511 CrossRef CAS.
- J. Li, W. Wang, W. Chen, Q. Gong, J. Luo, R. Lin, H. Xin, H. Zhang, D. Wang, Q. Peng, W. Zhu, C. Chen and Y. Li, Sub-nm ruthenium cluster as an efficient and robust catalyst for decomposition and synthesis of ammonia: break the “size shackles”, Nano Res., 2018, 11, 4774–4785 CrossRef CAS.
- L. D. Marks, Experimental studies of small particle structures, Rep. Prog. Phys., 1994, 57, 603–649 CrossRef CAS.
- F. Tao, S. Dag, L.-W. Wang, Z. Liu, D. R. Butcher, H. Bluhm, M. Salmeron and G. A. Somorjai, Break-up of stepped platinum catalyst surfaces by high CO coverage, Science, 2010, 327, 850–853 CrossRef CAS PubMed.
- J. Feng, L. Liu, X. Ju, M. Wang, X. Zhang, J. Wang and P. Chen, Sub-nanometer Ru clusters on ceria nanorods as efficient catalysts for ammonia synthesis under mild conditions, ACS Sustain. Chem. Eng., 2022, 10, 10181–10191 CrossRef CAS.
- S. Hirabayashi, M. Ichihashi and Y. Takeda, Optimization of ruthenium particle size and ceria support for enhanced activity of Ru/CeO2 cluster catalysts in ammonia synthesis under mild conditions, Catal. Lett., 2023, 154, 487–493 CrossRef.
- J. Wang, L. Liu, X. Zhang, J. Yu, X. Ju, J. Feng, J. Guo, T. He and P. Chen, Sub-nanometer Ru clusters on Sm2O3 obtained from a room temperature ion adsorption method for ammonia synthesis, Catal. Sci. Technol., 2022, 12, 7501–7509 RSC.
- X. Zhang, L. Liu, A. Wu, J. Zhu, R. Si, J. Guo, R. Chen, Q. Jiang, X. Ju and J. Feng, Synergizing surface hydride species and Ru clusters on Sm2O3 for efficient ammonia synthesis, ACS Catal., 2022, 12, 2178–2190 CrossRef CAS.
- Y. Zhang, S. Li, C. Sun, X. Cao, X. Wang and J. Yao, Defective g-C3N4 supported Ru3 single-cluster catalyst for ammonia synthesis through parallel reaction pathways, Nano Res., 2023, 16, 3580–3587 CrossRef CAS.
- Q.-Y. Fan, J.-L. Liu, F.-Q. Gong, Y. Wang and J. Cheng, Structural dynamics of Ru clusters during nitrogen dissociation in ammonia synthesis, Phys. Chem. Chem. Phys., 2022, 24, 10820–10825 RSC.
- Y. Zhou, Q. Sai, Z. Tan, C. Wang, X. Wang, B. Lin, J. Ni, J. Lin and L. Jiang, Highly efficient subnanometer Ru-based catalyst for ammonia synthesis via an associative mechanism, Chin. J. Chem. Eng., 2022, 43, 177–184 CrossRef CAS.
- S. E. Sivan, K. H. Kang, S. J. Han, O. Francis Ngome Okello, S.-Y. Choi, V. Sudheeshkumar, R. W. J. Scott, H.-J. Chae, S. Park and U. H. Lee, Facile MOF-derived one-pot synthetic approach toward Ru single atoms, nanoclusters, and nanoparticles dispersed on CeO2 supports for enhanced ammonia synthesis, J. Catal., 2022, 408, 316–328 CrossRef CAS.
- T. Bligaard, J. K. Nørskov, S. Dahl, J. Matthiesen, C. H. Christensen and J. Sehested, The Brønsted–Evans–Polanyi relation and the volcano curve in heterogeneous catalysis, J. Catal., 2004, 224, 206–217 CrossRef CAS.
- S. Dahl, A. Logadottir, C. J. H. Jacobsen and J. K. Nørskov, Electronic factors in catalysis: the volcano curve and the effect of promotion in catalytic ammonia synthesis, Appl. Catal., A, 2001, 222, 19–29 CrossRef CAS.
- A. J. Medford, A. Vojvodic, J. S. Hummelshøj, J. Voss, F. Abild-Pedersen, F. Studt, T. Bligaard, A. Nilsson and J. K. Nørskov, From the Sabatier principle to a predictive theory of transition-metal heterogeneous catalysis, J. Catal., 2015, 328, 36–42 CrossRef CAS.
- C. Cui, H. Zhang, R. Cheng, B. Huang and Z. Luo, On the nature of three-atom metal cluster catalysis for N2 reduction to ammonia, ACS Catal., 2022, 12, 14964–14975 CrossRef CAS.
- L. Liu, M. Lopez-Haro, C. W. Lopes, S. Rojas-Buzo, P. Concepcion, R. Manzorro, L. Simonelli, A. Sattler, P. Serna, J. J. Calvino and A. Corma, Structural modulation and direct measurement of subnanometric bimetallic PtSn clusters confined in zeolites, Nat. Catal., 2020, 3, 628–638 CrossRef CAS.
- X. Li, Y. Zhou, B. Qiao, X. Pan, C. Wang, L. Cao, L. Li, J. Lin and X. Wang, Enhanced stability of Pt/Al2O3 modified by Zn promoter for catalytic dehydrogenation of ethane, J. Energy Chem., 2020, 51, 14–20 CrossRef.
- T. N. Ye, S. W. Park, Y. Lu, J. Li, M. Sasase, M. Kitano, T. Tada and H. Hosono, Vacancy-enabled N2 activation for ammonia synthesis on an Ni-loaded catalyst, Nature, 2020, 583, 391–395 CrossRef CAS PubMed.
- T. N. Ye, S. W. Park, Y. Lu, J. Li, J. Wu, M. Sasase, M. Kitano and H. Hosono, Dissociative and associative concerted mechanism for ammonia synthesis over Co-based catalyst, J. Am. Chem. Soc., 2021, 143, 12857–12866 CrossRef CAS PubMed.
- L. Nguyen, S. Zhang, L. Wang, Y. Li, H. Yoshida, A. Patlolla, S. Takeda, A. I. Frenkel and F. Tao, Reduction of nitric oxide with hydrogen on catalysts of singly dispersed bimetallic sites Pt1Com and Pd1Con, ACS Catal., 2016, 6, 840–850 CrossRef CAS.
- J. Ni, Z. Tan, Q. Sai, J. Zhu, X. Wang, B. Lin, J. Lin, C.-t. Au and L. Jiang, Target-oriented confinement of Ru-Co nanoparticles inside N-doped carbon spheres via a benzoic acid guided process for high-efficient low-temperature ammonia synthesis, J. Energy Chem., 2021, 57, 140–146 CrossRef CAS.
- J. Yang, D. He, W. Chen, W. Zhu, H. Zhang, S. Ren, X. Wang, Q. Yang, Y. Wu and Y. Li, Bimetallic Ru–Co clusters derived from a confined alloying process within zeolite–imidazolate frameworks for efficient NH3 decomposition and synthesis, ACS Appl. Mater. Interfaces, 2017, 9, 39450–39455 CrossRef CAS PubMed.
- S. Zhang, L. Nguyen, J. X. Liang, J. Shan, J. J. Liu, A. I. Frenkel, A. Patlolla, W. Huang, J. Li and F. F. Tao, Catalysis on singly dispersed bimetallic sites, Nat. Commun., 2015, 6, 7938 CrossRef CAS PubMed.
- X.-L. Ma, Y. Yang, L.-M. Xu, H. Xiao, W.-Z. Yao and J. Li, Theoretical investigation on hydrogenation of dinitrogen triggered by singly dispersed bimetallic sites, J. Mater. Chem. A, 2022, 10, 6146–6152 RSC.
- X. L. Ma, J. C. Liu, H. Xiao and J. Li, Surface single-cluster catalyst for N2-to-NH3 thermal conversion, J. Am. Chem. Soc., 2018, 140, 46–49 CrossRef CAS PubMed.
- T. Nakao, T. Tada and H. Hosono, Transition metal-doped Ru nanoparticles loaded on metal hydrides for efficient ammonia synthesis from first principles, J. Phys. Chem. C, 2020, 124, 1529–1534 CrossRef CAS.
- R. Cheng, C. Cui and Z. Luo, Catalysis of dinitrogen activation and reduction by a single Fe13 cluster and its doped systems, Phys. Chem. Chem. Phys., 2023, 25, 1196–1204 RSC.
- R. T. Hannagan, G. Giannakakis, M. Flytzani-Stephanopoulos and E. C. H. Sykes, Single-atom alloy catalysis, Chem. Rev., 2020, 120, 12044–12088 CrossRef CAS PubMed.
- T. J. Zhang, A. G. Walsh, J. H. Yu and P. Zhang, Single-atom alloy catalysts: structural analysis, electronic properties and catalytic activities, Chem. Soc. Rev., 2021, 50, 569–588 RSC.
- Y. Zhang, J. Li, J. Cai, L. Yang, T. Zhang, J. Lin, X. Wang, C. Chen, L. Zheng, C.-t. Au, B. Yang and L. Jiang, Construction of spatial effect from atomically dispersed Co anchoring on subnanometer Ru cluster for enhanced N2-to-NH3 conversion, ACS Catal., 2021, 11, 4430–4440 CrossRef CAS.
- Y. Zhang, X. Peng, J. Deng, F. Sun, J. Cai, Y. Zhou, J. Ni, B. Lin, L. Zheng, X. Wang, J. Lin and L. Jiang, Tuning N2 activation pathway over Ru/Co sub-nanometer alloy for efficient ammonia synthesis, J. Catal., 2021, 404, 440–450 CrossRef CAS.
- A. R. Cholach and A. A. Bryliakova, Re-Co alloys and single-atom Re catalysts in ammonia synthesis: a DFT study, Mol. Catal., 2021, 513, 111801 CrossRef CAS.
- Y. Zhang, S. Li, C. Sun, P. Wang, Y. Yang, D. Yi, X. Wang and J. Yao, Understanding and modifying the scaling relations for ammonia synthesis on dilute metal alloys: from single-atom alloys to dimer alloys, ACS Catal., 2022, 12, 9201–9212 CrossRef CAS.
- A. R. Singh, J. H. Montoya, B. A. Rohr, C. Tsai, A. Vojyodic and J. K. Norskov, Computational design of active site structures with improved transition-state scaling for ammonia synthesis, ACS Catal., 2018, 8, 4017–4024 CrossRef CAS.
- M. Kitano, Y. Inoue, H. Ishikawa, K. Yamagata, T. Nakao, T. Tada, S. Matsuishi, T. Yokoyama, M. Hara and H. Hosono, Essential role of hydride ion in ruthenium-based ammonia synthesis catalysts, Chem. Sci., 2016, 7, 4036–4043 RSC.
- Y. Zhou, C. Wang, X. Peng, T. Zhang, X. Wang, Y. Jiang, H. Qi, L. Zheng, J. Lin and L. Jiang, Boosting efficient ammonia synthesis over atomically dispersed Co-based catalyst via the modulation of geometric and electronic structures, CCS Chem., 2022, 4, 1758–1769 CrossRef CAS.
- S. Wu, Y.-K. Peng, T.-Y. Chen, J. Mo, A. Large, I. McPherson, H.-L. Chou, I. Wilkinson, F. Venturini, D. Grinter, P. Ferrer Escorihuela, G. Held and S. C. E. Tsang, Removal of hydrogen poisoning by electrostatically polar MgO support for low-pressure NH3 synthesis at a high rate over the Ru catalyst, ACS Catal., 2020, 10, 5614–5622 CrossRef CAS.
- L. M. Azofra, N. Morlanes, A. Poater, M. K. Samantaray, B. Vidjayacoumar, K. Albahily, L. Cavallo and J. M. Basset, Single-site molybdenum on solid support materials for catalytic hydrogenation of N2-into-NH3, Angew. Chem., Int. Ed., 2018, 57, 15812–15816 CrossRef CAS PubMed.
- J. Z. Qiu, J. B. Hu, J. G. Lan, L. F. Wang, G. Y. Fu, R. J. Xiao, B. H. Ge and J. X. Jiang, Pure siliceous zeolite-supported Ru single-atom active sites for ammonia synthesis, Chem. Mater., 2019, 31, 9413–9421 CrossRef CAS.
- H. Duan, J.-C. Liu, M. Xu, Y. Zhao, X.-L. Ma, J. Dong, X. Zheng, J. Zheng, C. S. Allen, M. Danaie, Y.-K. Peng, T. Issariyakul, D. Chen, A. I. Kirkland, J.-C. Buffet, J. Li, S. C. E. Tsang and D. O'Hare, Molecular nitrogen promotes catalytic hydrodeoxygenation, Nat. Catal., 2019, 2, 1078–1087 CrossRef CAS.
- Y. Zhou, C.-Q. Xu, Z. Tan, H. Cai, X. Wang, J. Li, L. Zheng, C.-t. Au, J. Li and L. Jiang, Integrating dissociative and associative routes for efficient ammonia synthesis over a TiCN-promoted Ru-based catalyst, ACS Catal., 2022, 12, 2651–2660 CrossRef CAS.
- L. H. Mou, T. T. Han, P. E. S. Smith, E. Sharman and J. Jiang, Machine learning descriptors for data-driven catalysis study, Adv. Sci., 2023, 10, 2301020 CrossRef CAS PubMed.
- T. Toyao, Z. Maeno, S. Takakusagi, T. Kamachi, I. Takigawa and K. Shimizu, Machine learning for catalysis informatics: recent applications and prospects, ACS Catal., 2020, 10, 2260–2297 CrossRef CAS.
- X.-C. Hu, X.-P. Fu, W.-W. Wang, X. Wang, K. Wu, R. Si, C. Ma, C.-J. Jia and C.-H. Yan, Ceria-supported ruthenium clusters transforming from isolated single atoms for hydrogen production via decomposition of ammonia, Appl. Catal., B, 2020, 268, 118424–118437 CrossRef CAS.
Footnote |
† These authors contributed equally to this work. |
|
This journal is © The Royal Society of Chemistry 2024 |